A non canonical subtilase attenuates the transcriptional activation of defence responses in Arabidopsis thaliana
Abstract
Proteases play crucial physiological functions in all organisms by controlling the lifetime of proteins. Here, we identified an atypical protease of the subtilase family [SBT5.2(b)] that attenuates the transcriptional activation of plant defence independently of its protease activity. The SBT5.2 gene produces two distinct transcripts encoding a canonical secreted subtilase [SBT5.2(a)] and an intracellular protein [SBT5.2(b)]. Concomitant to SBT5.2(a) downregulation, SBT5.2(b) expression is induced after bacterial inoculation. SBT5.2(b) localizes to endosomes where it interacts with and retains the defence-related transcription factor MYB30. Nuclear exclusion of MYB30 results in its reduced transcriptional activation and, thus, suppressed resistance. sbt5.2 mutants, with abolished SBT5.2(a) and SBT5.2(b) expression, display enhanced defence that is suppressed in a myb30 mutant background. Moreover, overexpression of SBT5.2(b), but not SBT5.2(a), in sbt5.2 plants reverts the phenotypes displayed by sbt5.2 mutants. Overall, we uncover a regulatory mode of the transcriptional activation of defence responses previously undescribed in eukaryotes.
https://doi.org/10.7554/eLife.19755.001eLife digest
Like animals, plants have evolved numerous ways to protect themselves from disease. When a plant detects an invading microbe, it massively changes which genes it expresses to establish a defensive response. This is possible thanks to the action of a type of protein, named transcription factors, which are able to bind to DNA in the cell nucleus and regulate gene expression. However, triggering such a response comes at a cost, and so plants must keep their defensive response in check such that they can allocate resources in a balanced way.
In the model plant Arabidopsis, a protein named MYB30 is one transcription factor that is able to promote disease resistance. Previous research identified some proteins that can reduce the activity of this transcription factor to avoid triggering a response when it is not needed, for example, when no infectious microbes are present. However, it was likely that other proteins were also involved in the process.
Now, Serrano et al. report that an enzyme called SBT5.2 is an additional negative regulator of MYB30 activity. SBT5.2 belongs to a family of protein-degrading enzymes called subtilases, which are typically localized outside cells. As such, it was unclear how SBT5.2 could interact and regulate a transcription factor that is found inside the nucleus of plant cells.
Nevertheless, Serrano et al. found that the gene that encodes SBT5.2 actually gives rise to two distinct proteins. The first is a classical subtilase that is indeed located outside of the cell, and so cannot interact with MYB30 and does not affect its activity. The second protein is an atypical subtilase that localises to bubble-like compartments called vesicles within the cell and is able to highjack MYB30 on its way to the nucleus. When the atypical subtilase interacts with MYB30 at vesicles, it stops MYB30 from entering the nucleus. As a result, MYB30 cannot bind to the DNA nor activate its target genes. This means that the defensive response that normally depends on MYB30 is weakened.
The work of Serrano et al. uncovers a new way to regulate the expression of defence-related genes. Further unravelling the molecular mechanisms involved in the fine-tuning of gene expression represents a challenging task for future research.
https://doi.org/10.7554/eLife.19755.002Introduction
Regulation of protein turnover plays a central role in the proper functioning of eukaryotic cells. Indeed, proteases of different families have been shown to be involved in the control of metabolism, physiology, growth and adaptive responses to biotic and abiotic stimuli (van der Hoorn, 2008). Subtilisin-like proteases (subtilases) are serine proteases featuring a catalytic triad characterized by the three amino acids aspartate, histidine and serine (Dodson and Wlodawer, 1998). According to the MEROPS (http://merops.sanger.ac.uk) classification, subtilases belong to the S8 family within the SB clan of serine proteases and are grouped into two subfamilies, subtilisins (S8A) and kexins (S8B). Nine subtilases have been characterized in mammals, seven of which belong to the kexin group and two others to the S8A subfamily (pyrolysins). With no representatives of the kexin type, plant subtilases exclusively belong to the pyrolysin group within the S8A subfamily. Pyrolysin-related subtilase families are largely expanded throughout the plant kingdom with a degree of complexity that exceeds that of their mammalian counterparts (Schaller et al., 2012). In Arabidopsis the subtilase family comprises 56 members distributed in six distinct subgroups (SBT1-6) (Rautengarten et al., 2005). Despite their prevalence, our knowledge of the function of plant subtilases is rather poor. Subtilases are predicted to be secreted and have been involved in general protein turnover as well as in the highly specific regulation of plant development or responses to environmental changes and, more recently, in suppression of basal immunity and immune priming (Schaller et al., 2012; Figueiredo et al., 2014).
As sessile organisms, plants must face the diversity of pathogens that they encounter in their habitat. Plants, unlike mammals, lack mobile defender cells and a somatic adaptive immune system. Instead, they rely on the innate immunity of each cell and on systemic signals originating from infection sites. Plant resistance to disease is a costly response, closely connected to plant physiological and developmental processes, and often associated with the so-called hypersensitive response (HR), a form of programmed cell death that develops at attempted infection sites, allegedly to prevent pathogen propagation through the plant (Coll et al., 2011). The sharp limit of the HR suggests the existence of tight regulatory mechanisms to restrict cell death development to the inoculated zone although the molecular actors involved in this process remain unknown for the most part. In line with the high cellular cost of triggering defence and cell death-associated responses, negative regulatory mechanisms are used by the plant to attenuate the activation of immune-related functions and allow a balanced allocation of resources upon pathogen challenge.
Transcriptional reprogramming of the plant cell is a crucial step that allows mounting of efficient defence responses after pathogen attack. Transcription factors (TFs) and co-regulatory proteins play essential roles in launching and regulating the transcriptional changes that direct the plant defence response (Buscaill and Rivas, 2014; Tsuda and Somssich, 2015). MYB TFs of the R2R3 type (126 members in Arabidopsis) mostly regulate plant-specific functions (Dubos et al., 2010). Among the MYB TFs regulating defence-related transcription, Arabidopsis MYB30 is one of the best characterized. MYB30 promotes defence and cell death-associated responses through the transcriptional activation of genes related to the lipid biosynthesis pathway that leads to the production of very-long-chain fatty acids (VLCFAs) (Raffaele et al., 2008). MYB30 is targeted by the effector protein XopD, from the bacterial pathogen Xanthomonas campestris pv. campestris (Xcc), leading to suppression of MYB30-mediated transcriptional activation of plant resistance and thus underlining the important role played by MYB30 in plant defence regulation (Canonne et al., 2011).
A previously performed yeast two-hybrid (Y2H) screen using MYB30 as bait identified a secretory phospholipase (AtsPLA2-α) and a RING-type E3 ubiquitin ligase (MIEL1) that exert negative spatial and temporal control on MYB30 transcriptional activity through distinct molecular mechanisms (Froidure et al., 2010; Marino et al., 2013). Here, we describe SBT5.2, a serine protease of the subtilisin group, as a new MYB30-interacting partner. We demonstrate that the SBT5.2 transcript is alternatively spliced and that one of the two SBT5.2 splice variants, SBT5.2(b), whose expression pattern follows that of MYB30 after bacterial treatment, encodes an atypical subtilase that specifically mediates retention of MYB30 at endosomal vesicles. This phenomenon is independent of the integrity of the SBT5.2(b) catalytic triad, requires N-terminal myristoylation of SBT5.2(b) and results in attenuation of MYB30-mediated HR. Our work uncovers a novel regulatory mode for a subtilase protein and underlines the intricacy of the transcriptional regulation of plant responses to pathogen attack.
Results
Identification of SBT5.2
In order to search for components involved in MYB30-mediated signalling, a Y2H screen was previously conducted using a MYB30 version deleted from its transcriptional activation domain (MYB30ΔAD) (Froidure et al., 2010) as bait. A cDNA clone encoding the last 103 amino acids of the Arabidopsis serine protease of the subtilisin group SBT5.2 (At1g20160) was identified in this screen (Figure 1). SBT5.2 belongs to subgroup V (6 members) within the classification of the Arabidopsis subtilase family (Schaller et al., 2012; Rautengarten et al., 2005).
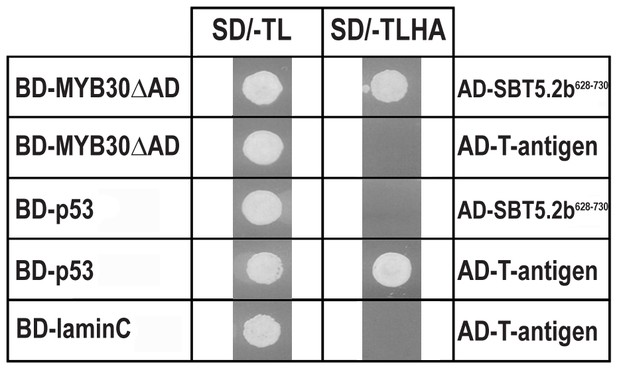
Specific interaction between MYB30 and SBT5.2 in yeast.
Yeasts are shown after growth for five days on low stringency (left; SD/-TL) or high stringency (right; SD/-TLHA) media. Co-expression of MYB30 deleted from its C-terminal transcription activation domain (MYB30△AD) and the isolated cDNA clone encoding the last 103 amino acids of SBT5.2 (SBT5.2628-730) resulted in yeast growth on selective medium. In a control experiment, yeast cells expressing MYB30△AD or SBT5.2628-730 with controls provided by Clontech (T-antigen or P53, respectively) were not able to grow on selective medium. BD, GAL4 DNA-binding domain; AD, GAL4 activation domain.
Two gene models are annotated in the TAIR database (http://www.arabidopsis.org) for At1g20160, suggesting that the corresponding transcript is alternatively spliced (Figure 2a). Comparison of the two SBT5.2 cDNA clones [designated SBT5.2(a) and SBT5.2(b)] with the genomic sequence revealed that the first intron is specifically spliced in the SBT5.2(b) cDNA (Figure 2—figure supplement 1a). Reverse transcription PCR (RT-PCR) using specific cDNA primers confirmed the existence of both splice variants in planta (Figure 2b). The sequence of SBT5.2(a) and SBT5.2(b) 5’ ends was determined by 5’ RACE and cDNA sequencing (Figure 2—figure supplement 1b). To gain knowledge on the relative abundance of both isoforms after bacterial inoculation, we monitored the expression of SBT5.2(a) and SBT5.2(b) in Col-0 wild-type plants inoculated with Pseudomonas syringae pv. tomato DC3000 expressing the avirulence gene AvrRpm1 (Pst AvrRpm1). As shown in Figure 2c, expression of SBT5.2(a) was downregulated after treatment with bacteria, whereas expression of SBT5.2(b) was induced and displayed an expression profile highly similar to that of MYB30 (Figure 2c). This data suggests that SBT5.2(b) may have a MYB30-related function.
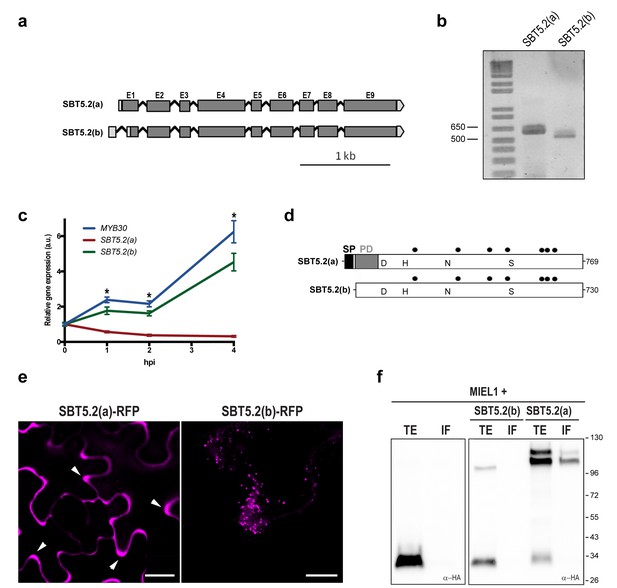
SBT5.2 is alternatively spliced.
(a) Genomic structure of SBT5.2 alternatively spliced variants. Exons are shown as dark gray boxes (E1-E9), introns as black lines between exons and 5’ and 3’ UTRs are shown in light gray. (b) RT-PCR analysis of SBT5.2(a) and SBT5.2(b) transcripts in four-week old Col-0 Arabidopsis leaves. (c) The relative expression of SBT5.2(a), SBT5.2(b) and MYB30 at the indicated timepoints after inoculation of Col-0 plants with Pst AvrRpm1 (5 × 107 cfu/ml). Expression values were normalized using SAND family gene as internal standard and related to the value of each gene at time 0, which is set at 1. The SEM values were calculated from 4 independent experiments (4 replicates/experiment). The asterisks indicate statistically significant values for the three tested genes according to a Student’s t-test (p<0.005) and with respect to gene expression values at time 0. (d) Schematic representation of SBT5.2(a) and SBT5.2(b) protein sequences. The signal peptide (SP) and the pro-domain (PD) in the SBT5.2(a) isoform are shown as black and grey boxes, respectively. Catalytically conserved Asp, His, Asn, and Ser residues are indicated. Putative N-glycosylation sites (PGSs) are indicated by black dots. (e) Confocal images of epidermal N. benthamiana cells 36 hr after Agrobacterium-mediated transient expression of the indicated constructs. Accumulation of SBT5.2(a)-RFP in apoplastic spaces is indicated by arrowheads. Bars = 10 µm. (f) Western blot analysis of total protein extracts (TE) and intercellular fluids (IF) from N. benthamiana leaves expressing the intracellular protein MIEL1 alone (left) or co-expressed with HA-tagged SBT proteins (right), as indicated. Molecular mass markers in kilodaltons are indicated on the right.
SBT5.2(a) corresponds to a transcript of 2402 bp, which is predicted to encode a 769 amino acid preproenzyme containing a signal peptide (SP) followed a by prodomain (PD), which acts as an intramolecular inhibitor, and a mature polypeptide (Figure 2d; Figure 2—figure supplement 1c). In contrast, SBT5.2(b) corresponds to a transcript of 2373 bp, predicted to encode a protein of 730 amino acids with no SP and lacking the first five amino acids of the PD (Figure 2d; Figure 2—figure supplement 1c). Except for their N-terminal differences, the two corresponding encoded proteins are predicted to be identical and contain a catalytic triad with three amino acids (D145, H210 and S546 in SBT5.2(a), and D106, H171 and S507 in SBT5.2(b)) conserved within serine proteases (Figure 2d; Figure 2—figure supplement 1b).
SBT5.2(a) is a secreted protein whereas SBT5.2(b) is intracellular
Alternative splicing (AS) of SBT5.2 may have important implications for the subcellular localization and function of the proteins encoded by the two transcripts. The presence of a SP and a PD in SBT5.2(a) suggests that this protein may enter the secretory pathway and be secreted to the extracellular space. Indeed, secretion of SBT5.2(a) was previously reported (Engineer et al., 2014; Kaschani et al., 2012). In contrast, the absence of the SP in SBT5.2(b) may prevent secretion of the protein. In order to test this possibility, the subcellular localization of the two proteins was first investigated using Agrobacterium-mediated transient expression of RFP-tagged SBT5.2(a) and SBT5.2(b) under the control of a dexamethasone- (Dex-) inducible promoter in leaf epidermal cells of N. benthamiana. As expected, SBT5.2(a) was found to be located in apoplastic spaces whereas SBT5.2(b) was detected at vesicle-like structures inside cells (Figure 2e).
To obtain biochemical validation of the subcellular localization of SBT5.2(a) and SBT5.2(b), HA-tagged versions of both proteins were transiently expressed in N. benthamiana and intercellular fluid (IF) was isolated. In order to control the detection of intracellular proteins in the IF, the intracellular protein MIEL1 (Marino et al., 2013) was co-expressed with SBT5.2 proteins. As expected for an intracellular protein, MIEL1 was detected in the total extract fraction (TE) and not in the IF, confirming that the IF fraction did not contain intracellular proteins due to unintentional cellular lysis during IF isolation (Figure 2f). SBT5.2(a) was detected in the IF as two protein bands that may correspond to the processed and unprocessed forms of the protease, whereas SBT5.2(b) was exclusively detected in the TE and never in the IF fraction (Figure 2f). These results confirm the secretion of SBT5.2(a) and the intracellular localization of SBT5.2(b).
SBT5.2(a), but not SBT5.2(b), is N-glycosylated
Most extracellular or secreted proteins are modified via N-glycosylation (Moremen et al., 2012). Seven putative N-linked glycosylation sites (PGSs; N in NxS/T motifs) are present in SBT5.2 proteins [N225, N363, N467, N525, N636, N650 and N678 in SBT5.2(a)]. In order to test whether SBT5.2 proteins are glycosylated in planta, protein extracts containing SBT5.2(a) and SBT5.2(b) were treated with PNGase F or EndoH and analysed for mobility shifts by Western Blot. At the end of their maturation in the secretory pathway, some plant N-linked glycans are modified, which renders them resistant to cleavage by glycosylases (Lerouge et al., 1998). In agreement, an electrophoretic shift was only observed for the slow migrating, unprocessed form of SBT5.2(a), whereas migration of the fully processed form remained unaltered (Figure 3a). In addition, SBT5.2(a) bound to and was eluted from a concanavalin A resin (Figure 3b), further suggesting that SBT5.2(a) is a glycosylated protein. Finally, the increased electrophoretic mobility of SBT5.2(a) in protein extracts from N. benthamiana leaves expressing HA-tagged SBT5.2(a) and treated with tunicamycin, an inhibitor of N-linked glycosylation of newly synthesized glycoproteins in the ER (Bassik and Kampmann, 2011), further confirmed SBT5.2(a) N-glycosylation in planta (Figure 3c).
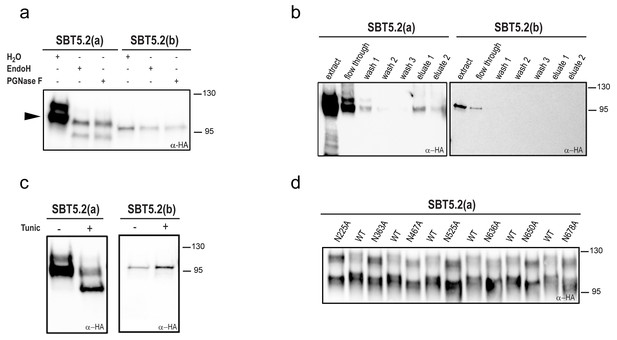
SBT5.2(a), but not SBT5.2(b), is glycosylated in planta.
(a) SBT5.2(a), but not SBT5.2(b), is deglycosylated by PNGase F and Endo H. Protein extracts containing HA-tagged SBT5.2(a) and SBT5.2(b) transiently expressed in N. benthamiana were treated (+) or not (−) with PNGase F or Endo H as indicated. The arrowhead on the left indicates the fully processed form of SBT5.2(a) whose mobility is not affected by the enzymatic treatment. (b) HA-tagged SBT5.2(a), but not SBT5.2(b) can be affinity purified using a concanavalin A resin. (c) Glycosylation of HA-tagged SBT5.2(a), but not SBT5.2(b), is blocked by tunicamycin treatment (+) after transient expression in N. benthamiana. (d) Electrophoretic mobility of individual HA-tagged PGS SBT5.2(a) mutants. Mutated N to A residues are indicated. WT: wild-type SBT5.2(a) proteins were interspersed to facilitate detection of the mobility shifts. In all cases, Western blot analyses were performed using anti-HA antibodies.
We next analyzed individual PGS removal mutants (in which the N residue was replaced by A) on high resolution SDS-PAGE gels to determine their electrophoretic mobility as compared to wild-type SBT5.2(a). This analysis revealed a small but significant mobility shift for all SBT5.2(a) PGS mutants (Figure 3d), suggesting that all PGS in SBT5.2(a) are used in planta.
Despite the fact that SBT5.2(b) contains the seven PGSs present in SBT5.2(a), (i) SBT5.2(b) electrophoretic mobility was not modified after treatment with PNGase F or EndoH (Figure 3a); (ii) neither SBT5.2(b) binding to nor elution from a concanavalin A resin was observed (Figure 3b); and (iii) no effect of tunicamycin leaf treatment on the SBT5.2(b) electrophoresis profile was detected (Figure 3c). These results, which are consistent with the absence of SP in SBT5.2(b) and our previous observation that SBT5.2(b) is not secreted, strongly suggest that SBT5.2(b) does not enter the secretory pathway and is therefore not N-glycosylated.
SBT5.2(a), but not SBT5.2(b), shows serine protease activity
Subtilases, as other proteases, are typically able to catalyze their self-processing to render a mature active polypeptide. The presence of the three conserved amino acids in the catalytic triad of SBT5.2 proteins is consistent with these proteins displaying protease activity. When transiently expressing SBT5.2(a) in leaf epidermal cells of N. benthamiana, two protein bands were detected that, as mentioned earlier, may correspond to the processed and unprocessed forms of the protease (Figure 4a). In contrast, only a single band was detected for SBT5.2(b), suggesting that this protein is either not processed or fully processed in planta (Figure 4a). In order to learn more about the proteolytic cleavage of SBT5.2 proteins, we engineered SBT5.2(a) and SBT5.2(b) mutant versions, in which the conserved histidine residue in the catalytic triad of both proteins was mutated to alanine, [SBT5.2(a)H210A and SBT5.2(b)H171A]. Following transient expression in N. benthamiana, mutation of the catalytic histidine residue did not affect migration of SBT5.2(b) as compared to the wild-type protein, suggesting that this protein does not self-process in planta (Figure 4a). In contrast, in the case of SBT5.2(a)H210A, only the slow migrating band, that very likely corresponds to the unprocessed form of the protein, was detected (Figure 4a). This observation suggests that SBT5.2(a) is able to auto-process in planta and is thus active as a protease.
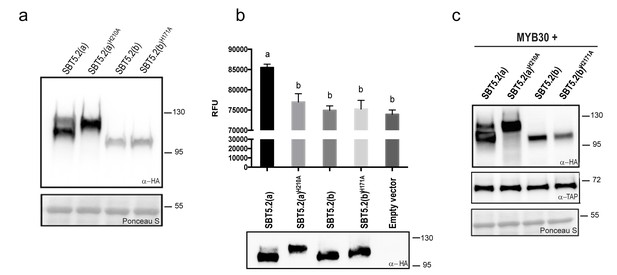
SBT5.2(a), but not SBT5.2(b), shows serine protease activity.
(a) Western blot analysis shows expression of HA-tagged SBT5.2(a), SBT5.2(b) and their catalytic mutant versions in N. benthamiana, as indicated. Ponceau S staining confirms equal loading. Molecular mass markers in kilodaltons are indicated on the right. (b) Fluorimetric assay to detect protease activity following incubation of Arabidopsis protoplasts expressing the indicated proteins with fluorescein isothiocyanate (FITC)-conjugated casein (top). RFU: relative fluorescence units. Error bars indicate SEM. Lowercase letters indicate significant differences as determined by Bonferroni-corrected p-values (p<0.001) obtained after ANOVA and subsequent LSD post-hoc test. All proteins were detected by Western blot (bottom). (c) TAP-tagged MYB30 was expressed in N. benthamiana alone or with SBT5.2(a), SBT5.2(b) and their catalytic mutant versions, as indicated. Western blot analysis shows the expression of TAP-tagged MYB30 and HA-tagged SBT proteins. Ponceau S staining confirms equal loading. Molecular mass markers in kilodaltons are indicated on the right.
The catalytic activity of HA-tagged SBT5.2(a) and SBT5.2(b) was further investigated using a fluorimetric assay. Protein extracts from Arabidopsis protoplasts expressing HA-tagged SBT5.2(a), SBT5.2(a)H210A, SBT5.2(b) or SBT5.2(b)H171A were incubated with the generic protease substrate casein conjugated to fluorescein isothiocyanate (FITC). Increased fluorescence was observed for SBT5.2(a), reflecting proteolytic cleavage of the FITC-casein substrate (Figure 4b). In contrast, fluorescence intensity in the case of SBT5.2(a)H210A, SBT5.2(b) and SBT5.2(b)H171A was indistinguishable from that of protoplasts transformed with an empty vector (Figure 4b), although their protein expression levels were comparable to those of SBT5.2(a) (Figure 4b). These results reinforce the idea that SBT5.2(a), but not SBT5.2(b), is correctly processed thus displaying protease activity.
To determine whether SBT5.2(a) or SBT5.2(b) are involved in MYB30 proteolytic processing, the in planta accumulation of MYB30 when expressed alone or together with the different SBT5.2 proteins was analysed. As shown in Figure 4c, MYB30 accumulation was consistently unaltered in the presence of SBT5.2(a) or SBT5.2(b) as compared to the expression observed in the presence of the respective subtilase catalytic mutant versions. These results suggest that neither SBT5.2(a) nor SBT5.2(b) are able to proteolytically cleave MYB30.
SBT5.2(b), but not SBT5.2(a), interacts with MYB30 at intracellular vesicles
MYB30 was previously localized to the nucleus of N. benthamiana and Arabidopsis cells (Froidure et al., 2010). In order to investigate MYB30 potential colocalization with SBT5.2(a) and/or SBT5.2(b), GFP-tagged MYB30 was co-expressed with RFP-tagged SBT5.2(a) or SBT5.2(b). Confocal microscopy analysis of N. benthamiana leaves transiently co-expressing GFP-MYB30 and SBT5.2(a)-RFP showed that these proteins do not co-localize in planta, as SBT5.2(a) and MYB30 retain their respective extracellular and nuclear localization when expressed together (Figure 5a). Surprisingly, when co-expressed with RFP-tagged SBT5.2(b), GFP-MYB30 was excluded from the nucleus and localized to the same vesicle-like structures where SBT5.2(b) was localized, suggesting a possible in planta interaction between the two proteins outside the nucleus (Figure 5a). Importantly, the unrelated MYB TF MYB123 retained its nuclear localization when co-expressed with SBT5.2(b), suggesting that SBT5.2(b)-mediated MYB30 nuclear exclusion is specific (Figure 5a). Moreover, SBT5.2(b)-mediated specific nuclear exclusion of MYB30 was confirmed in Arabidopsis protoplasts (Figure 5b).
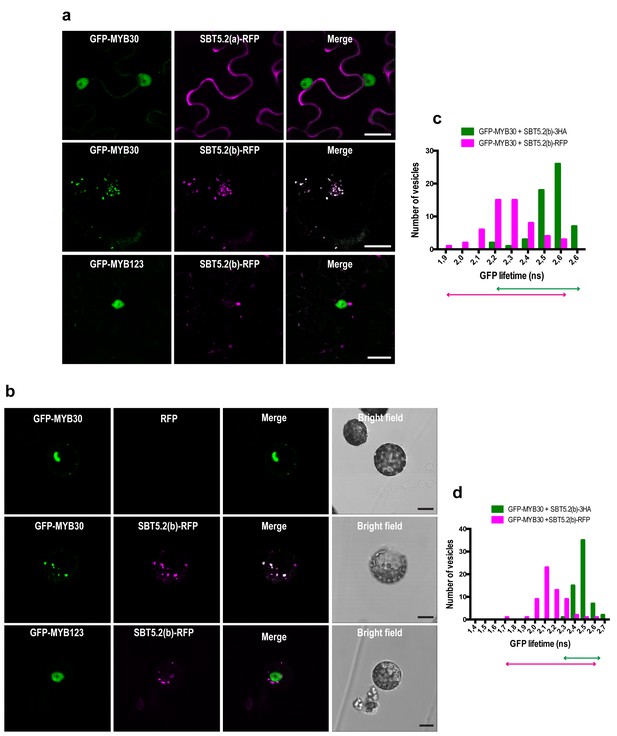
SBT5.2(b) mediates retention of MYB30 in intracellular vesicles.
(a) Confocal images of epidermal N. benthamiana cells 36 hr after Agrobacterium-mediated transient expression of the indicated constructs. (b) Confocal images of Arabidopsis protoplasts 16 hr after transformation of the indicated constructs. (c,d) GFP lifetime distribution of GFP-MYB30 in N. benthamiana cells (c) or Arabidopsis protoplasts (d) expressing SBT5.2(b). Histograms show the number of vesicles according to GFP-MYB30 lifetime classes in the presence of SBT5.2(b)-HA (green bars) or SBT5.2(b)-RFP (magenta bars). The degree of overlap of GFP lifetime distribution is represented with magenta (SBT5.2(b)-RFP) and green (SBT5.2(b)-HA) arrows. Bars = 10 µm.
We next sought out to confirm the interaction between MYB30 and SBT5.2 in plant cells. We focused on the study of the interaction between MYB30 and SBT5.2(b) because we were unable to detect a subcelullar co-localisation between MYB30 (nuclear) and SBT5.2(a) (secreted) (Figure 5a,b), which is a first requisite for the study of protein-protein interactions. The physical interaction between SBT5.2(b) and MYB30 was investigated in FRET-FLIM assays using GFP- (donor) and RFP- (acceptor) tagged MYB30 and SBT5.2(b), respectively. In order to avoid potential changes in GFP lifetime due to differences in the molecular environments of two distinct subcellular compartments [MYB30 being nuclear when expressed alone or in vesicle-like structures when co-expressed with SBT5.2(b)], the subcellular localization of GFP-tagged MYB30 when co-expressed with non-fluorescent HA-tagged, or an untagged version of SBT5.2(b), was therefore investigated. Importantly, both SBT5.2(b)-HA and untagged SBT5.2(b), which are not able to act as acceptors for GFP fluorescence, also led to MYB30 retention in intracellular vesicle-like structures (Figure 5—figure supplement 1a,b). A significant reduction of GFP lifetime was observed when GFP-MYB30 was co-expressed with RFP-tagged SBT5.2(b) as compared to co-expression with SBT5.2(b)-HA, both in N. benthamiana epidermal cells and in Arabidopsis protoplasts, thus confirming the physical interaction between the two proteins in intracellular vesicles (Figure 5c,d; Table 1). This interaction did not depend on the integrity of SBT5.2(b) catalytic triad, as shown by the reduced GFP lifetime of GFP-MYB30 when co-expressed with RFP-tagged SBT5.2(b)H171A (Table 1; Figure 5—figure supplement 1c).
FRET-FLIM analysis shows that MYB30 physically interacts with SBT5.2(b) at intracellular vesicles.
Donor | Acceptor | Lifetime* | SD† | N‡ | E§ | p-value# |
---|---|---|---|---|---|---|
N. benthamiana | ||||||
GFP-MYB30 | SBT5.2(b)-HA | 2.552 | 0.013 | 57 | - | |
GFP-MYB30 | SBT5.2(b)-RFP | 2.274 | 0.019 | 54 | 10.86 | 5.8 × 10−21 |
GFP-MYB30 | - | 2.669 | 0.009 | 82 | ||
GFP-MYB30 | SBT5.2(b)362-730-RFP | 2.271 | 0.016 | 51 | 14.91 | 3.8 × 10−49 |
GFP-MYB30 | SBT5.1(b)405-780-RFP | 2.592 | 0.018 | 44 | 2.86 | 4.2 × 10−05 |
GFP-MYB123 | - | 2.570 | 0.013 | 59 | ||
GFP-MYB123 | SBT5.2(b)362-730-RFP | 2.544 | 0.013 | 58 | 1.00 | 0.17 |
A. thaliana | ||||||
GFP-MYB30 | SBT5.2(b)-HA | 2.491 | 0.009 | 60 | - | |
GFP-MYB30 | SBT5.2(b)-RFP | 2.151 | 0.018 | 60 | 13.65 | 1.2 × 10−33 |
GFP-MYB30 | SBT5.2(b)H171A-HA | 2.473 | 0.012 | 60 | - | |
GFP-MYB30 | SBT5.2(b)H171A-RFP | 2.115 | 0.023 | 60 | 14.49 | 7.8 × 10−26 |
-
* Mean lifetime in nanoseconds
-
† Standard deviation
-
‡ Total number of measured vesicles
-
§ Percentage of FRET efficiency (E = 1 - τDA/τD) calculated by comparing the lifetime of the donor in the presence of the acceptor (τDA) with its lifetime in the absence of the acceptor (τD).
-
# p value of the difference between the donor lifetimes in the presence and in the absence of the acceptor (t-test)
The identification of a partial SBT5.2 cDNA clone in yeast suggested that the MYB30-SBT5.2(b) interaction is mediated by the C-terminus of SBT5.2(b). In order to confirm this idea, a truncated SBT5.2(b) version containing the C-terminal end of the protein [SBT5.2(b)362-730] fused to the RFP was generated and transiently expressed in N. benthamiana. SBT5.2(b)362-730-RFP presents a nucleocytoplasmic localization and colocalises with MYB30 in the nucleus (Figure 5—figure supplement 2a). A significant reduction of the average GFP lifetime was measured in nuclei coexpressing GFP-MYB30 and SBT5.2(b)362-730-RFP, as compared to nuclei expressing GFP-MYB30 alone (Figure 5—figure supplement 2d; Table 1), confirming that the C-terminus of SBT5.2(b) is sufficient for the interaction with MYB30. The specificity of this observation was highlighted by the lack of interaction between GFP-MYB30 and the equivalent C-terminal domain of the closest Arabidopsis SBT5.2 homolog, SBT5.1, (SBT5.1405-780-RFP) (Figure 5—figure supplement 2b,e; Table 1). Moreover, no significant reduction of the average GFP lifetime was detected between nuclei expressing the unrelated TF MYB123 [whose nuclear localization was not affected in the presence of full length SBT5.2(b) (Figure 5a,b)], when expressed alone or together with SBT5.2(b)362-730-RFP, despite the nuclear co-localization of the two proteins (Figure 5—figure supplement 2c,f; Table 1).
Together, our data confirms that MYB30 specifically interacts with SBT5.2(b) at vesicle-like structures. This interaction is mediated by SBT5.2(b) C-terminus, does not require an intact SBT5.2(b) catalytic triad and results in MYB30 nuclear exclusion.
An N-terminal myristoylation site in SBT5.2(b) determines its localization to endosomes and MYB30 nuclear exclusion
We next sought to determine the nature of the intracellular vesicle-like structures where SBT5.2(b) resides. Given the mobile character and varied sizes of these vesicles, we conducted co-localization experiments with VHA-a1 and SYP61, two markers for the trans-Golgi network/early endosomes (TGN/EE) (Dettmer et al., 2006; Tanaka et al., 2009) and ARA6 and SYP21, two markers for late endosomes/multivesicular bodies (LE/MVB) (Ueda et al., 2004; Uemura et al., 2004). Colocalization with of SBT5.2(b) with these endosomal markers was clearly observed in N. benthamiana leaves (Figure 6a, Figure 6—figure supplement 1). In contrast, no colocalization of SBT5.2(b)-RFP with the Golgi marker GmMan-GFP (Nelson et al., 2007) was detected (Figure 6a).
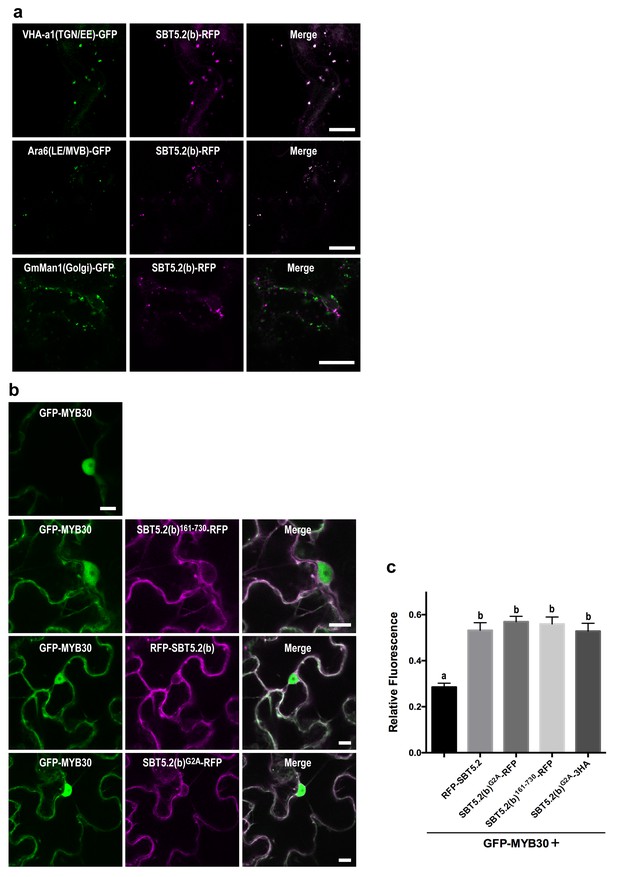
An N-terminal myristoylation site in SBT5.2(b) is required for its localization to endosomes and MYB30 nuclear exclusion.
(a,b) Confocal images of epidermal N. benthamiana cells 36 hr after Agrobacterium-mediated transient expression of the indicated constructs. Bars = 10 µm. (a) SBT5.2(b) localizes to endosomal vesicles. TGN/EE: trans-Golgi network/early endosomes; LE/MVB: late endosomes/multivesicular bodies. (b) A free N-terminal myristoylation site in SBT5.2(b) mediates its localization to endosomes and retention of MYB30 in endosomal vesicles. (c) Relative fluorescence values of cytoplasmic MYB30, expressed alone or with the indicated SBT5.2(b) versions, represented as ratios between cytoplasmic and nuclear fluorescence values in individual cells. Mean and SEM values were calculated from two independent experiments in which fifteen fluorescence measurements were taken per experiment and construct combination. Lowercase letters indicate statistically significant differences as determined by Bonferroni-corrected p-values (p<0.001) obtained after ANOVA and subsequent LSD post-hoc test.
The nucleocytoplasmic subcellular localization of SBT5.2(b)362-730 suggested that the N-terminal region of SBT5.2(b) is required for its localization to endosomes (Figure 5—figure supplement 2). In order to further test this idea, an N-terminal deletion of SBT5.2(b) was generated [SBT5.2(b)162-730]. RFP-tagged SBT5.2(b)162-730 indeed localized to the cytoplasm (Figure 6b). Furthermore, an N-terminally tagged RFP fusion of SBT5.2(b) also presented a cytoplasmic localization (Figure 6b), suggesting that a free SBT5.2(b)N-terminus is necessary for SBT5.2(b) endosomal targeting. Close inspection of the SBT5.2(b)N-terminal region uncovered the presence of a putative myristoylation site (MGSASSA; Figure 2—figure supplement 1c). A SBT5.2(b) version in which the putatively myristoylated Gly2 residue was mutated to Ala [SBT5.2(b)G2A] also localized to the cytoplasm (Figure 6b), confirming the importance of N-myristoylation for SBT5.2(b) endosomal targeting. Finally, co-expression of SBT5.2(b)162-730-RFP, RFP-SBT5.2(b) or SBT5.2(b)G2A RFP with GFP-MYB30 did not affect MYB30 nuclear targeting (Figure 6b). Interestingly, MYB30 accumulation in the cytoplasm was enhanced in the presence of these SBT5.2(b) versions, consistent with the presence in the three proteins of an intact SBT5.2 C-terminal domain that mediates the interaction with MYB30 (Figure 6c). An HA-tagged SBT5.2(b)G2A version induced the same effect confirming that the enhanced detection of GFP-MYB30 in the cytoplasm is not an artefact due the presence of a second fluorophore. Together, our data strongly suggest that a free N-terminal myristoylated residue is responsible of SBT5.2(b) targeting to the endosomes and of MYB30 retention in endosomal vesicles.
In order to further characterize the simultaneous localization of SBT5.2(b) to both early and late endosomes, HA-tagged versions of either SBT5.2(a), SBT5.2(b) or SBT5.2(b)G2A were co-expressed with both GFP-tagged VHA-a1 and RFP-tagged ARA6. When expressed with SBT5.2(a), both subcellular markers conserved their distinct subcellular localization in TGN/EE and LE/MVB, respectively (Figure 7). In contrast, co-expression with SBT5.2(b) led to co-localization of VHA-a1 and ARA6 in the same endosomal compartment, strongly suggesting that SBT5.2(b) is able to interfere with endosomal trafficking. Myristoylation of SBT5.2(b) appears to be essential to this effect, since expression of SBT5.2(b)G2A did not affect the discrete endosomal populations tagged by VHA-a1 or ARA6 (Figure 7).
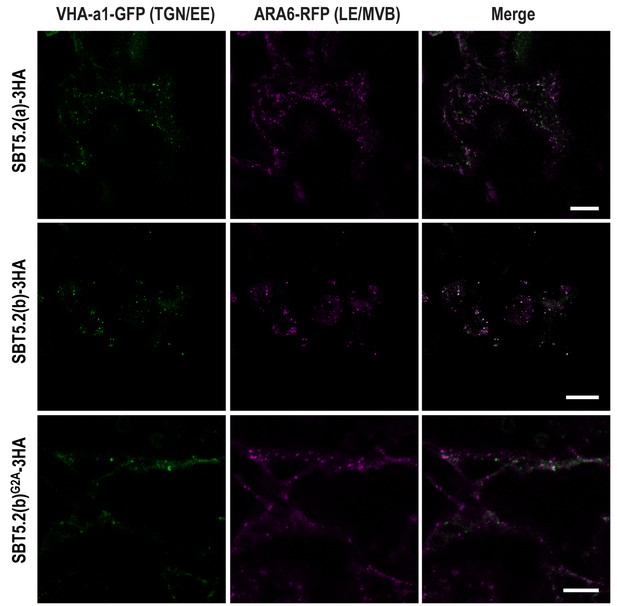
SBT5.2(b) leads to the formation of hybrid endosomal compartments in a myristoylation-dependent manner.
Confocal images of epidermal N. benthamiana cells 36 hr after Agrobacterium-mediated transient expression of the indicated constructs. TGN/EE: trans-Golgi network/early endosomes; LE/MVB: late endosomes/multivesicular bodies. Bars = 10 µm.
SBT5.2(b) attenuates MYB30-mediated responses to bacterial infection
To investigate the function of SBT5.2 in the plant response to bacterial inoculation, we used Arabidopsis sbt5.2 null mutants, sbt5.2–1 (SALK_012113) and sbt5.2–2 (SALK_132812C), both containing a T-DNA insertion in the last exon of SBT5.2. Despite the severe reduction of SBT5.2 expression in the mutant lines (Figure 8—figure supplement 1), no obvious macroscopic phenotype was observed in these plants. The phenotype of these lines in response to bacterial inoculation was next analysed. Similar to MYB30-overexpressing (MYB30OE), sbt5.2 mutant plants showed stronger HR cell death symptoms after inoculation with Pst AvrRpm1 as compared to Col-0 wild-type plants (Figure 8a). This phenotype was quantified by ion leakage measurements in leaf disk assays. Conductivity values measured in sbt5.2 and MYB30OE plants were significantly higher than those displayed by Col-0 wild type plants after bacterial inoculation (Figure 8b). In agreement with faster HR development, sbt5.2 plants showed increased resistance in response to inoculation with Pst AvrRpm1, as compared to wild-type plants (Figure 8c), confirming the role of SBT5.2 as a negative regulator of plant defence.
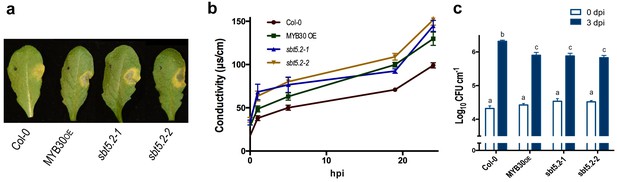
SBT5.2(b) is a negative regulator of resistance and HR responses in Arabidopsis in response to bacterial inoculation.
(a) Symptoms developed by the indicated Arabidopsis lines 60 hpi with Pst AvrRpm1 (2 × 106 cfu/ml). The pictures are representative of three independent experiments in which 4 plants of each line were infiltrated. (b) Quantification of cell death by measuring electrolyte leakage of the indicated Arabidopsis lines in a time course of 24 hr. Plants were inoculated with Pst AvrRpm1 (5 × 106 cfu/ml). Mean and SEM values were calculated from four independent experiments in which three plants were inoculated (four leaves/plant). (c) Growth of Pst AvrRpm1 in the indicated Arabidopsis lines. Bacterial growth 0 (white bars) and three days (blue bars) was measured after inoculation (5 × 105 cfu/ml). Mean bacterial densities were calculated from 6 independent experiments with 6 individual plants (4 leaves/plant). Statistical differences using multiple factor analysis of variance (ANOVA) (p<0.001) are indicated by letters.
Importantly, sbt5.2 mutant plants displayed higher expression of MYB30 VLCFA-related target genes FDH and CER2 (Raffaele et al., 2008) as compared to Col-0 wild-type plants 1 hr after inoculation (Figure 9a). Moreover, this phenotype was abolished in the myb30 mutant background (sbt5.2 myb30; Figure 9a) and correlated with loss of increased HR in the sbt5.2 myb30 double mutant (Figure 9b). Together, these data confirm that SBT5.2 negatively regulates Arabidopsis defence through repression of MYB30 transcriptional activity.
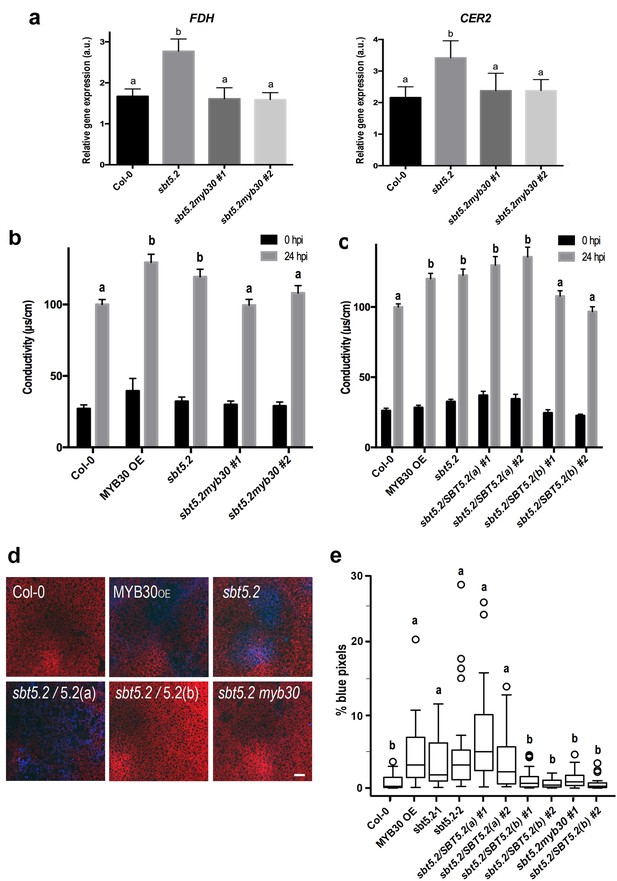
SBT5.2(b) attenuates MYB30-dependent transcriptional activation of VLCFA-related genes and hypersensitive cell death.
(a) Expression analysis of the MYB30 target genes FDH and CER2 in the indicated Arabidopsis lines 1 hr after inoculation with Pst AvrRpm1 (5 × 107 cfu/ml). Expression values of the individual genes were normalized using SAND family as internal standard. Mean and SEM values were calculated from 3 independent experiments (4 replicates/experiment). Statistical significance according to a Student’s t-test (p<0.05) is indicated by letters. (b,c) Quantification of cell death by measuring electrolyte leakage of the indicated Arabidopsis lines before (black bars) and 24 hr after (gray bars) inoculation with Pst AvrRpm1 (5 × 106 cfu/ml). Mean and SEM values were calculated from four independent experiments (three plants/experiment and four leaves/plant) and related to the value displayed by wild-type Col-0 plants, which is set at 100%. Statistical differences using multiple factor analysis of variance (ANOVA) (p<0.01) are indicated by letters. (d) Representative pictures of accumulation of phenolic compounds (blue coloration indicative of cell death) in the indicated Arabidopsis lines detected by epifluorescence 24 hr after inoculation with Pst AvrRpm1 (2 × 105 cfu/ml). Bar = 100 µm. (e) Blue pixels in the indicated lines were quantified using Image-Pro Plus and are shown as the percentage of the total number of blue pixels in each image. Boxplots are as follows: box limits, values between first and third quartiles; middle bar, median. Whiskers cover 1.5 times the interquartile distance and circles represent extreme values. Lowercase letters indicate significant differences with respect to the sbt5.5–2 line as determined by Bonferroni-corrected p-values (p<0.01) obtained after ANOVA and subsequent LSD post-hoc test.
In sbt5.2 mutant plants, expression of both SBT5.2(a) and SBT5.2(b) is affected. To obtain additional proof of the negative role specifically played by SBT5.2(b) on MYB30-mediated responses, sbt5.2 mutant plants were transformed with an HA-tagged version of either SBT5.2(a) or SBT5.2(b) under the control of the 35S promoter. Expression of SBT5.2 gene and protein was monitored by qRT-PCR and Western Blot analysis in two independent homozygous T4 lines for each construct (Figure 8—figure supplement 1b,c). Importantly, the increased HR phenotype displayed by the sbt5.2 mutant was specifically suppressed in sbt5.2 plants overexpressing SBT5.2(b), but not SBT5.2(a) (Figure 9c).
In order to obtain in vivo confirmation of the negative control of defence-related cell death exerted by SBT5.2(b), the above described lines were inoculated with a low dose of HR-inducing Pst AvrRpm1. Enhanced accumulation of phenolic compounds, characteristic of HR cell death, was detected in MYB30OE ans sbt5.2 mutant lines as compared to wild-type Col-0 and this phenotype was suppressed both in sbt5.2 myb30 double mutants and by overexpressing SBT5.2(b), but not SBT5.2(a), in the sbt5.2 mutant background (Figure 9d,e). Overall our data demonstrate that, in agreement with SBT5.2(b)-mediated retention of MYB30 in endosomes, negative regulation of MYB30-mediated defence-related cell death is specifically controlled by SBT5.2(b).
Discussion
AS is a fundamental process that allows generating a large number of mRNA and protein isoforms from a genome of limited size (Graveley, 2005). Moreover, AS plays crucial functions in eukaryotic cells, as it determines the binding properties, intracellular localisation, enzymatic activity, protein stability and posttranslational modifications of a large number of proteins (Stamm et al., 2005). In humans, the importance of AS is clearly highlighted by the fact that about 15% of genetic hereditary diseases are caused by mutations that affect splicing (Kornblihtt et al., 2013). In plants, AS plays fundamental roles in regulating plant growth, development, and responses to environmental signals (Staiger and Brown, 2013). A genome-wide transcriptomic analysis in Arabidopsis plants inoculated with bacteria uncovered a surprisingly large number of AS events (Howard et al., 2013). Although we are still far from understanding the functional implications of this transcriptome complexity, the function of numerous plant resistance genes, encoding immune receptors, appears to be regulated by AS (Yang et al., 2014; Gassmann, 2008). To our knowledge, our work represents the first described example of AS affecting the function of a protein of the subtilase family. AS of SBT5.2 results in the production of the atypical subtilase SBT5.2(b), uncovering a novel mode of regulation of defence reactions and contributing to further our understanding of the varied roles of AS in the control of plant immunity.
Following bacterial inoculation, the respective induction and repression of SBT5.2(b) and SBT5.2(a) expression suggests a functional role for SBT5.2(b) during defence regulation. This idea is reinforced by the observed co-regulation of SBT5.2(b) and MYB30 expression after bacterial treatment. Despite their pervasiveness, our current understanding of the functions of plants subtilases is still limited. Different studies suggest a role in both general protein turnover and regulation of plant development or responses to environmental cues (Schaller et al., 2012; Figueiredo et al., 2014). The first example of a plant subtilase potentially acting during plant-pathogen interactions was reported in tomato, where the expression of the subtilases P69B and P69C was induced by pathogen infection and treatment with salycilic acid (Jordá et al., 1999; Tornero et al., 1997). More recently, the Arabidopsis subtilase SBT3.3 was found to be involved in the regulation of immune signalling through chromatin remodelling of defence-related genes associated with the activation of immune priming (Ramírez et al., 2013).
Plant subtilases are usually synthesized in the form of preproprotein precursors, translocated via an N-terminal ER-targeting SP into the endomembrane system (Schaller et al., 2012). In addition, using mass spectrometry (Cedzich et al., 2009) and structural analyses (Murayama et al., 2012), plant subtilases were shown to be glycosylated in the secretory pathway and to accumulate extracellularly (Schaller et al., 2012). In agreement with the preproprotein structure, the maturation of the active enzyme from its inactive precursor requires at least two processing steps. After cleavage of the SP, subtilases are ultimately activated by cleavage of the PD producing the mature active enzyme (Taylor et al., 1997). Processing of the PD, an auto-inhibitor domain of plant subtilases (Nakagawa et al., 2010; Meyer et al., 2016), is an intramolecular autocatalytic reaction that occurs late in the ER or in the early Golgi (Cedzich et al., 2009). Here, we show that AS of the SBT5.2 gene has important implications for the subcellular localization and activity of the resulting isoforms, despite the nearly total conservation between the two protein sequences. Consistent with harbouring all canonical features of a subtilase, we confirmed that SBT5.2(a) enters the secretory pathway, where it is glycosylated, and is secreted to the extracellular space as shown before (Engineer et al., 2014; Kaschani et al., 2012). SBT5.2 was previously described as a negative regulator of stomatal density under high CO2 conditions through cleavage of the extracellular pro-peptide ligand EPF2 in the apoplast (Engineer et al., 2014). In contrast, in agreement with its lacking a SP and the first five amino acids of the PD, SBT5.2(b) does not enter the secretory pathway, its PD is thus not cleaved and may fold onto SBT5.2(b) catalytic domain inhibiting its protease activity. Indeed, despite the presence of PGSs and catalytic residues, we were unable to detect SBT5.2(b) glycosylation or protease activity. We also showed that MYB30 accumulation in planta is not affected by SBT5.2(a) or SB5.2(b), indicating that these proteins do not proteolytically cleave the TF. In the case of catalytically active SBT5.2(a), this can be explained by the lack of co-localization of both proteins. Despite their subcellular co-localization and physical interaction, lack of modification of MYB30 accumulation in the presence of SBT5.2(b) is consistent with SBT5.2(b) being inactive as a protease, as underlined by the finding that both wild-type SBT5.2(b) and catalytic mutant SBT5.2(b)H171A are able to interact with and retain MYB30 at endosomal vesicles. These results suggest an alternative mode of action of SBT5.2(b) on MYB30 activity, likely related to SBT5.2(b)-mediated MYB30 nuclear exclusion. Examples of proteases playing proteolysis-independent functions have been described in mammals. The proprotein convertase subtilisin/kexin type 9 (PCSK9) plays a major regulatory role in cholesterol homeostasis but does not require its catalytic activity to induce degradation of low-density lipoproteins (LDLs) (McNutt et al., 2007). In addition, γ-secretase, an aspartyl protease that performs the final proteolytic cleavage step in the processing cascade of the β-amyloid precursor protein (βAPP) (Vassar et al., 1999), displays proteolysis-independent functions during the regulation of βAPP maturation and trafficking (Wrigley et al., 2005).
SBT5.2(b) localises to both early and late endosomal compartments and this requires N-terminal myristoylation of the protein. Since co-expression of SBT5.2(b), but not SBT5.2(a), with both early and late endosome markers leads to colocalisation of both markers, it is tempting to speculate that SBT5.2(b) is able to interfere with endosomal trafficking leading to the formation of hybrid endosomes. In mammals, expression of constitutively active Rab5 blocks the conversion of early to late endosomes, giving rise to hybrid endosomal compartments and deregulation of autophagy (Rink et al., 2005). Similarly, overexpression of Arabidopsis sorting nexin SNX2b leads to the formation of large SNX2-containing endosome aggregations, which inhibits vesicle trafficking (Phan et al., 2008).
SBT5.2(b)-mediated regulation of MYB30 activity supports the emerging idea that endosomal trafficking pathways are not only central regulators of plasma membrane protein homeostasis but also control multiple signalling pathways, including those involved in plant disease resistance (Reyes et al., 2011). Enhanced susceptibility of plants impaired in regulators of endosome trafficking emphasizes the importance of endocytic processes in establishing defence (Lu et al., 2012). In addition, plasma membrane receptors that sense apoplastic microbes are immune-related cargos of plant trafficking pathways (Beck et al., 2012; Ben Khaled et al., 2015), and a number of intracellular immune receptors have been shown to constitutively localize to endomembranes (Takemoto et al., 2012; Engelhardt et al., 2012), further underlining the prominent role of endomembrane systems in determining plant resistance. Although the role of endosomes in implementing defence signalling is not yet well understood, they have been proposed as central subcellular sites for orchestration of the HR. Indeed, relocalisation of the potato resistance protein R3a from the cytoplasm to endosomal compartments is required to trigger the HR (Engelhardt et al., 2012). The importance of these compartments during immunity is further underlined by the fact that both plant and animal pathogens produce inhibitory effector proteins that specifically target endomembrane trafficking. For example, the Arabidopsis ADP ribosylation factor (ARF)-Guanine exchange factor (GEF) MIN7 is targeted and degraded by the bacterial effector HopM1 altering secretory trafficking (Nomura et al., 2006, 2011). In mammalian cells, the effector PipB2 from the bacterial pathogen Salmonella enterica causes a specific redistribution of late endosomes/lysosomes (LE/Lys) compartments to the cell periphery promoting formation of Salmonella-induced filaments (Sifs) (Knodler and Steele-Mortimer, 2005).
Our finding that SBT5.2(b) mediates MYB30 nuclear exclusion resulting in attenuation of MYB30 gene expression and HR, is in agreement with several reports describing controlled nuclear localisation as an efficient mechanism to regulate TF activity in eukaryotic cells. Subcellular compartmentalization of the E2F TF family, either in the nucleus or in the cytoplasm, is used to control cell cycle in differentiated skeletal cells (Gill and Hamel, 2000). p53, a tightly regulated animal TF that acts as a hub for numerous signalling pathways including apoptosis, shifts from the nucleus to the cytoplasm in the presence of HDM2, where it has important roles that are independent of its transcriptional activity (Boyd et al., 2000; Green and Kroemer, 2009). Similarly, alternative functions for MYB30 in endosomal vesicles, other than HR regulation, cannot be excluded at this stage. In addition, nuclear import of 'dormant' TFs plays important roles in the regulation of gene expression. Upon exposure to environmental stresses, several membrane-bound TFs have been shown to be proteolytically activated by either ubiquitin-mediated proteasome activities or by specific membrane-bound proteases (Hoppe et al., 2001), which may facilitate triggering quick transcriptional responses to ensure plant survival under stressful conditions (Kim et al., 2006; Seo et al., 2010). Notably, subtilases are known to be involved in the release of 'dormant' membrane-bound TFs. Arabidopsis SBT6.1 is able to cleave the ER-located TF bZIP17 that, once released, moves to the nucleus to activate transcription in response to salt stress (Liu et al., 2007). Finally, nuclear exclusion by localization to small vesicle-like structures has been reported as a negative regulatory mechanism of TF activity. Indeed, the small interference protein MIF1, promotes nuclear exclusion of the TF ZHD5 that regulates flower architecture and leaf development. As a result, ZHD5 is relocalised into cytoplasmic vesicle-like structures, which interferes with is transcriptional activity (Hong et al., 2011).
During the last few years, different regulatory mechanisms of MYB30-mediated HR have been uncovered (Raffaele and Rivas, 2013), including spatio-temporal control of MYB30 activity through the action of the secreted phospholipase AtsPLA2-α (that specifically relocalises to the nucleus in the presence of MYB30 [Froidure et al., 2010]) and the RING-type E3 ligase MIEL1 (that ubiquitinates MYB30 and leads to its proteasomal degradation [Marino et al., 2013]). SBT5.2(b)-mediated nuclear exclusion of MYB30 represents an additional regulatory mode of the activity of this TF, underlining the complexity of the regulatory modes of defence-related plant cell death responses. This intricate regulation of MYB30 is reminiscent of the tight control exerted on animal TFs such as p53 that is also multi-regulated through varied modes including protein-protein interactions, ubiquitination and, notably, nuclear exclusion. This sophisticated fine-tuning, which provides an efficient means to regulate fundamental cellular processes, appears as a general feature underlying the transcriptional control in eukaryotic cells.
Materials and methods
Cloning procedures
Request a detailed protocolPlasmids used in this study were constructed by Gateway technology (GW; Invitrogen, Waltham, MA, USA) following the instructions of the manufacturer. PCR products flanked by the attB sites were recombined into the pDONR207 vector (Invitrogen) via a BP reaction to create the corresponding entry clones with attL sites. Inserts cloned into the entry clones were subsequently recombined into the destination vectors via an LR reaction to create the expression constructs.
A fusion of fluorescent proteins to MYB30, SBT5.2(b), SBT5.2(b)G2A, VHA-a1, SYP61, ARA6 and SYP21 was accomplished using a multisite GATEWAY cloning strategy (Invitrogen) described previously (Serrano et al., 2014). Briefly, the full-length open reading frames of the indicated proteins were amplified from a plasmid template and cloned into the donor vector pBSDONR P1-P4 or the pBSDONR P4r-P2 by BP reaction (ampicillin-resistant vectors derived from pDONR221 from Invitrogen) (Gu and Innes, 2011). eGFP (Cormack et al., 1996) and RFP (Campbell et al., 2002) were cloned into pBSDONR P1-P4 for N-terminal fusion and into pBSDONR P4r-P2 for C-terminal fusion. To fuse SBT5.2(a), SBT5.2(b), SBT5.2(b)162-730,SBT5.2(b)G2A, VHA-a1, SYP61, ARA6 and SYP21 with the epitope tags, the P1-P4 clones were mixed with corresponding P4r-P2 and the desired destination vectors and recombined using Gateway LR Clonase II (Invitrogen). All the above pBSDONR constructs were recombined with the destination vector pEarleyGate100 (Earley et al., 2006) with the exception of SBT5.2(a) and SBT5.2(b), for which the corresponding pBSDONR constructs were recombined with the steroid-inducible destination vector pBAV154 (Vinatzer et al., 2006).
For yeast assays, the GAL4-BD-MYB30ΔAD fusion was previously described (Froidure et al., 2010). AD-SBT5.2(b)628-730 construct was generated from recombination of the corresponding entry constructs with the pGAD-AD-GW vector (Froidure et al., 2010).
Point mutations were generated using the QuickChange mutagenesis kit (Stratagene, Santa Clara, CA, USA) using the pENTR-SBT5.2 as a template and following the manufacturer’s instructions. Primers used for mutagenesis are shown in Supplementary file 1.
Yeast assays
Request a detailed protocolThe yeast two-hybrid screen and methods used for identification of SBT5.2 were previously described (Froidure et al., 2010). Briefly, an Arabidopsis thaliana Gal4 yeast two-hybrid cDNA prey library (MatchMaker; Clontech) was generated from mRNA isolated from leaves of four-week-old plants (Ws-4 ecotype) syringe-infiltrated with the Xanthomonas campestris pv. campestris 147 strain. An MYB30 version deleted from its C-terminal activation domain (amino acids 1 to 234) was used as bait for screening 2 × 106 independent transformants exhibiting His auxotrophy on selective plates.
5’ RACE assays
Request a detailed protocol5’ ends of SBT5.2 mRNA were determined using the GeneRacerTM RACE Ready kit (Invitrogen, France) according to manufacturers’ instructions, using RNA from Col-0 leaves and gene specific primers indicated in Supplementary file 1. PCR products were cloned in pGEM-T Easy vector (Promega Corporation, Fitchburg, WI, USA) and sequenced.
Plant and bacterial materials
Request a detailed protocolArabidopsis lines used in this study were in the Columbia background and grown in Jiffy pots under controlled conditions in a growth chamber at 21°C, with a 9-hr light period and a light intensity of 190 µmol.m−2.s−1. The MYB30ko line (SALK_122884) was reported before (Marino et al., 2013).
For transient expression of proteins in N. benthamiana, overnight bacterial cultures of Agrobacterium tumefaciens strain C58C1 or GV3101 carrying the vector of interest were harvested by centrifugation. Cells were resuspended in induction buffer (10 mM MgCl2, 10 mM MES, pH 5.6, and 150 µM acetosyringone) to an OD600 of 0.5. After 2 hr at 22°C, cells were infiltrated into leaves of four-week-old N. benthamiana plants. Two days after A. tumefaciens infiltration, leaf discs used for experiments were harvested and processed, or frozen immediately in liquid nitrogen and stored at –80°C. For tunicamycin treatment, 24 hr after Agrobacterium-mediated transient expression, leaf discs of N. benthamiana leaves were incubated in a solution of 10 µM tunicamycin for 20 hr at room temperature and later frozen in liquid nitrogen before processing.
When testing the effect of SBT5.2 proteins on MYB30 accumulation, to minimize differences in protein expression, which are inherent to transient assays, MYB30 was co-expressed with SBT5.2(a), or SBT5.2(b), and the respective catalytic mutant side by side in the same N. benthamiana leaf. To avoid ex planta protein degradation, the leaves were pre-treated with the protease inhibitor PMSF (1 mM) 30 min before harvesting the tissue for protein extraction.
Arabidopsis four-week-old plants were kept at high humidity 12 hr before inoculation and injected with a bacterial suspension of Pst AvrRpm1 at the indicated bacterial densities using a blunt syringe on the abaxial side of the leaves. For determination of in planta bacterial growth, the leaf samples were harvested 0 and three days after inoculation and ground on sterile water. A predetermined dilution for each sample was plated on King’s B medium and incubated at 28°C for two days. The data were submitted to a statistical analysis using Statgraphics Centurion XV.II Professional Software (Statpoint Technologies Inc., Warrenton, VA, USA). Normality of residues was verified by the Kolmogorov-Smirmov test. The effect of the genotype was tested by Multiple Factor ANOVA.
Fluorescence microscopy, FRET-FLIM and data analysis
Request a detailed protocolGFP and RFP fluorescence was analyzed with a confocal laser scanning microscope (TCS SP2-AOBS; Leica) using a x63 water immersion objective lens (numerical aperture 1.20; PL APO). GFP fluorescence was excited with the 488 nm ray line of the argon laser and recorded in one of the confocal channels in the 505 to 530 nm emission range. RFP fluorescence was excited with the 561 nm line ray of the He-Ne laser and detected in the range between 595 and 620 nm. Images were acquired in the sequential mode using Leica LCS software (version 2.61).
In order to quantify the fluorescence of MYB30 in the nucleus and cytoplasm, fluorescence intensity was measured using Leica LCS software (version 2.61). Region of interest (ROIs) were defined for each photograph and the mean value was taken as a fluorescence measure. Five fluorescence measures were obtained from 6 photographs taken from two independent experiments (n = 30).
The fluorescence lifetime of the donor was experimentally measured in the presence and absence of the acceptor. The FRET efficiency (E) was calculated by comparing the lifetime of the donor in the presence (tDA) or absence (tD) of the acceptor: E = 1-(tDA)/(tD). Statistical comparisons between control (donor) and assay (donor + acceptor) lifetime values were performed by Student t test. FRET-FLIM measurements were performed using a FLIM system coupled to a streak camera (Krishnan et al., 2003). The light source (l = 850 nm) was a pulsed pulsed femtosecond IR laser (Spectra-Physics, USA). All images were acquired with a 60x oil immersion lens (Plan Apo 1.4 numerical aperture, IR) mounted on an inverted microscope (Eclipse TE2000E, Nikon, Japan) coupled to the FLIM system. The fluorescence emission was directed back out into the detection unit through a band pass filter. The detector was composed of a streak camera (Streakscope C4334, Hamamatsu Photonics, Japan) coupled with a fast and high sensitivity CCD camera (model C8800-53C, Hamamatsu). For each region of interest (vesicle and nucleus), average fluorescence decay profiles were plotted and lifetimes were estimated by fitting data with exponential function using a non-linear least-squares estimation procedure.
Deglycosylation experiments
Request a detailed protocolProteins were extracted in 50 mM Tris-HCl, pH 7.5, 150 mM NaCl, 10% [v/v] glycerol, 1 mM PMSF, and 1% plant protease inhibitor cocktail (Sigma-Aldrich, St Louis, MO, USA) and centrifuged at 14,000g for 10 min at 4°C. Proteins in the supernatant were denatured and then incubated with PNGase F or Endo H (New England Biolabs, Ipswich, MA, USA) following the instructions of the manufacturer. Deglycosylation reactions were performed for 30 min and stopped by adding SDS-PAGE loading buffer and boiling. Proteins were detected by Western blot using anti-HA antibodies as described below.
Concanavalin A purification
Request a detailed protocolProteins were extracted in 50 mM Tris-HCl, pH 7.5, 150 mM NaCl, 10% [v/v] glycerol, 1 mM PMSF, and 1% plant protease inhibitor cocktail (Sigma-Aldrich) and centrifuged at 14,000 g for 10 min at 4°C. The supernatant was equilibrated in concanavalin A buffer (0.2 M Tris-HCl pH 7.5, 1 M NaCl, 200 mM MgCl2, 200 mM CaCl2) and applied to concanavalin A-agarose resin from Canavalia ensiformis (Sigma-Aldrich) pre-equilibrated in concanavalin A buffer. After three steps of washing with concanavalin A buffer, glycosylated proteins were eluted in concanavalin A buffer supplemented with 0.75 M α-D-methyl-glucoside and 0.75 M α-D-methylmannoside. The presence of HA-tagged SBT5.2 in the eluted proteins was confirmed by Western blot using anti HA antibodies as described below.
Isolation of intercellular (apoplastic) fluid
Request a detailed protocolN. benthamiana leaves transiently expressing the proteins of interest were harvested 48 hr after agroinfiltration and infiltrated with water. Intercellular fluids (IF) were isolated by centrifugation at 3000 g as previously described (de Wit and Spikman, 1982).
Gel blot analysis
Request a detailed protocolAntibodies used for Western blotting were anti-HA-HRP (3F10, Roche, Germany, 1:5000), and PAP anti-rabbit-HRP (Sigma, 1:10,000). Proteins were visualized using the Immobilon kit (Millipore, Billerica, MA, USA) under standard conditions.
Transient transfection of Arabidopsis protoplasts
Request a detailed protocolThe isolation and transient transfection of leaf mesophyll cell protoplasts from Arabidopsis plants (four weeks-old) was performed at room temperature following published procedures (Yoo et al., 2007). A total of 10 μg plasmid DNA was used for each transfection experiment and plasmids were mixed in an equal ratio for cotransfections.
Fluorescein isothiocyanate(FITC)-labeled casein assay for proteolytic activity
Request a detailed protocolFITC casein was used for the detection of proteolytic activity (Twining, 1984). sbt5.2 Arabidopsis protoplasts transfected with SBT5.2(a), SBT5.2(a)H210A, SBT5.2(b), SBT5.2(b)H171A or empty vector were lysed and used for the activity assay. A concentration of 400 µg/ml of FITC casein was used in a final volume of 25 µl of 150 mM NaCl, 20 mM phosphate buffer pH 7.6. Samples were incubated for 1 hr at 25°C and fluorescence was measured at excitation 485 nm and emission 520 nm using a microtiter fluorimeter (FL600, Bio-Tek, Highland Park, VT, USA).
Quantification of cell death
Request a detailed protocolFor electrolyte leakage measurement, four leaf discs (6-mm diameter) were harvested at the indicated timepoints after plant inoculation, washed, and incubated at room temperature in 5 ml of distilled water before measuring conductivity. The production of phenolic compounds was monitored under UV light using a Zeiss Axioplan microscope 24 hr after inoculation.
RNA extraction and Q-RT-PCR analysis
Request a detailed protocolMaterial for RNA analysis was grounded in liquid nitrogen and total RNA was isolated using the Nucleospin RNA plant kit (Macherey-Nagel, Germany) according to the manufacturer’s recommendations. Reverse transcription was performed using 1.5 µg of total RNA. Real-time quantitative PCR was performed on a Light Cycler 480 II machine (Roche Diagnostics, France), using Roche reagents. Primers used for Q-RT-PCR are provided as Supporting Information. Relative expression was calculated as the ∆Cp between each gene and the internal controls SAND family (At2g28390). Average ∆Cp was related to the value of each gene in each line at time 0.
References
-
Knocking out the door to tunicamycin entryPNAS 108:11731–11732.https://doi.org/10.1073/pnas.1109035108
-
A moving view: subcellular trafficking processes in pattern recognition receptor-triggered plant immunityAnnual Review of Phytopathology 53:379–402.https://doi.org/10.1146/annurev-phyto-080614-120347
-
An intact HDM2 RING-finger domain is required for nuclear exclusion of p53Nature Cell Biology 2:563–568.https://doi.org/10.1038/35023500
-
Transcriptional control of plant defence responsesCurrent Opinion in Plant Biology 20:35–46.https://doi.org/10.1016/j.pbi.2014.04.004
-
Programmed cell death in the plant immune systemCell Death and Differentiation 18:1247–1256.https://doi.org/10.1038/cdd.2011.37
-
Catalytic triads and their relativesTrends in Biochemical Sciences 23:347–352.https://doi.org/10.1016/S0968-0004(98)01254-7
-
MYB transcription factors in ArabidopsisTrends in Plant Science 15:573–581.https://doi.org/10.1016/j.tplants.2010.06.005
-
Subtilisin-like proteases in plant-pathogen recognition and immune priming: a perspectiveFrontiers in Plant Science 5:739.https://doi.org/10.3389/fpls.2014.00739
-
Alternative splicing in plant defenseCurrent Topics in Microbiology and Immunology 326:219–233.https://doi.org/10.1007/978-3-540-76776-3_12
-
Nuclear import and DNA binding of the ZHD5 transcription factor is modulated by a competitive peptide inhibitor in ArabidopsisJournal of Biological Chemistry 286:1659–1668.https://doi.org/10.1074/jbc.M110.167692
-
Membrane-bound transcription factors: regulated release by RIP or RUPCurrent Opinion in Cell Biology 13:344–348.https://doi.org/10.1016/S0955-0674(00)00218-0
-
A genomic cluster containing four differentially regulated subtilisin-like processing protease genes is in tomato plantsJournal of Biological Chemistry 274:2360–2365.https://doi.org/10.1074/jbc.274.4.2360
-
Activity-based protein profiling of infected plantsMethods in Molecular Biology 835:47–59.https://doi.org/10.1007/978-1-61779-501-5_3
-
A membrane-bound NAC transcription factor regulates cell division in arabidopsisThe Plant Cell Online 18:3132–3144.https://doi.org/10.1105/tpc.106.043018
-
The Salmonella effector PipB2 affects late endosome/lysosome distribution to mediate Sif extensionMolecular Biology of the Cell 16:4108–4123.https://doi.org/10.1091/mbc.E05-04-0367
-
Alternative splicing: a pivotal step between eukaryotic transcription and translationNature Reviews Molecular Cell Biology 14:153–165.https://doi.org/10.1038/nrm3525
-
N-glycoprotein biosynthesis in plants: recent developments and future trendsPlant Molecular Biology 38:31–48.https://doi.org/10.1023/A:1006012005654
-
Catalytic activity is not required for secreted PCSK9 to reduce low density lipoprotein receptors in HepG2 cellsJournal of Biological Chemistry 282:20799–20803.https://doi.org/10.1074/jbc.C700095200
-
Functional characterization of propeptides in plant subtilases as intramolecular chaperones and inhibitors of the mature proteaseJournal of Biological Chemistry 291:19449–19461.https://doi.org/10.1074/jbc.M116.744151
-
Vertebrate protein glycosylation: diversity, synthesis and functionNature Reviews Molecular Cell Biology 13:448–462.https://doi.org/10.1038/nrm3383
-
Crystal structure of cucumisin, a subtilisin-like endoprotease from Cucumis melo LJournal of Molecular Biology 423:386–396.https://doi.org/10.1016/j.jmb.2012.07.013
-
Functional analysis of the cucumisin propeptide as a potent inhibitor of its mature enzymeJournal of Biological Chemistry 285:29797–29807.https://doi.org/10.1074/jbc.M109.083162
-
Plant endosomal trafficking pathwaysCurrent Opinion in Plant Biology 14:666–673.https://doi.org/10.1016/j.pbi.2011.07.009
-
N-terminal motifs in some plant disease resistance proteins function in membrane attachment and contribute to disease resistanceMolecular Plant-Microbe Interactions 25:379–392.https://doi.org/10.1094/MPMI-11-10-0272
-
Identification of a new pathogen-induced member of the subtilisin-like processing protease family from plantsJournal of Biological Chemistry 272:14412–14419.https://doi.org/10.1074/jbc.272.22.14412
-
Transcriptional networks in plant immunityNew Phytologist 206:932–947.https://doi.org/10.1111/nph.13286
-
Fluorescein isothiocyanate-labeled casein assay for proteolytic enzymesAnalytical Biochemistry 143:30–34.https://doi.org/10.1016/0003-2697(84)90553-0
-
Functional differentiation of endosomes in Arabidopsis cellsThe Plant Journal 40:783–789.https://doi.org/10.1111/j.1365-313X.2004.02249.x
-
Systematic analysis of SNARE molecules in Arabidopsis: dissection of the post-Golgi network in plant cellsCell Structure and Function 29:49–65.https://doi.org/10.1247/csf.29.49
-
Plant proteases: from phenotypes to molecular mechanismsAnnual Review of Plant Biology 59:191–223.https://doi.org/10.1146/annurev.arplant.59.032607.092835
-
Functional overexpression of gamma-secretase reveals protease-independent trafficking functions and a critical role of lipids for protease activityJournal of Biological Chemistry 280:12523–12535.https://doi.org/10.1074/jbc.M413086200
-
Alternative splicing in plant immunityInternational Journal of Molecular Sciences 15:10424–10445.https://doi.org/10.3390/ijms150610424
Article and author information
Author details
Funding
Institut National de la Recherche Agronomique (INRA)
- Irene Serrano
AgreenSkills fellowship, EU Marie-Curie FP7 COFUND People Programme (grant agreement n° 267196)
- Irene Serrano
Freach Ministry of National Education and Research
- Pierre Buscaill
French Laboratory of Excellence project 'TULIP' (ANR-10-LABX-41)
- Susana Rivas
French Laboratory of Excellence project 'TULIP' (ANR-11-IDEX-0002-02)
- Susana Rivas
The funders had no role in study design, data collection and interpretation, or the decision to submit the work for publication.
Acknowledgements
We thank Céline Remblière for help with plant transformation. We thank Roger Innes for kindly providing the multisite gateway system, as well as VHA-a1 and ARA6 cDNAs and GmMan1 construct. We are grateful to Paul Hurst for help with plant inoculation and conductivity assays. PB was funded by a grant from the French Ministry of National Education and Research. IS is supported by INRA (Institut National de la Recherche Agronomique) and an AgreenSkills fellowship within the EU Marie-Curie FP7 COFUND People Programme (grant agreement n° 267196). Our work is supported by the French Laboratory of Excellence project 'TULIP' (ANR-10-LABX-41; ANR-11-IDEX-0002-02).
Copyright
© 2016, Serrano et al.
This article is distributed under the terms of the Creative Commons Attribution License, which permits unrestricted use and redistribution provided that the original author and source are credited.
Metrics
-
- 2,314
- views
-
- 538
- downloads
-
- 26
- citations
Views, downloads and citations are aggregated across all versions of this paper published by eLife.
Citations by DOI
-
- 26
- citations for umbrella DOI https://doi.org/10.7554/eLife.19755