ppargc1a controls nephron segmentation during zebrafish embryonic kidney ontogeny
Abstract
Nephron segmentation involves a concert of genetic and molecular signals that are not fully understood. Through a chemical screen, we discovered that alteration of peroxisome proliferator-activated receptor (PPAR) signaling disrupts nephron segmentation in the zebrafish embryonic kidney (Poureetezadi et al., 2016). Here, we show that the PPAR co-activator ppargc1a directs renal progenitor fate. ppargc1a mutants form a small distal late (DL) segment and an expanded proximal straight tubule (PST) segment. ppargc1a promotes DL fate by regulating the transcription factor tbx2b, and restricts expression of the transcription factor sim1a to inhibit PST fate. Interestingly, sim1a restricts ppargc1a expression to promote the PST, and PST development is fully restored in ppargc1a/sim1a-deficient embryos, suggesting Ppargc1a and Sim1a counterbalance each other in an antagonistic fashion to delineate the PST segment boundary during nephrogenesis. Taken together, our data reveal new roles for Ppargc1a during development, which have implications for understanding renal birth defects.
https://doi.org/10.7554/eLife.40266.001Introduction
The vertebrate kidney develops from the intermediate mesoderm and can have two or three stages depending on the organism, where amphibians and fish develop a pronephros and mesonephros, while others like birds and mammals form these structures and also generate a metanephros (Saxén and Sariola, 1987). Roles of the kidney include regulation of osmolarity, fluid balance, and blood filtration. The kidney performs these tasks with functional units known as nephrons. Nephrons reabsorb or secrete precise amounts of essential molecules, ranging from amino acids to electrolytes, based on the dynamic physiological needs of the organism. Nephrons are divided into three main components: the filtration unit, a tubule, and a collecting duct (Romagnani et al., 2013). Nephron tubules are further subdivided into unique epithelial segments that perform the specialized tasks of reabsorption or secretion of discrete cargos. Ongoing advances have shed light on a number of the molecular pathways and gene expression signatures associated with nephron segment formation in kidney forms across species (Desgrange and Cereghini, 2015; Lindström et al., 2015; Lindström et al., 2018). Nevertheless, there are still many remaining questions about the genetic mechanisms that control segment fates and what specifies boundary formation between adjacent nephron segments.
The embryonic zebrafish pronephros is a tractable model to study the processes of nephron segmentation (Gerlach and Wingert, 2013). Completely segmented at just 24 hours post fertilization (hpf), the pronephros is composed of two bilateral nephrons that possess a conserved order and arrangement of segment populations similar to other vertebrate nephrons (Wingert and Davidson, 2008). These domains include two proximal and two distal tubule segments: the proximal convoluted tubule (PCT), proximal straight tubule (PST), distal early (DE), and distal late (DL) (Wingert et al., 2007; Wingert and Davidson, 2011). Additionally, genetic studies in zebrafish are readily performed using reverse approaches like genome editing, knockdown or various overexpression techniques, as well as forward approaches like chemical genetics. Recently, we reported the results from a novel small molecule screen using the zebrafish pronephros as a segmentation model, which included the discovery that modulators of peroxisome proliferator-activated receptor (PPAR) signaling altered nephron segmentation (Poureetezadi et al., 2016). Until the present study, however, the functions of discrete PPAR signaling components during nephrogenesis have remained unknown.
PPAR family member peroxisome proliferator-activated receptor γ coactivator 1-alpha (Ppargc1a in zebrafish, PGC-1α in mammals) was discovered as a transcriptional coactivator for several nuclear hormone receptors such as PPAR alpha and gamma (PPARα, PPARγ), histone acetyltransferase steroid receptor coactivator 1 (SRC-1), and thyroid hormone receptor (Puigserver et al., 1998; Puigserver et al., 1999; Wu et al., 1999). PGC-1α serves diverse functions in various contexts not only as a transcriptional coactivator, but also by interactions with chromatin remodeling factors and RNA processing complexes (Knutti and Kralli, 2001; Puigserver and Spiegelman, 2003). PGC-1α is well known to regulate mitochondrial biogenesis and cellular metabolism (Lynch et al., 2018). Additionally, PGC-1α mediates the hepatocyte glucogenesis response to fasting (Herzig et al., 2001; Yoon et al., 2001), cardiac muscle and other slow-twitch muscle development (Lin et al., 2002; Russell et al., 2004), regulates angiogenesis (Arany et al., 2008; Patten et al., 2012; Saint-Geniez et al., 2013) as well as intestinal and skeletal stem cell fate during aging (D'Errico et al., 2011; Yu et al., 2018).
There has been an increasing appreciation for the roles of PGC-1α in adult renal physiology and disease, related in part to high metabolic demands of the kidney and the fact that it is the second most mitochondrial abundant organ (Pagliarini et al., 2008). PGC-1α is expressed in both the adult human kidney and the adult mouse kidney, specifically in the cortex and outer medulla (Tran et al., 2011; Fagerberg et al., 2014; Tran et al., 2016; Casemayou et al., 2017; Han et al., 2017). In experimental murine models of acute and chronic kidney disease, PGC-1α activity has been shown to mediate renoprotection in tubular cells, and deleterious outcomes have been associated with lowered or absent PGC-1α levels in various kidney injury models (Lynch et al., 2018). For example, in acute damage settings, PGC-1α expression is decreased and correlates with elevated fibrosis, whereas damage progression is attenuated when PGC-1α expression is induced (Tran et al., 2011; Tran et al., 2016; Han et al., 2017). Interestingly, ppargc1a/PGC-1α expression has also been annotated in many tissues of developing zebrafish and mice, including the kidney, but their roles in organogenesis events at these locations have not yet been fully ascertained (Bertrand et al., 2007; Thisse and Thisse, 2008; Diez-Roux et al., 2011; Finger et al., 2017). Most pertinent to the current study, the purpose(s) for the expression of ppargc1a transcripts in nascent nephrons has not been explored up until this point.
Here, we report the discovery that ppargc1a has essential roles during nephron segmentation in the zebrafish embryonic kidney. Our studies reveal that the spatiotemporal localization of ppargc1a transcripts in the developing intermediate mesoderm is highly dynamic, where expression throughout the renal progenitors becomes progressively localized to subdomains of the distal nephron segment precursors. Through loss-of-function studies, we show that ppargc1a is necessary for proper formation of two nephron segments, the DL and PST. Furthermore, our genetic studies demonstrate that ppargc1a influences the regionalized expression domains of two essential transcription factors, T-box 2b (tbx2b) and SIM bHLH transcription factor 1a (sim1a), which specify the DL and PST segments, respectively. We discovered that the PST segment boundary is established by an antagonistic relationship between ppargc1a and sim1a. Further, our data reveal that this opposing interaction constitutes a fascinating layer of redundancy with respect to other events that orchestrate nephron segmentation. Taken together, these studies divulge novel mechanisms that define nephron segment boundaries in the embryonic renal mesoderm. Our findings have implications for understanding the basis of nephrogenesis in humans during normal development and congenital disorders affecting renal ontogeny as well.
Results
Bioactive small molecule chemical genetic screen reveals that alteration of PPAR signaling leads to changes in embryonic nephron segmentation
Chemical genetic screening is an efficient method used to employ the strengths of the zebrafish as a model organism to study a wide range of biological processes (North et al., 2007; Garnaas et al., 2012; Nissim et al., 2014; Poureetezadi et al., 2014). By applying different compounds to embryonic zebrafish, one is able to identify novel regulators in a high-throughput manner (Poureetezadi and Wingert, 2016). In a chemical genetic screen of known bioactive compounds, we identified novel regulators of zebrafish pronephros segmentation using a riboprobe cocktail to survey alternating tubule populations (Poureetezadi et al., 2016). One class of identified hits was compounds known to alter the activity of PPAR signaling (Figure 1—figure supplement 1A,B). For example, bezafibrate, a PPAR alpha agonist, was found to reduce the length of the PCT and DE tubule segments, suggesting alterations in processes such as the patterning, growth or cell turnover in the developing nephron (Figure 1—figure supplement 1A,B). Treatment with two PPAR gamma antagonists, BADGE and GW-9662, was associated with an increased DE; further, GW-9662 treatment was also scored as leading to a PCT segment increase (Figure 1—figure supplement 1A,B). These results similarly suggested that alterations in PPAR signaling could modulate nephron segmentation.
To further explore the PPAR pathway result, we collected wild-type (WT) zebrafish embryos and then treated them with dimethyl sulfoxide (DMSO) vehicle control or 150 μM bezafibrate/DMSO from the 5 hpf stage (approximately 50% epiboly) until the 28 somite stage (ss) when the nephron is fully segmented. After removing the drug, embryos were fixed and whole mount in situ hybridization (WISH) was performed to specifically assess formation of each individual nephron tubule segment. For this, we utilized riboprobes to detect transcripts encoding: slc20a1a, to mark the PCT; trpm7, to mark the PST; slc12a1, to mark the DE; and slc12a3, to mark the DL (Wingert et al., 2007). Embryos incubated with bezafibrate displayed a significantly increased length of the PST segment and a reduced DL segment compared to WT controls (Figure 1A,B). In contrast, there were no significant changes in the length of either the PCT or DE segments (Figure 1—figure supplement 1C). This set of phenotypes was present in the majority of embryos (Figure 1—figure supplement 1D). These findings indicated that emergence of the PST and DL segment populations can be modulated by changes in PPAR signaling, and suggested that some component(s) of the PPAR network might normally serve as renal regulators during nephrogenesis.
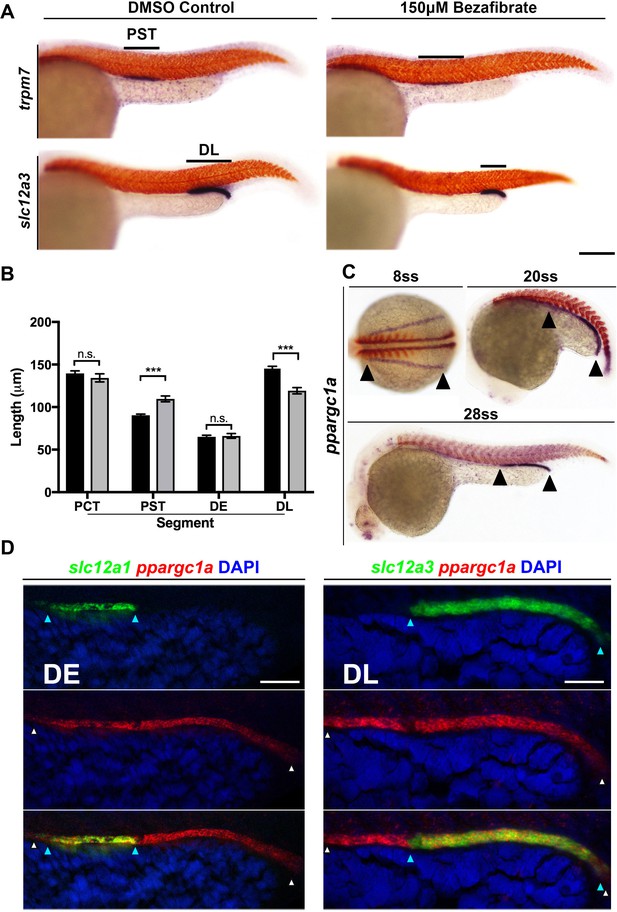
PPAR agonist bezafibrate alters zebrafish pronephros segmentation, and the PPAR coactivator ppargc1a exhibits a dynamic expression pattern in renal progenitors.
(A) Double WISH at the 28 ss for the PST segment marker trpm7 (top), and the DL segment marker slc12a3 (bottom), with smyhc (red) to mark somites in DMSO control (left) and PPAR agonist, 150 μM Bezafibrate-treated (right) samples confirmed the initial hit from the chemical screen. Scale bar = 90 μm. (B) Absolute length measurements of the changes to pronephros segment lengths in bezafibrate treated (grey) and control samples (black). (C) Double WISH for ppargc1a (purple) expression at the 8 ss, 20 ss, and 28 ss with somites stained (red) (8 ss = deltaC, 20 ss and 28 ss = smyhc). (D) Double FISH at the 28 ss showing colocalization of ppargc1a (red) with slc12a1 (distal early, left) and slc12a3 (DL, right). Expression boundaries are indicated with blue (DE, left and DL, right) and white (ppargc1a) arrowheads. Scale bars = 35 μm. Data are represented as ±SD, significant by t test comparing the drug treatment to the DMSO vehicle control, n.s. = not significant, *** = p < 0.001.
ppargc1a is dynamically expressed in the developing zebrafish embryonic kidney
Intrigued by these results, we next sought to identify whether the expression of any PPAR signaling components would situate them as possible candidates for involvement in nephron development. We surveyed online expression repositories and published literature and found that transcripts encoding ppargc1a/PGC-1α have been detected in nascent nephrons within the developing zebrafish pronephros and the mouse metanephros (Bertrand et al., 2007; Thisse and Thisse, 2008; Diez-Roux et al., 2011; Ai et al., 2017; Finger et al., 2017). To further investigate this, we performed WISH on WT embryos and assessed the spatiotemporal patterns of ppargc1a expression throughout the stages of zebrafish pronephros ontogeny. ppargc1a transcripts were expressed in a pattern suggesting their presence in the entire populace of the developing intermediate mesoderm at the 8 ss, followed by a caudal restriction at the 20 ss before localizing to the distal segments at the 28 ss (Figure 1C, Figure 1—figure supplement 2). At the 30 hpf time point, weak expression levels of ppargc1a transcripts were detected in the proximal tubule as well (Figure 1—figure supplement 2A).
To confirm that ppargc1a was expressed in renal precursors, we employed double fluorescent WISH (FISH) and confocal imaging in WT embryos. At the 15 ss, ppargc1a transcripts were co-localized in the entire domain of cells that expressed transcripts encoding the intermediate mesoderm marker paired box 2a (pax2a) (Figure 1—figure supplement 2B,C) (Krauss et al., 1991; Püschel et al., 1992). By the 28 ss, ppargc1a transcripts were colocalized only in cells that expressed the distal segment markers slc12a1 and slc12a3, indicating restriction to the DE and DL, respectively (Figure 1D). These data provide strong evidence that renal progenitors, followed by segment precursors and eventually differentiated distal segments, express ppargc1a. Based on the evidence that ppargc1a is dynamically expressed in the developing nephron, we hypothesized that it was involved in segment patterning.
ppargc1a is necessary for proper formation of proximal and distal segment boundaries
To define whether ppargc1a is essential for nephrogenesis, we designed several parallel strategies to perform loss of function studies. The ppargc1a locus is comprised of a series of 12 exons (Figure 2A), and these encode a peptide that shares high sequence similarity, particularly in key functional domains, to mouse and human PGC-1α (Figure 2—figure supplement 1) (Puigserver and Spiegelman, 2003). Four distinct locations of the ppargc1a sequence were targeted for experimental manipulation (Figure 2A, Figure 2—figure supplement 1) in order to disrupt transcriptional processing or translation. First, we obtained a ppargc1a genetic knockout line (ppargc1asa13186), which encodes a T->A substitution located in exon seven that results in a premature STOP codon and eliminates a series of essential peptide domains (Figure 2A) (ZIRC - Eugene, Oregon; Busch-Nentwich et al., 2013). Sequencing confirmed the mutation and we developed a genotyping assay, which utilizes PCR amplification followed by NdeI restriction fragment length polymorphism digest analysis where the enzyme can cut the WT but not the mutant allele (Figure 2—figure supplement 2D). Second, we developed genetic models of ppargc1a deficiency using morpholinos (MOs). These included a translation blocking MO (MO1) (Hanai et al., 2007; Bertrand et al., 2007) and two splice blocking MOs (SB MO1, SB MO2) that we designed and subsequently validated through microinjection and RT-PCR studies in WT embryos (Figure 2A, Figure 2—figure supplements 3 and 4).
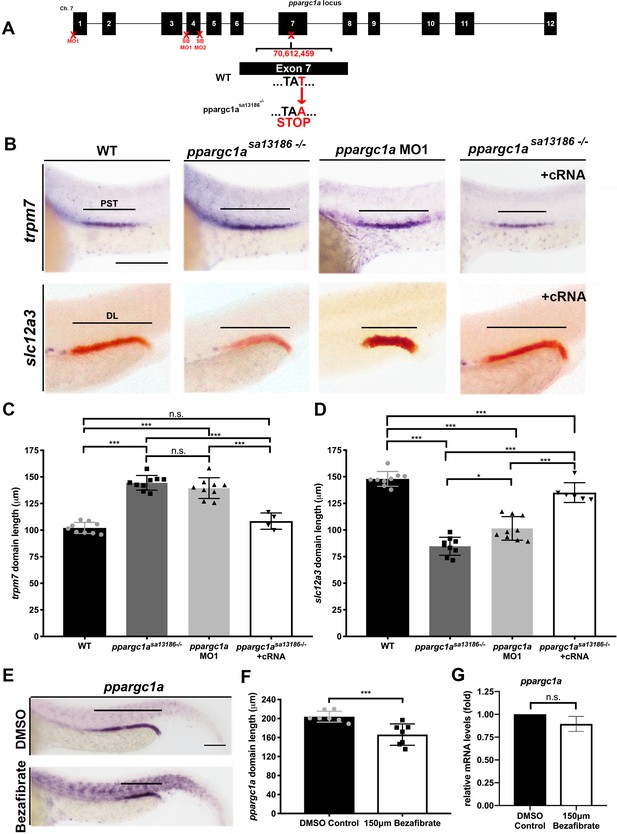
Loss-of-function studies show ppargc1a is necessary for proper PST and DL formation.
(A) Exon map of zebrafish ppargc1a and the target sites (X) for morpholinos (MO) and the location of the ppargc1asa13186 mutant allele. (B) WISH images of 28 ss ppargc1asa13186 WT siblings (WT), ppargc1asa13186-/-, ppargc1a MO1 injected, and ppargc1asa13186-/- + ppargc1a cRNA illustrating the changes in PST (trpm7-purple, top) and DL (slc12a3-red, bottom) formation in the ppargc1asa13186-/- and ppargc1a morphants, and the subsequent rescue when ppargc1a cRNA was added to the ppargc1asa13186-/-. Scale bars = 100 μm. Absolute length measurements of the PST (C), and DL (D) segments. (E) ppargc1a expression in DMSO control (top) and 150 μM bezafibrate-treated (bottom) zebrafish at the 28 ss following vehicle or vehicle/drug addition at the 5 hpf stage. Scale bars = 65 μm. (F) Absolute length measurements of the ppargc1a expression domain at 28 ss in DMSO control and bezafibrate-treated embryos from panel E. (G) qRT-PCR results showing ppargc1a RNA expression levels in bezafibrate-treated samples compared to DMSO controls. Data are represented as ±SD, significant by t test, n.s. = not significant, ** = p < 0.01, *** = p < 0.001.
The ppargc1a mutant and knockdown reagents were then utilized to evaluate nephron segment development. Embryos were collected from pairwise matings of ppargc1asa13186+/- adult carriers and fixed at the 28 ss. For the knockdowns, WT embryos were microinjected at the one-cell stage with either MO1, SB MO1 or SB MO2 and similarly fixed at the 28 ss. WISH was completed on the ppargc1a-deficient embryo cohorts using segment-specific riboprobes to assess formation of the PCT, PST, DE and DL. Both ppargc1asa13186-/-mutants and knockdown embryos had a significantly expanded PST segment and a significantly decreased DL segment (Figure 2B–D, Figure 2—figure supplements 3 and 4). In contrast, there were no significant changes in PCT or DE segment formation (Figure 2—figure supplement 2A–C).
To further verify the specificity of the phenotypes, we performed rescue studies in ppargc1a mutant embryos. Full-length ppargc1a capped mRNA (cRNA) was synthesized in vitro, purified and microinjected at the one-cell stage into clutches obtained from pairwise matings of ppargc1asa13186+/- adults. Segmentation was assessed by WISH at the 28 ss to evaluate the development of the PST and DL segments. ppargc1a cRNA was sufficient to rescue PST and DL segment length in ppargc1asa13186-/- mutant embryos (Figure 2B–D). This result confirmed that the nephron phenotypes observed in this mutant model are caused by specific disruption of ppargc1a and exclude the possibility of other underlying genetic alterations.
Next, we explored whether there was a connection between the outcomes of bezafibrate treatment and ppargc1a loss of function during nephron segmentation. Previous publications have reported that PPAR agonists, including bezafibrate, can cause an increase or decrease of ppargc1a/PGC1a expression in cells and tissues in a context-dependent manner (Pardo et al., 2011; Liao et al., 2010; Sanoudou et al., 2010; Goto et al., 2017; Wang and Moraes, 2011). Since bezafibrate treatment and ppargc1a deficiency caused matching segment phenotypes, we hypothesized that bezafibrate decreased ppargc1a expression in renal progenitors. To test this, WT embryos were treated with either vehicle control or 150 μM bezafibrate/DMSO beginning at different developmental times (4 hpf, 5 hpf, 6 hpf, 8 hpf, 9 hpf, 10 hpf, 5 ss and 10 ss), incubated until the 28 ss, and then WISH was performed. Compared to WT controls, bezafibrate treatment resulted in a significant decrease in the expression domain of ppargc1a in the pronephros when the drug was added between 4 and 9 hpf (Figure 2E,F, Figure 2—figure supplement 5A,B). Next, we explored whether the ppargc1a expression domain was altered at the 15 ss when it is normally expressed in the pax2a+ renal progenitor domain. Embryos were treated with DMSO vehicle or 150 μM bezafibrate/DMSO at the 5 hpf stage, and then fixed at 15 ss for WISH. Interestingly, the ppargc1a expression domain in renal progenitors at 15 ss was not altered by the bezafibrate treatment (Figure 2—figure supplement 5C). We also performed qRT-PCR on pools of 28 ss embryos treated with DMSO vehicle or 150 μM bezafibrate/DMSO at the 5 hpf stage to quantify ppargc1a expression levels. There was no significant difference in total ppargc1a mRNA levels between WT and bezafibrate-treated embryos (Figure 2G). Furthermore, we found that ppargc1a RNA overexpression was not sufficient to rescue DL development at the 28 ss in embryos treated with bezafibrate at the 5 hpf stage (Figure 2—figure supplement 5D,E). Additionally, we assessed the overall morphology as well as pronephros formation in 28 ss embryos treated with bezafibrate or vehicle control at the 5 hpf, as well as wild-type and ppargc1a-deficient embryos (Figure 2—figure supplement 6). Analysis of body length and pronephros length, the latter through WISH to detect expression of the pan-tubule and duct marker cdh17, showed no statistically significant differences between the groups (Figure 2—figure supplement 6). Taken together, these results are consistent with the notion that the ppargc1a expression domain is reduced in bezafibrate-treated embryos because the DL is reduced, and not specifically due to the loss of ppargc1a activity.
Loss of ppargc1a does not change cellular turnover in the developing nephrons
Segmentation of the renal progenitors in the intermediate mesoderm occurs from the early somitogenesis stages through to the 28 ss based on the detection of molecularly distinct regions that emerge and then show dynamic alterations over this developmental time period, all while the renal progenitors are also undergoing a mesenchymal to epithelial transition (Wingert et al., 2007; Wingert and Davidson, 2011; Li et al., 2014; Gerlach and Wingert, 2014; McKee et al., 2014; Kroeger and Wingert, 2014; Cheng and Wingert, 2015; Marra and Wingert, 2016; Drummond et al., 2017; Poureetezadi et al., 2016). The proliferation and caudal migration of renal precursors has also been reported to impact pronephros segment size (Naylor et al., 2016). To this end, we wanted to determine when the loss of ppargc1a first presented significant changes to the emerging segment domains, and to address if these changes were coincident with alterations in cellular dynamics in the nephron field.
A series of WISH studies were performed with PST and DL markers to compare these emerging segment populations in WT controls and ppargc1a-deficient embryos (Figure 3). We found that the earliest time point of divergence between WT and ppargc1a-deficient embryos occurred at the 20 ss, when there was a distinction in the expression domain of both the PST marker trpm7 and DL marker slc12a3 (Figure 3A–D, Figure 3—figure supplement 1). ppargc1a-deficient embryos displayed a significant increase in the emerging PST length and a significant decrease in the emerging DL length (Figure 3A–D). These changes correlate with the result that ppargc1a mutants and morphants exhibit a longer PST and shortened DL when segmentation is completed (Figure 2).
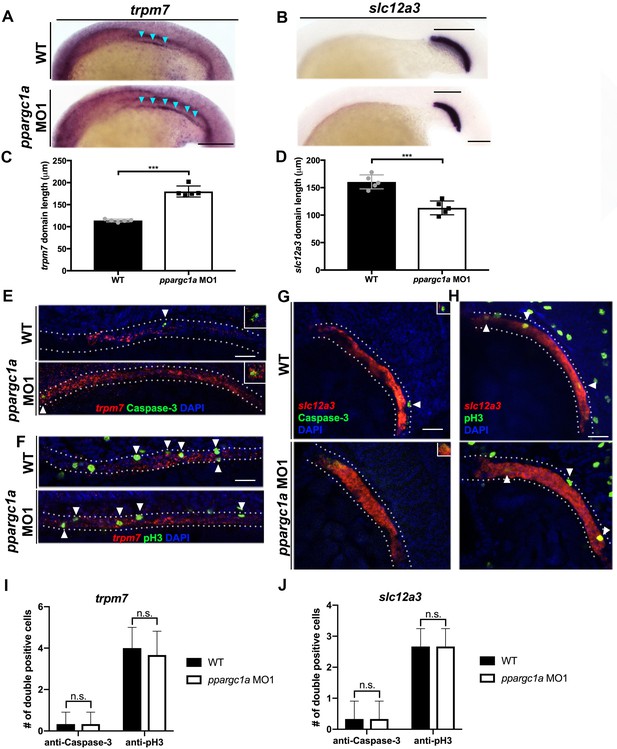
Loss of ppargc1a does result in segment changes at the 20 ss but no change in cellular turnover is observed.
(A) WISH at the 20 ss for trpm7 (left) and (B) slc12a3 (right) in WT (top) and ppargc1a MO (bottom). Blue arrowheads indicate trpm7 expression in the developing pronephros. Scale bar = 100 μm. The representative graphs showing absolute length measurements of trpm7 (C) and slc12a3 (D). (E) FISH/IHC for trpm7 (red) and anti-Caspase-3 (green) with DAPI (blue) in WT (top) and ppargc1a MO1 (bottom). (F) FISH/IHC for trpm7 (red) and anti-phospho-Histone H3 (green) in WT (top) and ppargc1a MO1 (bottom). (G) FISH/IHC for slc12a3 (red) and anti-Caspase-3 (green) with DAPI (blue) in WT (top) and ppargc1a MO1 (bottom). (H) FISH/IHC for slc12a3 (red) and anti-phospho-Histone H3 (green) with DAPI (blue) in WT (top) and ppargc1a MO1 (bottom). (I) The number of trpm7/Caspase-3 or trpm7/pH3 double-positive cells is depicted. (J) The number of slc12a3/Caspase-3 or slc12a3/pH3 double positive cells is depicted. (WT quantifications = black bars, ppargc1a-deficient quantifications = white bars.) Data are represented as ±SD, significant by t test, n.s. = not significant, *** = p < 0.001.
At this pivotal 20 ss time point, we then sought to identify whether either of these changes were associated with regional fluctuations in cell birth or death. To assess this, we combined FISH with whole mount immunohistochemistry and confocal imaging to assess the PST and DL. WT control and ppargc1a-deficient embryos were fixed at the 20 ss and nephron cells were detected based on trpm7 (PST) or slc12a3 (DL) transcripts in combination with either anti-Caspase-3 to detect cell death or anti-phospho-Histone H3 (anti-pH3) to label proliferating cells (Kroeger et al., 2017). The results showed that there was no significant difference between WT and ppargc1a-deficient embryos in the number of trpm7+/anti-Caspase-3+ cells (Figure 3E,I, Figure 3—figure supplement 2) or slc12a3+/anti-Caspase-3+ cells (Figure 3G,J, Figure 3—figure supplement 4). Quantification of trpm7+/pH3+ cell number (Figure 3F,I, Figure 3—figure supplement 3) and slc12a3+/pH3+ cell number (Figure 3H,J, Figure 3—figure supplement 5) also showed that there was no statistically significant difference between WT and ppargc1a-deficient groups. The results from these experiments suggest that there are no significant changes in cellular turnover driving the PST and DL segment boundary changes that occur in ppargc1a-deficient embryos.
ppargc1a promotes DL segment formation by positively regulating the expression domain of the tbx2b transcription factor in nephron precursors
To gain insight into how ppargc1a influences nephron segmentation, we next explored its relationship with the T-box transcription factor tbx2b, which was recently shown to be essential for DL formation (Drummond et al., 2017). Transcripts encoding tbx2b are highly expressed in the distal regions of the zebrafish pronephros, and loss of tbx2b results in a significantly decreased DL segment size (Drummond et al., 2017). To test the relationship between ppargc1a and tbx2b, WISH was performed on ppargc1a deficient embryos to assess tbx2b expression. Compared to WT controls, the tbx2b expression domain in the nephron was significantly reduced in ppargc1asa13186-/- mutants (Figure 4A,B) as well as ppargc1a morphants (data not shown). These results led us to hypothesize that loss of tbx2b expression underlies the decreased DL segment domain when Ppargc1a activity is compromised. When the reciprocal experiment was performed in tbx2b-deficient embryos (Drummond et al., 2017; Gross and Dowling, 2005), we detected no change in ppargc1a expression (Figure 4C,D), consistent with the notion that ppargc1a is upstream of tbx2b.
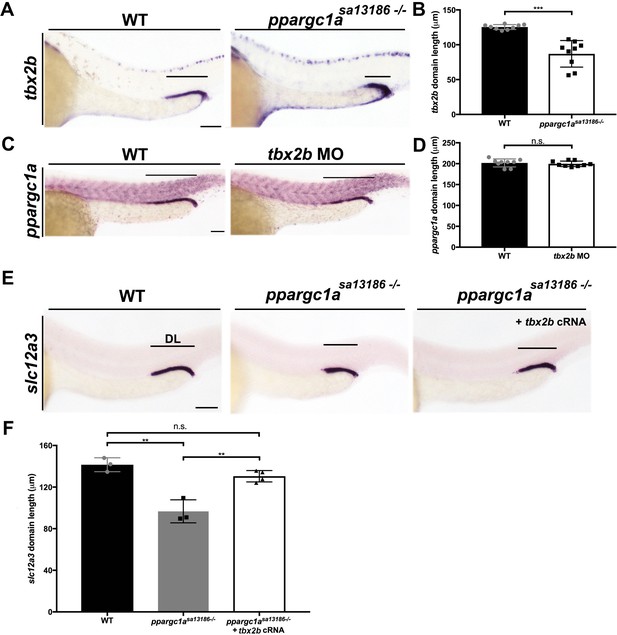
ppargc1a acts upstream of tbx2b to form the DL segment.
(A) WISH for tbx2b (purple) expression in 28 ss WT and ppargc1asa13186-/-zebrafish. Scale bars = 75 μm. (B) Absolute length measurements of tbx2b mRNA expression domains in WT and ppargc1asa13186-/- zebrafish. (C) ppargc1a expression in WT and tbx2b MO injected 28 ss zebrafish. Scale bars = 65 μm. (D) Absolute length measurements of ppargc1a expression domain in WT and tbx2b MO injected zebrafish. (E) WISH at 28 ss for slc12a3 (purple) in WT, ppargc1asa13186-/-, and ppargc1asa13186-/- injected with tbx2b cRNA. Scale bars = 75 μm. (F) Absolute length measurements of slc12a3 mRNA expression domains in WT, ppargc1asa13186-/-, and ppargc1asa13186-/- + tbx2b cRNA. Data are represented as ±SD, significant by t test, n.s. = not significant, ** = p < 0.01, and *** = p < 0.001.
To test this further, we examined whether overexpression of tbx2b was sufficient to rescue DL development in ppargc1a mutants. tbx2b cRNA was injected at the one-cell stage into clutches obtained from matings of ppargc1asa13186+/- adults, and segmentation was assessed at the 28 ss by WISH using our DL-specific riboprobe followed by genotype analysis (Figure 4E). While ppargc1asa13186-/- mutants displayed the hallmark short DL segment, there was no significant difference in DL length between WT controls and ppargc1asa13186-/- mutants that received tbx2b cRNA, indicating that tbx2b provision had rescued DL segment development (Figure 4E,F). Taken together, these results indicate that Ppargc1a regulates tbx2b, either directly or indirectly, to control formation of the DL segment.
ppargc1a regulates PST boundary formation through a reciprocally antagonistic relationship with the sim1a transcription factor
During zebrafish embryonic nephron segmentation, sim1a is necessary and sufficient for formation of the PST segment as well as the Corpuscle of Stannius (CS), the latter being an endocrine gland in teleost fish which arises from the intermediate mesoderm, where CS precursors are intermingled with distal segment precursors (Cheng et al., 2015). Thus, we sought to delineate the relationship between ppargc1a and sim1a. To do this, we examined ppargc1a expression in sim1a-deficient embryos in which transcript splicing is abrogated through morpholino knockdown (Figure 5—figure supplement 1) (Löhr et al., 2009; Cheng and Wingert, 2015). Interestingly, we found that the domain of ppargc1a expression in renal progenitors was significantly increased in length in the sim1a-deficient embryos compared to WT controls (Figure 5A,D). This result suggested sim1a was possibly upstream of ppargc1a.
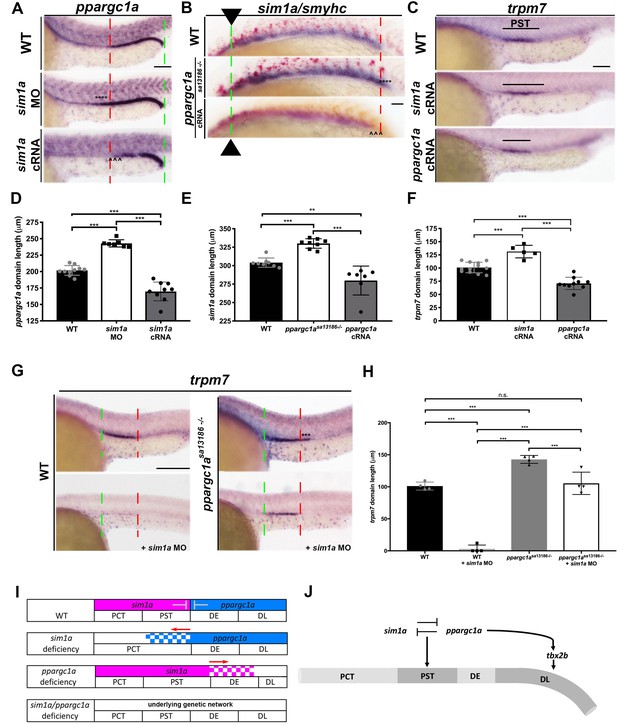
ppargc1a and sim1a have a reciprocal antagonistic relationship that is necessary to negotiate the PST segment boundary.
(A) WISH for ppargc1a (purple) expression at 28 ss in WT, sim1a MO, and sim1a cRNA injected zebrafish. The green and red dashed lines indicate the beginning and the end of WT expression, respectively, the expansion of the ppargc1a domain in sim1a MO is denoted with asterisks, and the reduction in the ppargc1a domain in sim1a cRNA injected is denoted with arrowheads. Scale bars = 60 μm. (B) WISH for sim1a (purple) and smyhc (red) in 20 ss WT, ppargc1asa13186-/-, and ppargc1a cRNA injected zebrafish. Arrows and the dashed green line represent the beginning of the sim1a domain expression, the red dashed line indicate the end of the WT sim1a expression domain to illustrate the expansion of the segment boundary in ppargc1asa13186-/- is denoted with asterisks, and the reduction of the sim1a domain in ppargc1a cRNA injected is denoted with arrowheads. Scale bar = 40 μm. (C) WISH for trpm7 at 28 ss in WT, sim1a cRNA, and ppargc1a cRNA injected zebrafish. Scale bar = 50 μm (D) Absolute length measurements of the ppargc1a domain in 28 ss in WT, sim1a MO injected, and sim1a cRNA injected zebrafish. (E) Absolute length measurements of the 20 ss sim1a domain in WT, ppargc1asa13186-/-, and ppargc1a cRNA injected zebrafish. (F) Absolute length measurements of the trpm7 domain in the 28 ss WT, sim1a cRNA injected, and ppargc1a cRNA injected zebrafish. (G) WISH for trpm7 (PST segment) in WT, WT + sim1 a MO, ppargc1asa13186-/-, and ppargc1asa13186-/- + sim1a MO zebrafish. The green and red dashed lines indicate the beginning and end of WT trpm7 expression domains respectively. Asterisks represent expanded expression domain. Scale bars = 100 μm. (H) Absolute length measurements for the trpm7 domain in WT, sim1a MO injected, ppargc1asa13186-/-, and ppargc1asa13186-/- sim1a MO injected zebrafish. (I) Expression summary table depicting the segment boundaries and expression domains of ppargc1a and sim1a in WT, sim1a-deficient, ppargc1a-deficient, and double-deficient zebrafish. (J) Genetic model illustrating the relationships supported in this study that ppargc1a works upstream of tbx2b to form the DL and has a cross-repressive relationship with sim1a to properly form the segment boundary of the PST. Data are represented as ±SD, significant by t test, n.s. = not significant, *** = p < 0.001.
Since ppargc1asa13186-/- mutants evince an increased PST segment (Figure 2B,C) in addition to an increased CS size (Figure 5—figure supplement 2A,B), we next examined whether Ppargc1a deficiency was associated with changes in sim1a expression in renal precursors. WISH was performed to investigate the pattern of sim1a expression in ppargc1asa13186-/- mutant embryos. This analysis revealed that ppargc1asa13186-/- mutant embryos had an increased sim1a domain at the 20 ss, a time point that coincides with sim1a expression in the PCT and PST segments, as well as at the 28 ss, which coincides with expression of sim1a in the CS anlage (Figure 5B,E, Figure 5—figure supplement 2C,D). Taken together, these results suggested that there were reciprocal antagonistic interactions between these two factors, which act to delineate segmental domains.
To further explore cross-repressive interactions, we next performed RNA overexpression studies with sim1a and ppargc1a in WT embryos. Interestingly, overexpression of sim1a cRNA led to a statistically significant decrease in the domain of ppargc1a transcript expression within the pronephros at the 28 ss (Figure 5A,D), as well as an increase in PST segment length (Figure 5C,F), the latter as previously reported (Cheng and Wingert, 2015). sim1a cRNA overexpression also had no effect on DL segment development (Figure 5—figure supplement 3), consistent with intact ppargc1a expression in the distal pronephros region (Figure 5A). Overexpression of ppargc1a cRNA caused a statistically significant decrease in the domain of sim1a transcript expression within the pronephros at the 20 ss (Figure 5B,E) as well as the CS anlage at the 28 ss (Figure 5—figure supplement 2C,D). ppargc1a cRNA overexpression also reduced the PST segment length at the 28 ss (Figure 5C,F). These results support the conclusion that sim1a and ppargc1a have repressive effects on each other in the context of renal progenitor development in the zebrafish.
In light of the observation that sim1a cRNA overexpression is sufficient to expand the PST segment (Figure 5C,E) (Cheng and Wingert, 2015), we hypothesized that the expansion of the sim1a expression in ppargc1a mutants was responsible for the enlarged PST phenotype. To interrogate this and gain further insight about the relationship between ppargc1a and sim1a, we tested the outcome of sim1a knockdown in ppargc1a mutants compared to WT embryos. sim1a MO was injected at the one-cell stage into ppargc1asa13186 heterozygous in-crosses, and nephron segmentation was assessed in these and uninjected controls by WISH at the 28 ss to study PST development. Consistent with our previous results, sim1a deficiency abrogated the PST in WT embryos, and there was a larger PST in the ppargc1asa13186-/- mutants (Figure 5G,H). By comparison, ppargc1a/sim1a doubly deficient embryos formed a PST segment of normal length (Figure 5G,H). Taken together, these results suggest that sim1a promotes PST fate by acting to control the expression domain of ppargc1a in proximal renal progenitors, which establishes the proper boundary of the PST segments (Figure 5I). Additionally, these results show that ppargc1a serves an antagonistic role to sim1a, restricting the spatial domain of sim1a expression from distal renal progenitors to define the PST segment boundary (Figure 5I).
Discussion
Elucidating the genetic regulators that direct cell fate decisions during nephron ontogeny is paramount to understanding how molecular changes cause renal organogenesis defects, and can be applied to advance regenerative medicine approaches for the treatment of kidney disease. Evidence from our chemical genetic screen led us to identify that PPAR signaling was a possible candidate for regulating the nephron segment lineages during embryogenesis. Here, we determined that ppargc1a expression in renal progenitors is essential to mitigate segment fate choices that establish segment identities in the pronephros.
Specifically, we discovered that ppargc1a is necessary for proper formation of the PST and DL segment boundaries. We also identified the timing associated with these segmentation changes and observed cellular turnover analogous to WT embryos, suggesting that segment phenotypes in ppargc1a mutants are not related to alterations in cell proliferation or cell death. We ascertained that there are two critical genetic pathways that ppargc1a regulates to control PST and DL segment size. Through a series of genetic studies, we determined that ppargc1a acts upstream of tbx2b to promote DL formation and we uncovered an intriguing, reciprocally antagonistic relationship between sim1a and ppargc1a that operates to properly form the PST segment (Figure 5J), as well as the CS anlage (Figure 5—figure supplement 2). Discovery of the opposing activities of ppargc1a and sim1a in the present work highlights for the first time how the precise dimension of the PST is defined by reciprocal antagonism during segmentation of the kidney nephron unit. While this interplay is essential for segment fate choice, a fascinating aspect revealed by our studies is that there is an underlying genetic network that enables PST segment development to transpire normally in the absence of both of these powerful opposing transcription factors. Future efforts to identify these other genetic components are needed to decipher the mechanisms of PST formation, where there have been few advances in understanding the patterning of this segment despite progress in understanding ciliated cell fate choice in this pronephros region (Marra et al., 2016). With respect to DL segment ontogeny, additional work is also needed to ascertain how ppargc1a relates to known DL regulators such as the transcription factors mecom, tbx2a, and emx1 along with prostaglandin signaling (Li et al., 2014; Drummond et al., 2017; Poureetezadi et al., 2016; Morales et al., 2018).
Previous studies have established that PGC-1α can exert its regulatory effects on the transcription of target genes in cell-specific contexts through its interactions with a variety of nuclear receptors. Transcriptional regulation by PGC-1α is known to play key roles in diverse biological processes, from mitochondrial biogenesis to metabolic activities, in which PGC-1α coordinates dynamic responses to physiological demands (Lin et al., 2005). Additionally, PGC-1α coordinates transcriptional activities during cell differentiation, such as in erythrocyte maturation, where it has unique and shared nuclear targets with its family member PGC-1α that impact globin gene regulation (Cui et al., 2014). Transcriptional profiling of renal progenitors in ppargc1a mutants, assessment of chromatin state with techniques such as the assay for transposase accessible chromatin using sequencing (ATAC-seq) and chromatin immunoprecipitation with sequencing (ChIP-Seq), can delineate the possible direct and indirect targets of Ppargc1a. As coactivators typically function in multiprotein complexes, identification of both the relevant nuclear receptor targets and other binding partners of Ppargc1a in renal progenitors will be crucial to gaining additional insight on the emergence of nephron segment populations and the establishment of boundaries between adjacent segments. The precedence that members of the PGC-1 family can have redundant activities suggests that future investigations should also explore this possibility for Ppargc1a during nephron segmentation (Cui et al., 2014). Such redundancy might explain the absence of congenital kidney defects in the PGC-1α murine knockout, and may necessitate combined deficiency studies to delineate the roles of PGC-1 members in mammalian renal development.
Further explorations of the mechanisms by which Ppargc1a regulates renal progenitors should also consider possibilities in addition to transcriptional control because of the precedence that PGC-1α possesses a number of molecular activities depending on the context. PGC-1α is highly versatile, whereby it can interact with a range of molecules other than transcription factors. PGC-1α can recruit histone acetyl transferase containing coactivator proteins and can also interact with RNA processing complexes (Knutti and Kralli, 2001; Puigserver and Spiegelman, 2003). Proteomics approaches in renal progenitors may thus identify Ppargc1a binding partners with such activities and if so, will highlight other directions by which to elucidate the roles of Ppargc1a during nephron segmentation.
While functions for Ppargc1a in vertebrate renal development have not been reported until the present study, interesting roles of PGC-1α have been identified during the response to kidney damage in mammals. PGC-1α serves a renoprotective function in the murine kidney. PGC-1α is downregulated after ischemic and sepsis induced acute kidney injury, and normal renal function is restored with overexpression of PGC-1α (Tran et al., 2011; Tran et al., 2016). PGC-1α is also reduced in three different modes of chronic kidney disease: toxic, obstructive, and genetic (Han et al., 2017). Hes1 represses ppargc1a during Notch-induced renal fibrosis; however, induced overexpression of ppargc1a can ameliorate this process (Han et al., 2017). In light of the potent influence that Ppargc1a exacts on renal progenitors during embryonic kidney development, it is intriguing to speculate whether modulation of Ppargc1a could be utilized further to stimulate regenerative therapies. In addition, Ppargc1a is a prime candidate for being involved in the capacity of the zebrafish adult to regenerate nephrons and undergo neonephrogenesis, where the prediction would be that Ppargc1a is similarly essential in nephron precursors to mitigate emergence of the PST and DL segment fates (Diep et al., 2011; McCampbell and Wingert, 2014; McCampbell et al., 2014; McCampbell et al., 2015; Drummond and Wingert, 2016).
While there have been ongoing advancements in our understanding of nephron patterning during development and the pathways that facilitate nephron epithelial regeneration following damage, many gaps in knowledge still remain. The continued identification of the genetic networks that regulate renal progenitors in these contexts has far-reaching implications (Chambers and Wingert, 2016). Our new insights into nephron segmentation have divulged novel mechanisms that define nephron segment boundaries in the embryonic renal mesoderm. Taken together, our data show for the first time that ppargc1a is required for pivotal renal progenitor fate decisions that establish nephron segment pattern during kidney development. Given the fundamental conservation of segment pattern across vertebrate nephrons, we speculate that these newly discovered roles of Ppargc1a will provide useful clues about PGC-1α functions in mammalian kidney development. As alterations in Ppargc1a activity have potent effects on nephron segment fate, our results suggest that Ppargc1a/PGC-1α may be an important molecular target for medical applications or engineering approaches involving the directed differentiation of pluripotent stem cells to fashion kidney organoids (Chambers et al., 2016). Here, we have only begun to appreciate the importance of ppargc1a in kidney development, focusing on the role it plays in segment boundary formation.
Materials and methods
Reagent type (species) or resource | Designation | Source or reference | Identifiers | Additional information |
---|---|---|---|---|
Antibody | anti-Caspase-3 (rabbit) | BD Biosciences | 559565 | dilution 1:100 |
Antibody | phospho-Histone H3 (Ser10) (rabbit) | Millipore | 06–570 | dilution 1:200 |
Antibody | Alexa Fluor anti-rabbit secondary (goat) | Invitrogen | A11037 | dilution 1:500 |
Chemical compound, drug | dimethyl sulfoxide (DMSO) | American Bioanalytical | AB03091-00100 | |
Chemical compound, drug | bezafibrate | Enzo Life Sciences | BML-GR211-0001 | |
Genetic reagent (Danio rerio) | ppargc1asa13186 zebrafish line | Zebrafish International Resource Center (ZIRC) | ZMP:sa13186 | Zebrafish Mutation Project allele sa13186 |
Commercial assay or kit | PCR purification kit | Qiagen | 28106 | |
Commercial assay or kit | NdeI restriction endonuclease enzyme | New England BioLabs | R0111S | |
Commercial assay or kit | TRIzol Reagent | Invitrogen | 15596018 | |
Commercial assay or kit | qScript cDNA SuperMix | QuantaBio | VWR 101414–106 | |
Commercial assay or kit | PerfeCTa SYBR Green SuperMix with ROX | QuantaBio | VWR 101414–160 | |
Chemical compound, drug | mMESSAGE mMACHINE SP6 Transcription kit | Ambion | AM1340 | |
Other | custom antisense morpholino oligonucleotide | Gene Tools, LLC | ppargc1a ATG MO1 (ZFIN: MO1-ppargc1a) | 5’–CCTGATTACACCTGTCCCACGCCAT–3’ |
Other | custom antisense morpholino oligonucleotide | Gene Tools, LLC | ppargc1a SB MO1 | 5’–GGAGCTTCTTCAGCTACAAACAGAG–3’ |
Other | custom antisense morpholino oligonucleotide | Gene Tools, LLC | ppargc1a SB MO2 | 5’–GGTGAGCAGCTACCTTGGCAACAGC–3’ |
Other | custom antisense morpholino oligonucleotide | Gene Tools, LLC | tbx2b MO | 5’–CCTGTAAAAACTGGATCTCTCATCGG–3’ |
Other | custom antisense morpholino oligonucleotide | Gene Tools, LLC | sim1a MO | 5’–TGTGATTGTGTACCTGAAGCAGATG–3’ |
Software, algorithm | Nikon Elements imaging software | Nikon | ||
Software, algorithm | Graphpad Prism 8 | GraphPad Prism (https://www.graphpad.com/scientific-software/prism/) | ||
Software, algorithm | ImageJ | ImageJ (https://imagej.nih.gov/ij/) |
Zebrafish husbandry and ethics statement
Request a detailed protocolZebrafish were maintained in the Center for Zebrafish Research at the University of Notre Dame. All studies were performed with approval of the University of Notre Dame Institutional Animal Care and Use Committee (IACUC), under protocol numbers 13–021 and 16–025. For experiments with WT zebrafish, we utilized the Tübingen strain. Embryos were raised and staged as described (Kimmel et al., 1995). For all molecular studies, embryos were incubated in E3 medium from fertilization through the desired developmental stage at 28°C, anesthetized with 0.02% tricaine, and then fixed for analysis using 4% paraformaldehyde/1 x phosphate buffered saline (Westerfield, 1993).
Whole mount and fluorescent whole mount in situ hybridization (WISH, FISH)
Request a detailed protocolWISH was performed as previously described (Cheng et al., 2014; Galloway et al., 2008; Lengerke et al., 2011) with antisense RNA probes either digoxigenin-labeled (ppargc1a, slc20a1a, trpm7, slc12a1, slc12a3, sim1a, tbx2b, stc1, cdh17) or fluorescein-labeled (smyhc, slc12a3, -slc12a1, pax2a) by in vitro transcription using IMAGE clone templates as previously described (Wingert et al., 2007; O'Brien et al., 2011; Gerlach and Wingert, 2014; McKee et al., 2014). FISH was performed as previously described (Brend and Holley, 2009; Marra et al., 2017). For all gene expression studies, every analysis was done in triplicate for each genetic model, in a blinded fashion, with sample sizes of at least n = 20 for each replicate. A minimum of 5 representative individuals from each replicate were imaged and quantified, then subjected to statistical analysis.
Immunofluorescence (IF)
Request a detailed protocolWhole mount IF experiments were completed as previously described (Kroeger et al., 2017; Marra et al., 2017). For cell death and proliferation assays, rabbit anti-Caspase-3 diluted 1:100 (BD Biosciences 559565) and rabbit phospho-Histone H3 (Ser10) antibody diluted 1:200 (Millipore 06–570) were used, respectively. Anti-rabbit secondary antibody (Alexa Fluor, Invitrogen) was diluted 1:500.
Chemical treatments
Request a detailed protocolChemical treatments were completed as previously described (Poureetezadi et al., 2014; Poureetezadi et al., 2016). Bezafibrate (Enzo Life Sciences, BML-GR211-0001) was dissolved in 100% DMSO to make 1 M stocks and diluted to the working dosage. For segment analysis, treatments were completed in triplicate with sample sizes of at least 20 embryos per replicate.
Genetic models
Request a detailed protocolThe ppargc1asa13186 line was obtained from ZIRC (Eugene, OR) (Busch-Nentwich et al., 2013). Mutant embryos and heterozygous adults were identified by performing PCR with the following primers flanking the mutation site: forward 5’–GGGCCGGCATGTGGAATGTAAAGACTTAAACATGCCAACCTCCACTACTACGACATCATCGTTGTCTTCCACCCCCCCTTCGTCTTCCTCACTGGCCA GG–3’ and reverse 5’–TCCCACTACCCCGCTATAGAAGGCTTGCTGAGGCTTTCCAAAGTGCTTGTTGAGCTCGTCCCGGATCTCCTGGTCCCTAAGAAGTTTCCTGCCACCAGAA–3’. PCR products were purified (Qiagen) and sent to the Notre Dame Genomics Core for sequencing analysis or subjected to restriction enzyme digest with NdeI (New England BioLabs) and separation on a 2% agarose gel to identify WT, heterozygous, or mutant samples. Antisense morpholino oligonucleotides (MOs) were obtained from Gene Tools, LLC (Philomath, OR) and solubilized in DNase/RNase-free water to create 4 mM stock solutions which were then stored at −20°C. Zebrafish embryos were injected at the 1 cell stage with 1–2 nL of diluted MO. ppargc1a was targeted with the following: ATG MO1 ‘MO1’ 5’–CCTGATTACACCTGTCCCACGCCAT–3’ (400 µM) (Hanai et al., 2007; Bertrand et al., 2007), Splice MO1 ‘SB MO1’ 5’–GGAGCTTCTTCAGCTACAAACAGAG–3’ (400 µM), and Splice MO2 ‘SB MO2’ 5’–GGTGAGCAGCTACCTTGGCAACAGC–3’ (400 µM). tbx2b knockdowns were performed with an ATG MO 5’–CCTGTAAAAACTGGATCTCTCATCGG–3’ (400 µM) (Gross and Dowling, 2005; Drummond et al., 2017). To target sim1a, a splice blocking MO 5’–TGTGATTGTGTACCTGAAGCAGATG–3’ (400 µM) was used (Löhr et al., 2009; Cheng and Wingert, 2015). RT-PCR was completed to determine efficacy of the sim1a splice MO knockdown as previously described (Marra and Wingert, 2016). To complete RT-PCR the following primers were used: ppargc1a-FWD 5’–AATGCCAGTGATCAGAGCTGTCCTT–3’, ppargc1a-RVS 5’–CAGCTCAGTGCAGGGACGTCTCATG–3’, sim1a-FWD 5’–GAATCTTGGGGCCATGTGAGTCGAACGACTTCACTGG–3’, sim1a-RVS 5’–GTACAGGATTTTCCCATCAGGAGCCACCACAAAGATG–3’.
Quantitative real-time PCR
Request a detailed protocolGroups of 25–30 bezafibrate treated and vehicle control zebrafish were pooled with their respective group at the 28 ss. RNA was extracted using TRIZOL (Ambion) following the manufacturer instructions. cDNA was generated by qScript cDNA SuperMix (QuantaBio). qRT-PCR reactions were completed using PerfeCTa SYBR Green SuperMix with ROX (QuantaBio). To target ppargc1a, 100 ng of cDNA was optimal. For 18S control 1 ng was optimal. Primers used to amplify ppargc1a were forward 5’–AATGCCAGTGATCAGAGCTGTCCTT–3’ and reverse 5’–GTTCTGTGCCTTGCCACCTGGGTAT–3’. To target 18S the primers were as follows: forward 5’–TCGGCTACCACATCCAAGGAAGGCAGC–3’ and reverse 5’–TTGCTGGAATTACCGCGGCTGCTGGCA–3’. The AB StepOnePlus quantitative real time PCR machine program was: holding stage 2 min at 50°C, holding stage for 10 min at 95°C, then cDNA was amplified during 40 cycles, alternating between 15 s at 95°C to denature the cDNA and 1 min at 62°C for primer annealing and product extension. Data were recorded after each cycle to obtain the Ct values. Three technical replicates were completed for each of the three biological replicates for both treatments with the median Ct value normalized to control. Delta delta Ct was used for data analysis with 18S as a reference gene and the results calculated as relative expression change relative to DMSO control. For statistical analysis a Student’s t test was performed using the delta Ct values obtained after normalization to the 18S reference gene.
cRNA synthesis, and microinjections, rescue studies
Request a detailed protocolThe zebrafish ppargc1a ORF was cloned in to a pUC57 vector flanked by a 5’ KOZAK sequence, a Cla1 restriction enzyme site, and a SP6 promoter region, and on the 3’ by a series of STOP codons, a SV40 poly A tail, a Not1 restriction enzyme site, and a t7 promoter region. ppargc1a cRNA was generated by linearizing with Not1 and sp6 run off with the mMESSAGE mMACHINE SP6 Transcription kit (Ambion). cRNA was injected into WT and ppargc1asa13186 mutants at the one-cell stage at a concentration of 900 pg. Rescue studies were completed by performing WISH on injected ppargc1asa13186 mutants, then samples were imaged and genotyped. A minimum of three samples for each genotype was used to calculate segment phenotypes.
Image acquisition and quantification of phenotypes
Request a detailed protocolWISH images were acquired using a Nikon Eclipse Ni with a DS-Fi2 camera. FISH and immunofluorescence images were acquired using a Nikon C2 confocal microscope. Segment phenotypes were quantified using the Nikon Elements imaging software polyline tool. Unless otherwise stated, a minimum of three representative samples from each biological replicate were imaged and measured. Experiments were completed in triplicate. From these measurements, an average and standard deviation (SD) were calculated, and unpaired t-tests or one-way ANOVA tests were completed to compare control and experimental measurements.
Data availability
All data generated or analysed during this study are included in the manuscript and supporting files.
References
-
Zebrafish Whole Mount High-Resolution Double Fluorescent In Situ HybridizationJournal of Visualized Experiments 25:pii1229.https://doi.org/10.3791/1229
-
DataSanger Institute Zebrafish Mutation Project mutant data submissionZFIN Direct Data Submission. ID ZDB-PUB-130425-4.
-
Hepatocyte nuclear factor-1β controls mitochondrial respiration in renal tubular cellsJournal of the American Society of Nephrology 28:3205–3217.https://doi.org/10.1681/ASN.2016050508
-
Renal progenitors: Roles in kidney disease and regenerationWorld Journal of Stem Cells 8:367–375.https://doi.org/10.4252/wjsc.v8.i11.367
-
Evolving technology: creating kidney organoids from stem cellsAIMS Bioengineering 3:305–318.https://doi.org/10.3934/bioeng.2016.3.305
-
Flat mount preparation for observation and analysis of zebrafish embryo specimens stained by whole mount in situ hybridizationJournal of Visualized Experiments 89:e51604.https://doi.org/10.3791/51604
-
PGC-1 coactivator activity is required for murine erythropoiesisMolecular and Cellular Biology 34:1956–1965.https://doi.org/10.1128/MCB.00247-14
-
Peroxisome proliferator-activated receptor- coactivator 1- (PGC1 ) is a metabolic regulator of intestinal epithelial cell fateProceedings of the National Academy of Sciences 108:6603–6608.https://doi.org/10.1073/pnas.1016354108
-
Insights into kidney stem cell development and regeneration using zebrafishWorld Journal of Stem Cells 8:22–31.https://doi.org/10.4252/wjsc.v8.i2.22
-
Analysis of the human tissue-specific expression by genome-wide integration of transcriptomics and antibody-based proteomicsMolecular & Cellular Proteomics 13:397–406.https://doi.org/10.1074/mcp.M113.035600
-
The mouse Gene Expression Database (GXD) updateNucleic Acids Research 32:D730–D736.https://doi.org/10.1093/nar/gkh069
-
Combinatorial regulation of novel erythroid gene expression in zebrafishExperimental Hematology 36:424–432.https://doi.org/10.1016/j.exphem.2007.11.015
-
Rargb regulates organ laterality in a zebrafish model of right atrial isomerismDevelopmental Biology 372:178–189.https://doi.org/10.1016/j.ydbio.2012.09.001
-
Kidney organogenesis in the zebrafish: insights into vertebrate nephrogenesis and regenerationWiley Interdisciplinary Reviews: Developmental Biology 2:559–585.https://doi.org/10.1002/wdev.92
-
PGC-1α Protects from Notch-Induced Kidney Fibrosis DevelopmentJournal of the American Society of Nephrology 28:3312–3322.https://doi.org/10.1681/ASN.2017020130
-
The muscle-specific ubiquitin ligase atrogin-1/MAFbx mediates statin-induced muscle toxicityJournal of Clinical Investigation 117:3940–3951.https://doi.org/10.1172/JCI32741
-
Stages of embryonic development of the zebrafishDevelopmental Dynamics 203:253–310.https://doi.org/10.1002/aja.1002030302
-
PGC-1, a versatile coactivatorTrends in Endocrinology & Metabolism 12:360–365.https://doi.org/10.1016/S1043-2760(01)00457-X
-
Expression of the zebrafish paired box gene pax[zf-b] during early neurogenesisDevelopment 113:1193–1206.
-
Interactions between Cdx genes and retinoic acid modulate early cardiogenesisDevelopmental Biology 354:134–142.https://doi.org/10.1016/j.ydbio.2011.03.027
-
Conserved and Divergent Features of Human and Mouse Kidney OrganogenesisJournal of the American Society of Nephrology 29:ASN.2017080887.https://doi.org/10.1681/ASN.2017080887
-
PGC1α in the kidneyAmerican Journal of Physiology-Renal Physiology 314:F1–F8.https://doi.org/10.1152/ajprenal.00263.2017
-
Visualizing multiciliated cells in the zebrafish through a combined protocol of whole mount fluorescent in situ hybridization and immunofluorescenceJournal of Visualized Experiments 129:e56261.https://doi.org/10.3791/56261
-
Analysis of nephron composition and function in the adult zebrafish kidneyJournal of Visualized Experiments 90:e51644.https://doi.org/10.3791/51644
-
Atlas of Cellular Dynamics during Zebrafish Adult Kidney RegenerationStem Cells International 2015:1–19.https://doi.org/10.1155/2015/547636
-
New tides: using zebrafish to study renal regenerationTranslational Research 163:109–122.https://doi.org/10.1016/j.trsl.2013.10.003
-
Temporal and spatial expression of tight junction genes during zebrafish pronephros developmentGene Expression Patterns 16:104–113.https://doi.org/10.1016/j.gep.2014.11.001
-
A manual small molecule screen approaching high-throughput using zebrafish embryosJournal of Visualized Experiments 93:e52063.https://doi.org/10.3791/52063
-
Little fish, big catch: zebrafish as a model for kidney diseaseKidney International 89:1204–1210.https://doi.org/10.1016/j.kint.2016.01.031
-
Comparative analysis of Pax-2 protein distributions during neurulation in mice and zebrafishMechanisms of Development 38:197–208.https://doi.org/10.1016/0925-4773(92)90053-M
-
Renal progenitors: an evolutionary conserved strategy for kidney regenerationNature Reviews Nephrology 9:137–146.https://doi.org/10.1038/nrneph.2012.290
-
Cardiac-specific induction of the transcriptional coactivator peroxisome proliferator-activated receptor gamma coactivator-1alpha promotes mitochondrial biogenesis and reversible cardiomyopathy in a developmental stage-dependent mannerCirculation Research 94:525–533.https://doi.org/10.1161/01.RES.0000117088.36577.EB
-
PGC-1α regulates normal and pathological angiogenesis in the retinaThe American Journal of Pathology 182:255–265.https://doi.org/10.1016/j.ajpath.2012.09.003
-
Role of Esrrg in the fibrate-mediated regulation of lipid metabolism genes in human ApoA-I transgenic miceThe Pharmacogenomics Journal 10:165–179.https://doi.org/10.1038/tpj.2009.51
-
Early organogenesis of the kidneyPediatric Nephrology 1:385–392.https://doi.org/10.1007/BF00849241
-
DataExpression from: unexpected novel relational links uncovered by extensive developmental profiling of nuclear receptor expressionZFIN Direct Data Submission. ID ZDB-PUB-080220-1.
-
PGC-1α promotes recovery after acute kidney injury during systemic inflammation in miceJournal of Clinical Investigation 121:4003–4014.https://doi.org/10.1172/JCI58662
-
The zebrafish pronephros: a model to study nephron segmentationKidney International 73:1120–1127.https://doi.org/10.1038/ki.2008.37
Article and author information
Author details
Funding
National Institutes of Health (R01DK100237)
- Rebecca A Wingert
The funders had no role in study design, data collection and interpretation, or the decision to submit the work for publication.
Acknowledgements
NIH Grant R01DK100237 to RAW supported this work. We are grateful to Elizabeth and Michael Gallagher for a generous gift to the University of Notre Dame on behalf of their family for the support of stem cell research. The funders had no role in the study design, data collection and analysis, decision to publish, or manuscript preparation. We thank the staffs of the Department of Biological Sciences and the Center for Zebrafish Research at the University of Notre Dame for their dedication and care of our zebrafish aquarium. We thank ED for contributions to preliminary investigations on the effects of bezafibrate on zebrafish nephron development. We also extend special thanks to LG for assistance with analytical tools. Finally, we thank all the current and previous members of our lab for their support, discussions, and insights about this work.
Ethics
Animal experimentation: Zebrafish were maintained in the Center for Zebrafish Research at the University of Notre Dame. All studies were performed with approval of the University of Notre Dame Institutional Animal Care and Use Committee (IACUC), under protocol numbers 13-021 and 16-025.
Copyright
© 2018, Chambers et al.
This article is distributed under the terms of the Creative Commons Attribution License, which permits unrestricted use and redistribution provided that the original author and source are credited.
Metrics
-
- 1,678
- views
-
- 231
- downloads
-
- 26
- citations
Views, downloads and citations are aggregated across all versions of this paper published by eLife.
Citations by DOI
-
- 26
- citations for umbrella DOI https://doi.org/10.7554/eLife.40266