Multicellularity: From brief encounters to lifelong unions
When multicellular organisms developed from unicellular ancestors, it was a major evolutionary transition (Maynard Smith and Szathmary, 1995). Multicellular life is thought to have evolved by two mechanisms—clonal development or aggregative development (Grosberg and Strathmann, 2007; Figure 1)—but we are just beginning to understand its genetic basis.
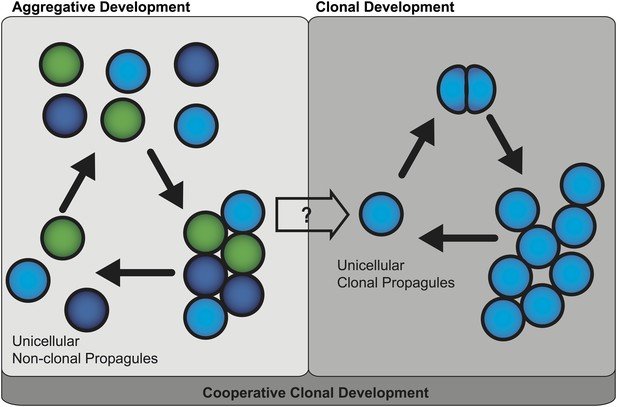
Two mechanisms of multicellular evolution.
On the left, organisms that evolved multicellularity by aggregative development have a life cycle where individuals from the environment aggregate, and cooperate to form a multicellular organism. These cells need not be genetically identical (indicated by the different colors). In many organisms with this life cycle, only some cells are dispersed for reproduction. On the right, organisms that evolved multicellularity by clonal development remain attached together after each cell division, forming groups of undifferentiated cells. Each cell in the group can produce a genetically identical reproductive cell, or ‘propagule’, that produces genetically uniform offspring. If aggregative development and clonal development are both important for multicellular evolution in metazoa, as the results of Sebé-Pedrós et al. suggest, then a new unified mechanism—‘cooperative clonal development’—is required.
In plants and animals, multicellularity is thought to have evolved as a result of clonal development (King, 2004; Rokas, 2008). Here, ancient unicellular organisms evolved methods of cell-cell adhesion that prevented their cells from fully separating after cell division. At first these organisms resembled clumps of undifferentiated cells, but later cells within these organisms specialized, allowing the evolution of complex and differentiated tissues. Significantly, the organisms that evolved multicellularity by clonal development must go through a single cell stage every generation, which means that all the cells in each multicellular organism are genetically identical.
Aggregative development, on the other hand, is thought to be the less common mechanism because it is typically observed in organisms with unusual life cycles, such as the slime molds and slime bacteria (Bonner, 2000; Rokas, 2008). Aggregative development involves previously free-living, single cells gathering together and cooperating to form a multicellular organism. As such, the cells in the resultant organism may not necessarily be genetically identical. Moreover, in most organisms that undergo aggregative development, only subsets of cells are dispersed for reproduction. This means that these organisms do not always have to go through the ‘unicellular bottleneck’ that limits genetic diversity during clonal development (Grosberg and Strathmann, 2007).
One potential disadvantage of aggregative development is that individual cells can act selfishly or ‘cheat’ to ensure they are selected for reproduction, even if this reduces the fitness of the multicellular organisms as a whole. Because of this, it is assumed that the cost of actively preventing cheating in aggregative organisms limits their potential to evolve complex tissues and organs (Bonner, 2000). As such, the unicellular bottleneck is considered important for the evolution of complex tissues in plants and animals (Grosberg and Strathmann, 2007). Now Iñaki Ruiz-Trillo of the Institut de Biologia Evolutiva and the University of Barcelona, Benjamin Blencowe of the University Toronto and co-workers—including Arnau Sebé-Pedrós as first author—have challenged this assumption by examining the life cycle of a close unicellular relative of the multicellular animals or ‘metazoa’, Capsaspora owczarzaki (Sebé-Pedrós et al., 2013).
Metazoans evolved from a primitive amoeba- or fungal-like unicellular organism between about 0.8 and 1 billion years ago. Although very few relatives of these unicellular pre-metazoans exist today (King, 2004; Rokas, 2008), the choanoflagellates were amongst the first to be recognized as such (King, 2004). Representative organisms are found as either unicellular organisms, or in clonal groups called ‘rosettes’. Since these multicellular rosettes are formed by cell division in which the daughter cells do not separate, this has been considered to support a clonal development origin for metazoan. However, the recent discovery that choanoflagellate multicellularity is influenced by compounds produced by a symbiotic bacteria suggests that our understanding of the events that lead to animal multicellularity may be incomplete (Alegado et al., 2012).
Recently, a new group of amoeba-like organisms that are slightly more distant relatives of the metazoa were discovered (Steenkamp et al., 2006; Ruiz-Trillo et al., 2007, 2008; Shalchian-Tabrizi et al., 2008; Torruella et al., 2012; Suga et al., 2013). The genome of one of these species, Capsaspora owczarzarki, was sequenced and was found to contain several families of proteins that were thought to absent in unicellular pre-metazoans (King et al., 2008). This means that these protein families were most likely present in the ancestors of the metazoans, but have subsequently been lost in the choanoflagellates. Indeed with the completion of genome sequences for organisms occupying the lower branches of the metazoan family tree, a short list of gene families required for the evolution of multicellular animals is now available (Suga et al., 2013). However, these comparative genomics studies have yet to determine the specific genes that were required for the first steps toward clonal multicellularity.
Now Sebé-Pedrós, Ruiz-Trillo, Blencowe and colleagues—who are based in Barcelona, Toronto and the Broad Institute—report the surprising discovery that this species has an aggregative life cycle, not a clonal life cycle as expected. Sebé-Pedrós et al. have also identified a small subset of genes that are linked to aggregative multicellularity. Capsaspora multiplies as amoeba-like cells with small finger-like projections, called filipodia, and these allow the cells to move across a surface in search of nutrients. After the filopodial stage, and presumably when nutrients are exhausted, the cells follow one of two developmental fates. In some cases cells retract their filopodia, detach from the surface and form individual cysts. However, cells can follow an alternative pathway where they randomly aggregate, and produce a thickened extracellular matrix that holds them together. These multicellular aggregates can later break down into individual cysts, possibly aiding in dispersal. The discovery that a close unicellular relative of metazoa has an aggregative life cycle stage challenges the idea that clonal development led to the evolution of multicellular metazoans.
Sebé-Pedrós et al. also compared the gene expression profiles of these three life cycle stages. Filopodial cells show the expected signs of cell proliferation; cysts, on the other hand, show a signature of cell starvation and signs of entering into a dormant state. However, the aggregative cells express genes that are required for cell-cell adhesion and cell-cell communication, but are not expressed during the other life cycle stages. Furthermore, as the specific proteins involved in cell-cell adhesion and cell-cell communication may interact with each other, this suggests that these two pathways might have co-evolved. If future work shows that these two pathways did co-evolve, it suggests a strong link between them and the evolution of aggregative development.
Thus these findings question whether clonal development and aggregative development are really two distinct mechanisms of multicellular evolution. One possible scenario for the evolution of multicellular metazoa is a combination of these two mechanisms, or ‘cooperative clonal development’ (Figure 1). In this scenario when, environmental conditions became adverse, unicellular pre-metazoans were able to cooperate and form aggregates of similar cells. At some point, cooperative aggregation became a selective advantage that led to more permanent cell-cell adhesion. Subsequently, clonal development became a selective advantage by short-circuiting reproductive dispersal of individual cells. Once clonal development evolved, genetic uniformity was selected for through a single cell bottleneck. The evolution of clonal development thus provided a selective advantage in controlling cheating, thus allowing the evolution of complex developmental patterns that are the hallmark of animal diversity present today.
References
-
BookFirst signals. The evolution of multicellular developmentPrinceton, NJ: Princeton University Press.
-
The evolution of multicellularity: a Minor major transition?Annual Review of Ecology, Evolution, and Systematics 38:621–654.https://doi.org/10.1146/annurev.ecolsys.36.102403.114735
-
The unicellular ancestry of animal developmentDevelopmental Cell 7:313–325.https://doi.org/10.1016/j.devcel.2004.08.010
-
The origins of multicellularity: a multi-taxon genome InitiativeTrends in Genetics 23:113–118.https://doi.org/10.1016/j.tig.2007.01.005
-
A phylogenomic investigation into the origin of metazoaMolecular Biology and Evolution 25:664–672.https://doi.org/10.1093/molbev/msn006
-
The Protistan origins of animals and fungiMolecular Biology and Evolution 23:93–106.https://doi.org/10.1093/molbev/msj011
-
The Capsaspora genome reveals a complex unicellular prehistory of animalsNature Communications 4:2325.https://doi.org/10.1038/ncomms3325
-
Phylogenetic relationships within the Opisthokonta based on phylogenomic analyses of conserved single-copy protein domainsMolecular Biology and Evolution 29:531–544.https://doi.org/10.1093/molbev/msr185
Article and author information
Author details
Publication history
- Version of Record published: December 24, 2013 (version 1)
Copyright
© 2013, Olson
This article is distributed under the terms of the Creative Commons Attribution License, which permits unrestricted use and redistribution provided that the original author and source are credited.
Metrics
-
- 1,288
- views
-
- 125
- downloads
-
- 11
- citations
Views, downloads and citations are aggregated across all versions of this paper published by eLife.
Download links
Downloads (link to download the article as PDF)
Open citations (links to open the citations from this article in various online reference manager services)
Cite this article (links to download the citations from this article in formats compatible with various reference manager tools)
Further reading
-
- Evolutionary Biology
The RNA world hypothesis proposes that during the early evolution of life, primordial genomes of the first self-propagating evolutionary units existed in the form of RNA-like polymers. Autonomous, non-enzymatic, and sustained replication of such information carriers presents a problem, because product formation and hybridization between template and copy strands reduces replication speed. Kinetics of growth is then parabolic with the benefit of entailing competitive coexistence, thereby maintaining diversity. Here, we test the information-maintaining ability of parabolic growth in stochastic multispecies population models under the constraints of constant total population size and chemostat conditions. We find that large population sizes and small differences in the replication rates favor the stable coexistence of the vast majority of replicator species (‘genes’), while the error threshold problem is alleviated relative to exponential amplification. In addition, sequence properties (GC content) and the strength of resource competition mediated by the rate of resource inflow determine the number of coexisting variants, suggesting that fluctuations in building block availability favored repeated cycles of exploration and exploitation. Stochastic parabolic growth could thus have played a pivotal role in preserving viable sequences generated by random abiotic synthesis and providing diverse genetic raw material to the early evolution of functional ribozymes.
-
- Ecology
- Evolutionary Biology
Seasonal animal dormancy is widely interpreted as a physiological response for surviving energetic challenges during the harshest times of the year (the physiological constraint hypothesis). However, there are other mutually non-exclusive hypotheses to explain the timing of animal dormancy, that is, entry into and emergence from hibernation (i.e. dormancy phenology). Survival advantages of dormancy that have been proposed are reduced risks of predation and competition (the ‘life-history’ hypothesis), but comparative tests across animal species are few. Using the phylogenetic comparative method applied to more than 20 hibernating mammalian species, we found support for both hypotheses as explanations for the phenology of dormancy. In accordance with the life-history hypotheses, sex differences in hibernation emergence and immergence were favored by the sex difference in reproductive effort. In addition, physiological constraint may influence the trade-off between survival and reproduction such that low temperatures and precipitation, as well as smaller body mass, influence sex differences in phenology. We also compiled initial evidence that ectotherm dormancy may be (1) less temperature dependent than previously thought and (2) associated with trade-offs consistent with the life-history hypothesis. Thus, dormancy during non-life-threatening periods that are unfavorable for reproduction may be more widespread than previously thought.