Dopamine System: Mapping neural circuits with CLARITY
How can a relatively small number of neurons influence aspects of behavior as diverse as motivation, learning, reward and movement by releasing just one type of molecule? This is the challenge facing neuroscientists working on the dopamine system – a population of ∼30,000 neurons that are located in just two regions of the brain.
At first it was thought that the neurons in the dopamine system were all quite similar, but over the past decade it has become clear that they exhibit a range of molecular, anatomical and functional properties (Lammel et al., 2008; Margolis et al., 2008; Lammel et al., 2011). Moreover, it seems that distinct subsets of dopamine neurons belong to discrete circuits that carry out different functions, although the architecture of these circuits remains an open question. For the most part, projections from a given subset of dopamine neurons target one region of the brain (Yetnikoff et al., 2014), but projections to a given subset arise from many different regions. It has been challenging to determine whether the different subsets all receive the same inputs, or whether some regions of the brain project more to one subset than another.
Now, in eLife, Mitsuko Watabe-Uchida and colleagues at Harvard University and the Cold Spring Harbor Laboratory – including William Menegas as first author – report that almost all dopamine neurons receive the same set of inputs from a range of brain regions (Menegas et al., 2015). However, one exception is a subset of dopamine neurons that project to a region called the tail of the striatum (Figure 1).
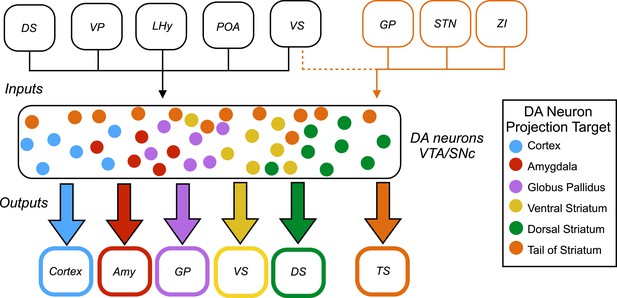
Inputs and outputs of dopamine neurons.
Dopamine (DA) neurons are found in the ventral tegmental area (VTA) and the substantia nigra pars compacta (SNc). Dopamine neurons projecting to the tail of the striatum (TS) receive the majority of their input from the globus pallidus (GP), the subthalamic nucleus (STN), and the zona incerta (ZI), with a small amount of input coming from the ventral striatum (VS). Dopamine neurons that project to the cortex, amygdala (Amy), globus pallidus, ventral striatum and dorsal striatum (DS) receive the majority of their inputs from the following regions: the ventral striatum, dorsal striatum, ventral pallidum (VP), lateral hypothalamus (LHy), and preoptic area (POA). In Parkinson’s disease, dopamine neurons projecting to the posterior putamen (which is functionally similar to the tail of the striatum) are the first to degenerate (Kish et al., 1988), so their unique pattern of inputs is especially interesting to researchers.
Menegas et al. used a combination of a powerful anatomical technique called CLARITY (Chung et al., 2013) and light-sheet microscopy to map the input and output projections of dopamine neurons in an intact mouse brain. First, subsets of dopamine neurons were classified according to which of eight regions – medial pre-frontal cortex, orbitofrontal cortex, central amygdala, globus pallidus, ventral striatum, dorsal striatum, tail of the striatum, or lateral habenula – they projected onto. Next, a given subset, based on its projection target, was infected to express two proteins (avian retroviral receptor and rabies virus envelop glycoprotein). Then, three weeks later, a modified rabies virus was injected into the dopamine neurons. This virus spreads retrogradely and labels neurons projecting to the dopamine neurons with green fluorescent protein. Menegas et al. had to develop a suite of new data acquisition and analysis tools to map the 3D position of the fluorescently labeled neurons and align their results across animals.
This analysis revealed that most subsets of dopamine neurons receive similar inputs from a number of regions of the brain, primarily from the ventral striatum and the hypothalamus, regardless of projection target (Figure 1). This suggests that functional specialization in the dopamine system arises within the two regions where the dopamine neurons are found – the ventral tegmental area and the substantia nigra pars compacta – or downstream from these regions.
A notable exception was the subset of dopamine neurons that project to the tail of the striatum: the majority of the inputs to this subset came from regions of the brain that do not project strongly onto the other subsets (Figure 1). This suggests that the activity of this subset of neurons is regulated in a way that differs from the regulation of the other subsets of dopamine neurons.
The findings of Menegas et al. largely complement recent results on the organization of neural circuits within the dopamine system from two other groups, but a few differences are worth noting. Within the substantia nigra pars compacta, Lerner et al. observed a reciprocally connected architecture, with the neurons that project to the dorsomedial striatum receiving more input from this region, and likewise for the neurons that project to the dorsolateral striatum (Lerner et al., 2015). Additionally, Beier et al. observed a ‘biased input/discrete output’ architecture within the ventral tegmental area, with neurons projecting to the medial nucleus accumbens and the lateral nucleus accumbens receiving different patterns of input (Beier et al., 2015). These discrepancies may reflect some limitations of the automated analysis used by Menegas et al.
These results from Menegas et al. show that the outputs of dopaminergic neurons are more distinctive then their inputs. This has implications for how and where specialization of dopami-nergic circuits arises, and suggests that many important computations are performed locally within the ventral tegmental area and the substantia nigra pars compacta. An immediate challenge is to gain a deeper understanding of the local microcircuit dynamics that govern the activity of dopamine neurons within these two regions.
References
Article and author information
Author details
Publication history
- Version of Record published: October 9, 2015 (version 1)
Copyright
© 2015, Willard and Gittis
This article is distributed under the terms of the Creative Commons Attribution License, which permits unrestricted use and redistribution provided that the original author and source are credited.
Metrics
-
- 3,993
- views
-
- 571
- downloads
-
- 8
- citations
Views, downloads and citations are aggregated across all versions of this paper published by eLife.
Download links
Downloads (link to download the article as PDF)
Open citations (links to open the citations from this article in various online reference manager services)
Cite this article (links to download the citations from this article in formats compatible with various reference manager tools)
Further reading
-
- Neuroscience
Nociceptive sensory neurons convey pain-related signals to the CNS using action potentials. Loss-of-function mutations in the voltage-gated sodium channel NaV1.7 cause insensitivity to pain (presumably by reducing nociceptor excitability) but clinical trials seeking to treat pain by inhibiting NaV1.7 pharmacologically have struggled. This may reflect the variable contribution of NaV1.7 to nociceptor excitability. Contrary to claims that NaV1.7 is necessary for nociceptors to initiate action potentials, we show that nociceptors can achieve similar excitability using different combinations of NaV1.3, NaV1.7, and NaV1.8. Selectively blocking one of those NaV subtypes reduces nociceptor excitability only if the other subtypes are weakly expressed. For example, excitability relies on NaV1.8 in acutely dissociated nociceptors but responsibility shifts to NaV1.7 and NaV1.3 by the fourth day in culture. A similar shift in NaV dependence occurs in vivo after inflammation, impacting ability of the NaV1.7-selective inhibitor PF-05089771 to reduce pain in behavioral tests. Flexible use of different NaV subtypes exemplifies degeneracy – achieving similar function using different components – and compromises reliable modulation of nociceptor excitability by subtype-selective inhibitors. Identifying the dominant NaV subtype to predict drug efficacy is not trivial. Degeneracy at the cellular level must be considered when choosing drug targets at the molecular level.
-
- Neuroscience
Despite substantial progress in mapping the trajectory of network plasticity resulting from focal ischemic stroke, the extent and nature of changes in neuronal excitability and activity within the peri-infarct cortex of mice remains poorly defined. Most of the available data have been acquired from anesthetized animals, acute tissue slices, or infer changes in excitability from immunoassays on extracted tissue, and thus may not reflect cortical activity dynamics in the intact cortex of an awake animal. Here, in vivo two-photon calcium imaging in awake, behaving mice was used to longitudinally track cortical activity, network functional connectivity, and neural assembly architecture for 2 months following photothrombotic stroke targeting the forelimb somatosensory cortex. Sensorimotor recovery was tracked over the weeks following stroke, allowing us to relate network changes to behavior. Our data revealed spatially restricted but long-lasting alterations in somatosensory neural network function and connectivity. Specifically, we demonstrate significant and long-lasting disruptions in neural assembly architecture concurrent with a deficit in functional connectivity between individual neurons. Reductions in neuronal spiking in peri-infarct cortex were transient but predictive of impairment in skilled locomotion measured in the tapered beam task. Notably, altered neural networks were highly localized, with assembly architecture and neural connectivity relatively unaltered a short distance from the peri-infarct cortex, even in regions within ‘remapped’ forelimb functional representations identified using mesoscale imaging with anaesthetized preparations 8 weeks after stroke. Thus, using longitudinal two-photon microscopy in awake animals, these data show a complex spatiotemporal relationship between peri-infarct neuronal network function and behavioral recovery. Moreover, the data highlight an apparent disconnect between dramatic functional remapping identified using strong sensory stimulation in anaesthetized mice compared to more subtle and spatially restricted changes in individual neuron and local network function in awake mice during stroke recovery.