A novel N-terminal extension in mitochondrial TRAP1 serves as a thermal regulator of chaperone activity
Decision letter
-
Jeffery W KellyReviewing Editor; Scripps Research Institute, United States
eLife posts the editorial decision letter and author response on a selection of the published articles (subject to the approval of the authors). An edited version of the letter sent to the authors after peer review is shown, indicating the substantive concerns or comments; minor concerns are not usually shown. Reviewers have the opportunity to discuss the decision before the letter is sent (see review process). Similarly, the author response typically shows only responses to the major concerns raised by the reviewers.
Thank you for sending your work entitled “A novel N-terminal extension in mitochondrial Hsp90 (TRAP1) serves as a thermal regulator of chaperone activity” for consideration at eLife. Your article has been favorably evaluated by John Kuriyan (Senior editor), a Reviewing editor, and 2 reviewers.
The Reviewing editor and the reviewers have commented positively on your manuscript. Both reviewers have identified one point that needs additional support. This issue concerns the fact that the physiological relevance of the work is not so clear, since the major effects for human TRAP1 happen at temperatures below 37°C. Both reviewers were interested in seeing data recorded at higher temperatures. Please address this important issue in the revised manuscript and as many of the comments in the two reviews as possible.
Reviewer #1
Hsp90 is a molecular chaperone found in prokaryotes and in the cytosol as well as organelles of the eukaryotic cell. Its function is coupled to ATP hydrolysis along with associated large conformational changes. While the key steps of the Hsp90 ATPase cycle are understood, the regulation of these structural rearrangements is still elusive. Previously, the authors solved the crystal structure of mitochondrial Hsp90, TRAP1, bound to AMPPNP.
They identified a 14-residue extension of the N-terminal beta-strand which crosses over between protomers in the closed state. This 'strap' is found in higher eukaryotes but is absent in yeast and bacteria. The authors also showed that point mutations or the deletion of the strap in TRAP1 (of zebra fish) or endoplasmic Grp94 results in an increase in ATPase activity. In this study, they show that a temperature-dependent kinetic barrier limits the conformational changes from the apo to the closed form of TRAP1. At lower temperature (23°C) TRAP1is predominantly open, even in the presence of AMPPNP. Local conformational changes associated with lid closure are a part of the rate limiting step to the closed state and are regulated by the strap in TRAP1.
This is an interesting observation adding to our understanding of aspects of the conformational cycle of Hsp90 and species-specific differences.
However, the biological function of the temperature regulation remains unclear to me, assuming that 37°C may be the resting state of human mitochondria and higher temperature present during energy generation or under thermal stress conditions. Thus the regulation should be active at higher temperatures, such as 42°C. Experiments addressing this issue would be of interest.
Specific points:
1) The authors state that the kinetic barrier for closure is large and unusually sensitive to temperature changes. Examples should be included to allow for comparison.
2) The kinetic data should include fits and resulting rate constants (and also which equation was used) to judge quality of the kinetic model.
3) If the measured conformational change is indeed rate limiting then its temperature dependence should be the same as that of the ATPase activity measured before. Is this indeed the case? How is the relationship of the shortened variant?
4) Figure 1: Do other Hsp90 isoforms also show a similar trend over a temperature range or is this special just for TRAP1?
5) Experiments in Figure 1 and 2 do not show kinetics, just a shift of equilibria.
6) EM images for delta strap should be included.
7) In an Agard publication from 2008 (Southworth & Agard, Cell 2008) EM images of HtpG, yeast and human Hsp90 are shown in the apo state, with AMPPNP, and with ADP. The human Hsp90α is in an open conformation with AMPPNP at 37°C (according to them TRAP1 is fully closed at 37°C).
8) Figure 2–figure supplement 1: Isn't this the same figure as Figure 2C just without the delta-strap?
9) Is it known that the trans contacts that strap forms in the closed conformation stabilize the closed conformation?
10) Figure 4A: For the NTD:MD rotation, the FRET probe does not show a significant change in the FRET signal.
11) Figure 4B: Why was saturation not reached for the 42°C sample?
12) Data for the dimer closure FRET construct should be included and it would be important to see how delta-strap acts in the FRET assay at different temperatures to compare with the SAXS data.
13) Why was the double strap mutant too unstable for NTD rotation FRET and not for inter-protomer FRET? Isn't it the same construct just with a different Cys label?
14) Figure 5A: The legend says that in the apo state the lid probe is in equilibrium between mobile and immobile states. In the Results section it is stated that the apo form is predominantly immobile.
15) According to the authors, deleting strap compromises the stability of the closed structure and hence enhances the reopening rate and shifts the equilibrium towards the open state. If deleting strap shifts the equilibrium towards the open state, why is the delta-strap construct predominantly closed?
In this context, the authors mention that the effect of deleting the strap on the opening of the NTD interface is smaller than the effect on the kinetic barrier corresponding to the release of strap from the apo state. The respective numbers seem to be missing from Table 3.
16) Are the ATPase activities of the cys-free version of hTRAP1 and zebrafish TRAP1 mutant identical to the respective wild type proteins?
17) Figure 6. The legend says delta strap is ∼7 fold faster; text says ∼8 fold faster.
18) Figure 6C: a trace showing that in presence of ATP without Mg2+, there is no ATPase activity should be added. Not adding Mg2+ is not necessarily equivalent to not having (ambient) Mg2+ present in the solution. The ATP induced changes in FRET signals should be measured also in the presence of EDTA.
19) The authors mention that in the absence of Mg2+, ATP and AMPPNP show pronounced differences in kinetics of FRET signal, the difference with/without Mg2+ should be even more pronounced, that is the kinetics of o/c may be substantially faster in the presence of Mg2+. Measuring the FRET kinetics upon addition of Mg-ATP and Mg-AMPPNP is crucial to show that ATP induced closing kinetics in absence of Mg2+ are indeed representative for the ATPase cycle.
20) How does one know that Mg2+ can actually bind to the closed form; and that a re-open is not necessary for this to happen?
Reviewer #2
In this study, Partridge and colleagues investigate the role of the N-terminal extension (“strap”) of TRAP1, the mitochondrial Hsp90 isoform, which in the previously solved crystal structure of the TRAP1 dimer wraps around the N-domain of the opposite protomer. They characterized the effects of the strap on the dimer closure kinetics, the rotation of the N-domain relative to the M-domain, the ATP hydrolysis and movement of the ATP lid (N-domain) using negative stain electron microscopy (EM), small angle x-ray scattering (SAXS), fluorescence resonance energy transfer (FRET) and electron paramagnetic resonance (EPR) measurements. They demonstrate that the strap region is responsible for a temperature-dependent increase in the rate of TRAP1 closure, as well as the increase in the ATPase activity.
The data presented here is convincing and interesting. The physiological relevance of the observed phenomenon is not so clear since the major effects for human TRAP1 happen at temperatures below 37°C. Nevertheless, the story could be published after the authors addressed the raised issues.
Major comments
1) Figure 1 shows negative stain EM images of TRAP1 in the presence of AMPPNP pre-incubated at different temperatures. Few representative samples are picked from each grid to show the transition from open to closed conformation with the increase in temperature. The authors should quantify the open and closed structures from a representative square of the electron micrograph.
2) Using SAXS the authors measured AMPPNP-induced transition of human and zebrafish TRAP1 to the closed conformation between 20 and 36°C (Figure 2). To determine the physiological relevance of their observations the authors could have measured the AMPPNP induced transition of human TRAP1 at 37 to 42°C. Does human TRAP1 become more active at heat shock temperatures?
3) Figure 4: The authors investigate AMPPNP-induced changes in TRAP1 conformation using FRET. Control experiments with only the acceptor dye need to be shown especially as the changes in fluorescence for the NTD:MD rotation seems to be very small. The temperature at which the experiment of Figure 4(A) has been performed should be mentioned in the figure legend.
4) The dimer closure FRET experiments have been performed only at 30°C. As the paper deals with effect of temperature on ATPase rates of TRAP1, it would be very important to see the change in rate of dimer closure at different temperatures. It would also be interesting whether the rates of NTD-MD-rotation and dimer closure are similar to each other.
5) In Table 2 the authors write that the steady state ATPase rate for human TRAP1 was 0.463 min-1 and in Table 3 the write that the closing rate for human TRAP1 at 30°C was 0.02 min-1. These values do not fit together and contradict the claims of the authors. The authors should indicate at which temperature the ATPase assays were performed and correlate the closing rate with the ATP hydrolysis rates to substantiate their claims. Maybe the authors will have to measure the closing rate upon addition of ATP instead of AMPPNP. This seems possible since omission of Mg prevents hydrolysis as the authors have shown.
https://doi.org/10.7554/eLife.03487.020Author response
The Reviewing editor and the other reviewers have commented positively on your manuscript. Both reviewers have identified one point that needs additional support. This issue concerns the fact that the physiological relevance of the work is not so clear, since the major effects for human TRAP1 happen at temperatures below 37°C. Both reviewers were interested in seeing data recorded at higher temperatures. Please address this important issue in the revised manuscript and as many of the comments in the two reviews as possible.
The major question echoed by all was whether the experiments done in this paper could be done at higher temperatures to better reflect how the observed temperature dependent activity of TRAP1 would be beneficial above homeostatic temperatures of the organism for the homologs tested. We have attempted to address these concerns by including new data recorded at temperatures above 37°C. 37°C data were already included in the original manuscript. This includes new electron micrographs of samples incubated at 42°C, SAXS data up to 43°C, and better explanation of previously recorded data demonstrating that the rate of ATPase activity in TRAP1 will continue to increase until 60°C, at which point TRAP1 begins to denature. The new data taken at temperatures above 37°C has been added to already existing figures.
The new EM data has been incorporated into Figure 1, panel A. The new SAXS data has been incorporated into Figure 2, panels A, B, C, D. Table 1 has also been updated to include data above 37°C, reflecting the changes made to Figure 2. We also responded to the reviewer’s requests by including FRET data for both types of probes, intra and now inter FRET (Figure 4B). The increasing temperature series for both sets of FRET probes behaves similarly and increases as a response to temperature with each assay having a comparable fold increase. Further we added extensive temperature series of closure kinetics measured using the inter FRET probe between 23 and 42°C for the delta strap variant of human TRAP1. Two new panels have been added with this data (Figure 4D, E). Importantly this data, together with that previously shown in Figure 4B allows calculation of an Arrhenius activation energy ±strap. This shows that the strap contributes ∼60% of the activation energy measured for WT TRAP1 (Figure 4E, Figure 4 legend and within the main text). Further, we have made every attempt to improve the manuscript as suggested.
These edits include changes to main text figures, as well as further edits to the text. We have additionally pointed out examples of data recorded at temperatures above 37°C that were included in the original submission, such as the FRET experiment measuring the temperature dependence of NTD rotation. These changes and our responses to individual comments by reviewers are discussed in more detail below.
Reviewer #1
Hsp90 is a molecular chaperone found in prokaryotes and in the cytosol as well as organelles of the eukaryotic cell. Its function is coupled to ATP hydrolysis along with associated large conformational changes. While the key steps of the Hsp90 ATPase cycle are understood, the regulation of these structural rearrangements is still elusive. Previously, the authors solved the crystal structure of mitochondrial Hsp90, TRAP1, bound to AMPPNP.
They identified a 14-residue extension of the N-terminal beta-strand which crosses over between protomers in the closed state. This 'strap' is found in higher eukaryotes but is absent in yeast and bacteria. The authors also showed that point mutations or the deletion of the strap in TRAP1 (of zebra fish) or endoplasmic Grp94 results in an increase in ATPase activity. In this study, they show that a temperature-dependent kinetic barrier limits the conformational changes from the apo to the closed form of TRAP1. At lower temperature (23⁰C) TRAP1is predominantly open, even in the presence of AMPPNP. Local conformational changes associated with lid closure are a part of the rate limiting step to the closed state and are regulated by the strap in TRAP1.
This is an interesting observation adding to our understanding of aspects of the conformational cycle of Hsp90 and species-specific differences.
However, the biological function of the temperature regulation remains unclear to me, assuming that 37⁰C may be the resting state of human mitochondria and higher temperature present during energy generation or under thermal stress conditions. Thus the regulation should be active at higher temperatures, such as 42⁰C. Experiments addressing this issue would be of interest.
We have attempted to address the concern of all reviewers by now including
SAXS and EM data taken at temperatures above 37°C. Both high temperature datasets agree with the basic observation that a compact or “closed” conformation dominates the population at equilibrium.
With EM we see that the predominant form is a closed conformation at higher temperatures as demonstrated in Figure 1. Additionally there continues to be an increase in the % closed population as measured with SAXS, Figure 2. This increase in the % closed population in both species has been tabulated in Table 1. Looking at both Figure 2 and Table 1 you can see that the % closed does continue to increase beyond 37 °C, although there is one outlier in all this data and that is WT zTRAP1, which shows a decrease in % closed. Presumably, temperatures above 37° C are physiologically irrelevant for zebrafish. That said, Δstrap zTRAP1 did continue to show an increase in the % closed population. Concerning steady-state ATP hydrolysis measurements at temperatures above 37 °C we also modified the text to make it more obvious that temperature dependence of ATPase had previously been characterized for TRAP1 by Johannes Buchner’s lab in manuscripts referenced in the text.
Our original submitted manuscript did include some FRET measurements taken at 42°C with WT hTRAP1 showing a dramatic increase in the rate of closure compared with 36°C, Figure 4B and Table 3. A 42°C closure rate of the Δstrap variant is also included in Figure 4D and Table 3.
Specific points:
1) The authors state that the kinetic barrier for closure is large and unusually sensitive to temperature changes. Examples should be included to allow for comparison.
We thank the reviewer for pointing this out and we have tried to better emphasize this point in the first section of the Results. hHsp90 has no change while yHsp90 and TRAP1 do have temperature sensitivity, however TRAP1 appears to be more extreme. By collecting a new series of Δstrap temperature data (Figure 4D), we can now calculate an Arrhenius activation energy for both the WT and Δstrap variants of TRAP1. Arrhenius fits are now included in Figure 4E and with modifications included in the main text. We have also included a quantification of the activation energy for Hsp90 homologs (yHsp90 and Grp94) in Figure 4–figure supplement 2, utilizing previously reported rates found in Frey et al 2007. Comparing our calculated activation energy as well as a previously reported value (Leskovar et al 2008), we find that the other homologs have significantly lower activation energies. These data clearly show that TRAP1 has unusually large response to temperature changes.
2) The kinetic data should include fits and resulting rate constants (and also which equation was used) to judge quality of the kinetic model.
Agreed. The kinetic data in the manuscript now all include a plot of the fit used to determine the rate constant. The equations are now included in the methods section as well.
3) If the measured conformational change is indeed rate limiting then its temperature dependence should be the same as that of the ATPase activity measured before. Is this indeed the case? How is the relationship of the shortened variant?
We have better highlighted in the text our observation of the difference in closure rate (measured by FRET) with AMPPNP and ATP, and the steady-state ATPase rates. We find that the closure rate measured with ATP better matches the ATPase rate measured for the fully labelled Inter FRET probe used in experiments shown in Figure 6D and in the same buffer and temperature conditions (see Methods). Though we have not measured the temperature dependence of closure with ATP at the full range of temperatures as AMPPNP, we do show that the closure rate is slower at 25 °C and that the fold difference in closure rate is greater between WT and Δstrap protein at 25 °C (Table 3). The difference between the ATP analogs suggests that the energetics differ which could shift (but not mitigate) the observed temperature dependence of closure depending on the analog used. Importantly, we point out that despite experimental differences that could arise due to cysteine removal, labelling, or usage of varying nucleotide, our observation of the unique temperature dependent closure (also supported by Leskovar et al 2008) and the role of the strap as a structural element responsible for regulating this observation remains constant across all experiments in our manuscript.
Our proposal that closure is rate limiting is supported by previous studies (Hessling et al 2009 and Leskovar et al 2008) as well as our measurements in Figures 4 and 6, which allow us to evaluate the rate of closure, re-opening and hydrolysis in matching conditions for our WT and Δstrap FRET probes. We were able to decouple the closure and hydrolysis steps by removing MgCl2 from the closure reactions in the presence of nucleotide and find that closure is much slower than hydrolysis, with both WT and Δstrap hTRAP1 (Figure 6D and 6E, expounded upon within our manuscript). These experiments also show that removal of the strap has the greatest impact on the closure rate, a significant but smaller effect on re-opening, and a minor effect on hydrolysis.
We have additionally included FRET experiments with the Inter FRET probes to monitor the rates of closure ± the strap (Figure 4D). From this data an Arrhenius plot of both the WT and Δstrap proteins has been added in Figure 4E. Calculating the difference in Ea between WT and Δstrap we assign the contribution of the strap to Ea at approximately 60% of the measured Ea for WT hTRAP1 (48.8 kcal/mol Ea for WT; 29 kcal/mol for Δstrap). These data are consistent with the steady-state SAXS and ATPase, and show that removal of the strap region lowers the energy barrier between apo and the closed state.
4) Figure 1: Do other Hsp90 isoforms also show a similar trend over a temperature range or is this special just for TRAP1?
This trend is not just specific for TRAP1 and we would like to point the reviewers to Leskovar et al 2008 and Krukenberg et al 2008. The sensitive temperature range and specific rates do vary dramatically among Hsp90 homologs, with TRAP1 displaying particularly heightened sensitivity (also see specific point 1 author response above). Regulation via the strap is the focus of our manuscript and the strap does not exist in the yHsp90 and bHsp90 homologs. In addition to TRAP1 and Grp94, the temperature sensitivity has also been recently reported for an Hsp90α alternative splice variant (Tripathi et al 2013) that importantly is imparted by a long N-terminal extension of ∼122 amino acids. We have made an effort to highlight these points in our discussion section.
5) Experiments in Figure 1 and 2 do not show kinetics, just a shift of equilibria.
We have changed the titles and Figure descriptions to better highlight these experiments as equilibrium experiments. These experiments initially suggested to us that TRAP1 might have a unique energy landscape, which we set out to elucidate the underlying mechanism of the phenomena.
6) EM images for delta strap should be included.
We have not collected EM images for Δstrap. After taking the initial EM images of the WT at various temperatures we moved to measure the % closed state by SAXS, which is a much more quantitative measure of conformational states and has previously be used to measure Hsp90 conformational equilibrium by our lab and others (Frey et al 2007).
7) In an Agard publication from 2008 (Southworth & Agard, Cell 2008) EM images of HtpG, yeast and human Hsp90 are shown in the apo state, with AMPPNP, and with ADP. The human Hsp90α is in an open conformation with AMPPNP at 37⁰C (according to them TRAP1 is fully closed at 37⁰C).
TRAP1 is the mitochondrial variant that shows noticeably different behavior from the cytosolic form of hHsp90 that was shown in the (Southworth & Agard, Cell 2008) manuscript. In this study we sought to bootstrap from our recent TRAP1 crystal structure (none are available for Hsp90α) to gain possible molecular insights into this difference. Cytosolic hHsp90 does not significantly close with AMPPNP at 37 °C suggesting a different energetic landscape and consequently a much lower ATPase activity, as highlighted in Southworth et al. It is important to note that although the energetics are different between Hsp90 homologs (likely due to different physiological environments, specific clients, and different requirements for co-chaperones) the underlying conformational states and mechanism of protein folding are conserved as also highlighted in Southworth et al.
8) Figure 2–figure supplement 1: Isn't this the same figure as Figure 2C just without the delta-strap?
Figure 2–figure supplement 1 (now Figure 2D) is meant to demonstrate that TRAP1 will remain closed even after cooling the sample back to 20°C for two hours. By this observation TRAP1 is kinetically trapped in the closed state after heating and will remain closed even when cooled. These data support a large temperature dependent barrier to the closed state that is overcome upon increasing temperature and a stable closed state once the transition has occurred rather than a pronounced temperature dependence of the equilibrium states. As mentioned above, the supplemental figure has now been combined with Figure 2, panel D, in an attempt to make this less confusing.
9) Is it known that the trans contacts that strap forms in the closed conformation stabilize the closed conformation?
From our previously published crystal structure of TRAP1 (Lavery et al 2014), cocrystallized with AMPPNP, we know that the strap makes substantial contacts with the trans-NTD while in the closed conformation, suggesting a role in stabilization. However, this is most directly shown by our new observation that deleting the strap accelerates reopening (Figure 6A).
10) Figure 4A: For the NTD:MD rotation, the FRET probe does not show a significant change in the FRET signal.
The change in FRET signal with the NTD:MD rotation probe is on par with previously published results using the same probes but with bHsp90 (Street et al, 2011). The measurements are quite reliable even though the delta for this set of probe positions is less than that for the cross protomer set.
11) Figure 4B: Why was saturation not reached for the 42⁰C sample?
It does. We have now included the full dataset in Figure 4B to better depict saturation at 42°C.
12) Data for the dimer closure FRET construct should be included and it would be important to see how delta-strap acts in the FRET assay at different temperatures to compare with the SAXS data.
We now show temperature dependent closure as measured with FRET using both the NTD rotation and dimer closure probes (Figure 4B). We observe that the dimer closure probes (Inter FRET) display comparable temperature dependent closure as the NTD rotation probe (Intra FRET). Most directly, we have now included a new temperature dependent closure series for the Δstrap variant (Figure 4D).
These measurements show a dramatic loss of temperature dependent closure and quantification of the activation energy difference (Arrhenius plot using WT and Δstrap FRET data) shows that the strap contributes over half of the WT Ea (Figure 4E).
The FRET data is in good agreement with the SAXS data, which shows an increase in closed state at higher temperatures measured after 1 hour at the respective temperature. By estimating the % closed state at 1 hour from the FRET data (Author response image 1) we get 9%, 42%, 66%, 90% and 87% (compared to 2%, 31%, 41%, 74% and 84% at the respective temperatures, Table 1). Considering the 1 hour time point for the Δstrap, the reaction has reached completion according to our FRET and SAXS measurements in Figure 3B and 4C/D, respectively. Although our normalized FRET data is only an estimate of the percent closed state molecules and shouldn’t be taken as a quantitate number, estimated percent closed state compared to the SAXS data show the same trend.
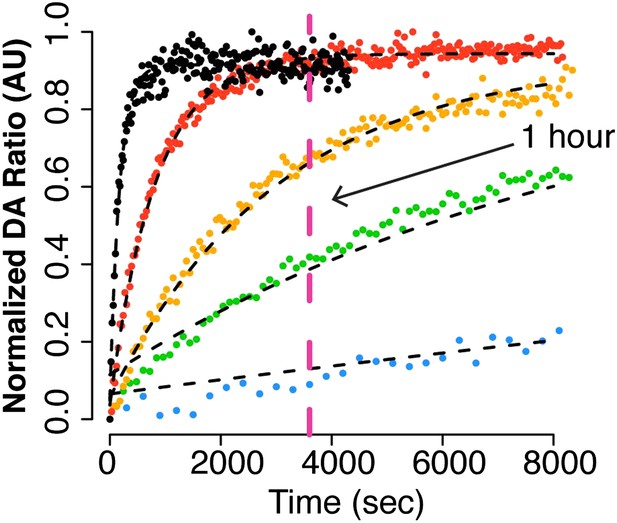
Adapted from Figure 4B (Intra FRET probe data set).
13) Why was the double strap mutant too unstable for NTD rotation FRET and not for inter-protomer FRET? Isn't it the same construct just with a different Cys label?
No, it is a different construct having two incorporated cysteine’s (S133C.E407C), within one protomer, whereas the inter-protomer FRET uses only 1 Cys per protomer (either E407C or E140C). We do not have a detailed explanation for why removing the strap in combination with the 2 Cys mutations in one protomer with the NTD rotation FRET pair destabilizes the protein. During purification of the Δstrap NTD FRET protein most was lost to cleavage products even when protease inhibitors were included.
The small amount of full-length protein that was purified did not appear unstable during experimental measurements, but given the unusual behavior during purification we did not have enough confidence in our measurements to report quantitative rates and draw conclusions from these measurements.
14) Figure 5A: The legend says that in the apo state the lid probe is in equilibrium between mobile and immobile states. In the Results section it is stated that the apo form is predominantly immobile.
We have reworded the legend to state that the apo state is in equilibrium between mobile and immobile as measured with EPR. After addition of AMPPNP there is a substantial change such that the mobile population dominates.
15) According to the authors, deleting strap compromises the stability of the closed structure and hence enhances the reopening rate and shifts the equilibrium towards the open state. If deleting strap shifts the equilibrium towards the open state, why is the delta-strap construct predominantly closed?
In this context, the authors mention that the effect of deleting the strap on the opening of the NTD interface is smaller than the effect on the kinetic barrier corresponding to the release of strap from the apo state. The respective numbers seem to be missing from Table 3.
The respective numbers that should be considered are listed in Table 3 under the Kclose and Kreopen heading for the CysFree hTRAP1 (E140C/E407C) and the Δstrap double (E140C/E407C) constructs. By taking the ratio of the rates, Kclose has a 16-fold difference while Kreopen has an 8-fold difference. This is further described in the main text in the FRET based “Dissecting further regulatory functions of the NTD-strap” section of the Results section in this manuscript. Thus deleting the strap destabilizes the open state more than it destabilizes the closed state. However, the overall equilibrium is still in favor of the open state in the absence of ATP.
16) Are the ATPase activities of the cys-free version of hTRAP1 and zebrafish TRAP1 mutant identical to the respective wild type proteins?
No. The cys-free versions of hTRAP1 and zTRAP1 are faster than WT in ATPase rates by 1.5–2 fold. This is presented in our previous manuscript (Lavery et al 2014). The molecular basis for the increased ATPase in the Cysteine Free TRAP1 constructs is unknown; however, all of our measurements are done by comparing WT to mutant (Δstrap, or strap point mutants) in matching conditions. We are interested and drawing conclusions from the fold change between mutant protein and the respective WT protein. As mentioned above we also point out that despite any differences that come about due to cysteine removal, labelling or nucleotide used in the experiments, our observation of the unique temperature dependent closure and the role of the strap as the structural piece responsible for these observation remains constant across all experiments in our manuscript.
17) Figure 6. The legend says delta strap is ∼7 fold faster; text says ∼8 fold faster.
Thank you for pointing this out. This was a mistake and the difference has now been corrected. The legend now matches the text with “8-fold”.
18) Figure 6C: a trace showing that in presence of ATP without Mg2+, there is no ATPase activity should be added. Not adding Mg2+ is not necessarily equivalent to not having (ambient) Mg2+ present in the solution. The ATP induced changes in FRET signals should be measured also in the presence of EDTA.
The coupled NADH reaction used to measure ATPase rates is dependent on
Mg2+ so we are unable to do the comparable ATPase experiment in absence of Mg2+.
19) The authors mention that in the absence of Mg2+, ATP and AMPPNP show pronounced differences in kinetics of FRET signal, the difference with/without Mg2+ should be even more pronounced, that is the kinetics of o/c may be substantially faster in the presence of Mg2+. Measuring the FRET kinetics upon addition of Mg-ATP and Mg-AMPPNP is crucial to show that ATP induced closing kinetics in absence of Mg2+ are indeed representative for the ATPase cycle.
While the closure rate with ATP-Mg2+ would be ideal to compare to AMPPNPMg2+ (all FRET data in Figure 4 is done with AMPPNP-Mg2+), we are unable to measure closure as hydrolysis is faster than closure, hence the closed state does not build up in the presence of Mg2+. Rather, we measure a FRET signal that is always equivalent to Apo (no change from time zero). This was also seen in FRET studies with yeast Hsp90 (yHsp90) in Hessling et al, 2009. We noted the differences in the manuscript as an observation, however we feel uncovering the molecular reason for variability of rates and affinity between the two ATP analogs is beyond the scope of this manuscript. While interesting, here our focus is on the changes in these rates that are connected with the strap.
20) How does one know that Mg2+ can actually bind to the closed form; and that a re-open is not necessary for this to happen?
ATP hydrolysis upon addition of Mg2+ is relatively fast compared to the reopening rate 0.463 vs. 0.002 for WT protein or 13.3 vs. 0.016 for Δstrap protein. This strongly suggests that Mg2+ can bind to the closed state.
Reviewer #2
In this study, Partridge and colleagues investigate the role of the N-terminal extension (“strap”) of TRAP1, the mitochondrial Hsp90 isoform, which in the previously solved crystal structure of the TRAP1 dimer wraps around the N-domain of the opposite protomer. They characterized the effects of the strap on the dimer closure kinetics, the rotation of the N-domain relative to the M-domain, the ATP hydrolysis and movement of the ATP lid (N-domain) using negative stain electron microscopy (EM), small angle x-ray scattering (SAXS), fluorescence resonance energy transfer (FRET) and electron paramagnetic resonance (EPR) measurements. They demonstrate that the strap region is responsible for a temperature-dependent increase in the rate of TRAP1 closure, as well as the increase in the ATPase activity.
The data presented here is convincing and interesting. The physiological relevance of the observed phenomenon is not so clear since the major effects for human TRAP1 happen at temperatures below 37⁰C. Nevertheless, the story could be published after the authors addressed the raised issues.
We thank reviewer for the suggested experiments to strengthen our manuscript. We have attempted to address this concern, echoed by both the editor and Reviewer 1, by including new EM and SAXS data recorded above 37°C. It is clear from the SAXS data that the rate of closure continues to increase as temperatures increase above 37 °C. This is also clear from the FRET closure data (Figure 4B) which shows a significant increase in closure rate at 42°C. A more detailed description of the additional data and references has been described in the comments above for Reviewer 1.
Major comments
1) Figure 1 shows negative stain EM images of TRAP1 in the presence of AMPPNP pre-incubated at different temperatures. Few representative samples are picked from each grid to show the transition from open to closed conformation with the increase in temperature. The authors should quantify the open and closed structures from a representative square of the electron micrograph.
We very much agree that quantification of the %closed verses Apo state at each temperature is quite important and must be included. While we have done this in the past (Southworth, 2008), in practice, this is a somewhat painful and laborious procedure to do rigorously and instead here we chose to quantify the %closed state by SAXS. This is a significantly more quantitative assay for measuring equilibrium of states and has previously be used to measure Hsp90 conformational equilibrium by our lab and others (Frey et al, 2007). The quantification of %closed state as measured by SAXS can be seen in Figure 3 and Table 1.
2) Using SAXS the authors measured AMPPNP-induced transition of human and zebrafish TRAP1 to the closed conformation between 20 and 36⁰C (Figure 2). To determine the physiological relevance of their observations the authors could have measured the AMPPNP induced transition of human TRAP1 at 37 to 42⁰C. Does human TRAP1 become more active at heat shock temperatures?
We now include SAXS data above 37°C and up to 43°C. Interestingly, TRAP1 from humans seems to have the largest jump in activity around 36-40°C, just where it might be most physiologically relevant. Analogously, the largest jump in % closed for TRAP1 from zebrafish seems to be in the 20-30°C range. Both species loose temperature sensitivity without the strap. However both species do show a jump in activity in Δstrap when going up to 43°C.
3) Figure 4: The authors investigate AMPPNP-induced changes in TRAP1 conformation using FRET. Control experiments with only the acceptor dye need to be shown especially as the changes in fluorescence for the NTD:MD rotation seems to be very small. The temperature at which the experiment of Figure 4(A) has been performed should be mentioned in the figure legend.
Addition of nucleotide showed an appropriate anti-correlation of the donor/acceptor FRET signals and the anticipated direction of the change in FRET (increase in FRET for Inter FRET probe, and decrease in FRET for Intra FRET probe). The steady-state scans shown in Figure 4A were taken after the closure reaction was complete. Here, to avoid any temperature dependence on the dyes, etc., closure was induced by heat shock for 1hr and then the samples actually measured at room temperature. The temperature at which the scans were done has been added to the figure legend.
4) The dimer closure FRET experiments have been performed only at 30⁰C. As the paper deals with effect of temperature on ATPase rates of TRAP1, it would be very important to see the change in rate of dimer closure at different temperatures. It would also be interesting whether the rates of NTD-MD-rotation and dimer closure are similar to each other.
Because NTD-MD rotation is tightly coupled to closure, the closure rates measured by either probe set are similar; we had initially hoped to be able to tease apart these individual steps, but in practice, they seem kinetically inseparable (differences due to probe locations). We have now included the temperature dependent closure experiment (Figure 4B) and note that both probe sets are comparable in matching experiments. We chose to do the dimer closure FRET experiment +/- strap at 30 °C with SAXS, EM and ATPase as this is the temperature where we see the largest differences between WT and Δstrap in the temperature range assayed. Additionally, we have now included a temperature dependent closure series for the Δstrap variant (Figure 4D). As predicted, comparing the fold changes of closure rates (Table 3) at each temperature we see the largest fold change at lower temperatures (23 °C: 24-fold, 30 °C: 16-fold, 32 °C: 12-fold, 36 °C: 7-fold, and 42 °C: 3-fold). The clear impact of the strap is revealed from a comparison of the Arrhenius plots in Figure 4E.
5) In Table 2 the authors write that the steady state ATPase rate for human TRAP1 was 0.463 min-1 and in Table 3 the write that the closing rate for human TRAP1 at 30⁰C was 0.02 min-1. These values do not fit together and contradict the claims of the authors. The authors should indicate at which temperature the ATPase assays were performed and correlate the closing rate with the ATP hydrolysis rates to substantiate their claims. Maybe the authors will have to measure the closing rate upon addition of ATP instead of AMPPNP. This seems possible since omission of Mg prevents hydrolysis as the authors have shown.
We do recognize the significant difference between closure rates determined with AMPPNP and the ATPase measurements taken with ATP. For reference the best comparison should be done with the same protein and buffer conditions used in the FRET experiments with ATP as indicated in Table 2 and Table 3 (0.79 min-1 ATPase- Table 2 vs. 0.42 min-1 closure- Table 3). There is a strong nucleotide dependence on the closure rates, and the closed state never builds up with ATP plus Mg2+ as hydrolysis is faster than closure. However, for this manuscript we would like to focus on a more broadened and molecular aspect of the strap mediating temperature sensitivity to regulate closure and thereby activity of TRAP1 in both zebrafish and human. We have done matched experiments for WT and Δstrap for each assay and have drawn our conclusions from the differences between these matched experiments.
We show the temperature dependence of the closure reaction and the loss of temperature dependence upon deletion of the strap is robustly observed between multiple biophysical and biochemical of experiments. We ask that the reviewers please excuse our reluctance to dive even further into molecular differences between ATP analogs as we feel this is beyond the scope of our study.
https://doi.org/10.7554/eLife.03487.021