Evolution: The enigmatic xenopsins
How did animals develop the ability to detect light and see? The process began when a transmembrane protein evolved into an efficient light sensor called opsin (Feuda et al., 2012). Subsequently, a stunning diversity of opsins emerged in various animal phyla that set the stage for the evolution of eyes.
The two most-studied opsins are r-opsins, which are are found in rhabdomeric photoreceptor cells, and c-opsins, which are found in ciliary photoreceptor cells (Arendt et al., 2004). The r-opsins are commonly stored at the top of rhabdomeric cells in the folded cell membrane, called the rhabdom, which became the major light sensor in invertebrate eyes. In contrast, the c-opsins are transported into the cilium of ciliary photoreceptor cells, where the membrane is likewise folded to expand the light-sensitive surface. Ciliary photoreceptors are the main constituent of vertebrate eyes.
Besides the r- and c-opsins, which are well characterized, other opsins are now becoming the focus of attention. For example, tetraopsins are co-expressed with r-opsins in the eyes of segmented worms (Gühmann et al., 2015), and cnidopsins locate to the light-sensitive cilia of jellyfish eyes (Bielecki et al., 2014). The newest addition to the zoo of opsins, however, is an enigmatic group called the xenopsins (Ramirez et al., 2016). Now, in eLife, Harald Hausen and colleagues at the University of Bergen – including Oliver Vöcking, who is also at the University of Pittsburgh, as first author – report the results of a study that reveals more about how opsins evolved (Vöcking et al., 2017).
Vöcking et al. first present a molecular phylogeny, based on RNA sequence data, that distinguishes a total of ten distinct opsin families (Figure 1). Complementing this analysis, they then plot information about the introns (which are not present in the sequenced RNA molecules) of all available opsin genes, so that for the first time we can exhaustively compare how the opsin families are evolutionarily related with the structure of those genes. This analysis reveals distinctive intron patterns for the majority of the opsin families. Moreover, and rather strikingly, some introns are shared between families, suggesting that these families are more closely related: for example, the ‘anthozoan opsins II’ (only found in the polyps of anthozoans such as sea anemones and corals) and the ctenopsins (which are found in ctenophores, also known as comb jellies; Schnitzler et al., 2012) share one intron with each other and two introns each with c-opsins. This is in line with the results of the molecular phylogeny that likewise links the three families and indicates that the ctenopsins and the anthozoan opsins represent offshoots of the c-opsin branch. This is a huge step forward in our understanding of how c-opsin and ciliary photoreceptors evolved.
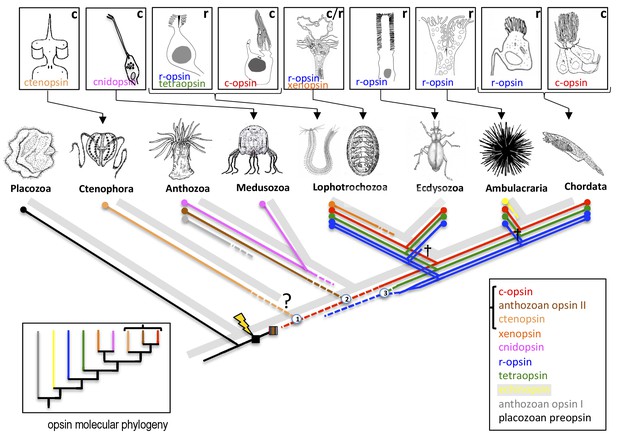
The evolution of opsins and photoreceptor cells.
The animal evolutionary tree (light grey) shows the relationships between a number of different animal phyla; the question mark indicates the current uncertainty about when the ctenophores emerged. Different phyla have evolved their own photoreceptors (top; ‘r’ denotes rhabdomeric photoreceptors, ‘c’ denotes ciliary photoreceptors) that each use specific opsins to detect light (named in the panel of its respective photoreceptor). To investigate when these opsins and photoreceptors evolved, Vöcking et al. determined the molecular phylogeny of ten families of opsins (bottom left; colored lines correspond with the colored text in the bottom right box). Further confirmation that the bracketed opsins are closely related came from an analysis that shows that their genes share introns. Placing the opsin molecular phylogeny (colored lines in main image) on top of the animal evolutionary tree (light grey) suggests that if both are correct, certain opsins (such as the xenopsins) must have been independently lost from multiple phyla during evolution. Opsins first evolved after the divergence of the Placozoa (lightning bolt) and then went through an early period of diversification (striped box). Numbers in circles mark opsin family relationships that are supported by evidence from shared introns. Crosses indicate the loss of an opsin in a given lineage. Dashed lines indicate opsin relationships inferred from the intron comparison.
The position of the xenopsins, however, remains enigmatic. While the molecular phylogeny suggests they are closely related to the cnidopsins, the intron comparison reveals one shared intron with the r-opsins, which is insufficient to draw any conclusion. Even more puzzling, despite their apparent deep rooting in the opsin molecular phylogeny, the xenopsins are found only in the Lophotrochozoans (a superphylum that includes mollusks). This would imply that xenopsins have frequently been lost throughout animal evolution.
Complicating matters further, Vöcking et al. investigated the expression of xenopsin in the chiton, a marine mollusk. They found that xenopsin is co-expressed with r-opsins in the rhabdomeric photoreceptor cells of the larvae. They also found that these cells bear a cilium and express genes that help to localize opsins to cilia. Thus an r-opsin may be found in the rhabdom and a xenopsin in the cilium of the same photoreceptor, giving these cells a somewhat chimeric appearance.
To validate and explore this finding further, it will be necessary to test whether xenopsin and possibly other opsins indeed localize to the cilium and to determine the phototransduction cascades that the opsins trigger when they detect light. Only then will we understand how the diversity of the new opsins fits into the classical picture of rhabdomeric and ciliary photoreceptors.
Another challenge emerges from the molecular phylogeny. As it stands, this suggests that all opsin families outside of the c-opsin branch existed very early in animal evolution, even before the birth of the ctenophores (whose phylogenetic position is currently debated; see Simion et al., 2017). This is implausible for the xenopsins, especially so for the anthozoan and echinoderm opsins that only exist in one phylum, because it means they must have been lost in all other phyla. Of course, this paradox might also reflect the difficulty of using molecular phylogenies to resolve molecular interrelationships across animal phyla that separated more than 600 million years ago.
References
-
The last common ancestor of most bilaterian animals possessed at least nine opsinsGenome Biology and Evolution 8:3640–3652.https://doi.org/10.1093/gbe/evw248
Article and author information
Author details
Publication history
- Version of Record published: October 19, 2017 (version 1)
Copyright
© 2017, Arendt
This article is distributed under the terms of the Creative Commons Attribution License, which permits unrestricted use and redistribution provided that the original author and source are credited.
Metrics
-
- 2,180
- views
-
- 288
- downloads
-
- 13
- citations
Views, downloads and citations are aggregated across all versions of this paper published by eLife.
Download links
Downloads (link to download the article as PDF)
Open citations (links to open the citations from this article in various online reference manager services)
Cite this article (links to download the citations from this article in formats compatible with various reference manager tools)
Further reading
-
- Genetics and Genomics
Uncovering the regulators of cellular aging will unravel the complexity of aging biology and identify potential therapeutic interventions to delay the onset and progress of chronic, aging-related diseases. In this work, we systematically compared genesets involved in regulating the lifespan of Saccharomyces cerevisiae (a powerful model organism to study the cellular aging of humans) and those with expression changes under rapamycin treatment. Among the functionally uncharacterized genes in the overlap set, YBR238C stood out as the only one downregulated by rapamycin and with an increased chronological and replicative lifespan upon deletion. We show that YBR238C and its paralog RMD9 oppositely affect mitochondria and aging. YBR238C deletion increases the cellular lifespan by enhancing mitochondrial function. Its overexpression accelerates cellular aging via mitochondrial dysfunction. We find that the phenotypic effect of YBR238C is largely explained by HAP4- and RMD9-dependent mechanisms. Furthermore, we find that genetic- or chemical-based induction of mitochondrial dysfunction increases TORC1 (Target of Rapamycin Complex 1) activity that, subsequently, accelerates cellular aging. Notably, TORC1 inhibition by rapamycin (or deletion of YBR238C) improves the shortened lifespan under these mitochondrial dysfunction conditions in yeast and human cells. The growth of mutant cells (a proxy of TORC1 activity) with enhanced mitochondrial function is sensitive to rapamycin whereas the growth of defective mitochondrial mutants is largely resistant to rapamycin compared to wild type. Our findings demonstrate a feedback loop between TORC1 and mitochondria (the TORC1–MItochondria–TORC1 (TOMITO) signaling process) that regulates cellular aging processes. Hereby, YBR238C is an effector of TORC1 modulating mitochondrial function.
-
- Genetics and Genomics
- Neuroscience
Adenine phosphoribosyltransferase (APRT) and hypoxanthine-guanine phosphoribosyltransferase (HGPRT) are two structurally related enzymes involved in purine recycling in humans. Inherited mutations that suppress HGPRT activity are associated with Lesch–Nyhan disease (LND), a rare X-linked metabolic and neurological disorder in children, characterized by hyperuricemia, dystonia, and compulsive self-injury. To date, no treatment is available for these neurological defects and no animal model recapitulates all symptoms of LND patients. Here, we studied LND-related mechanisms in the fruit fly. By combining enzymatic assays and phylogenetic analysis, we confirm that no HGPRT activity is expressed in Drosophila melanogaster, making the APRT homolog (Aprt) the only purine-recycling enzyme in this organism. Whereas APRT deficiency does not trigger neurological defects in humans, we observed that Drosophila Aprt mutants show both metabolic and neurobehavioral disturbances, including increased uric acid levels, locomotor impairments, sleep alterations, seizure-like behavior, reduced lifespan, and reduction of adenosine signaling and content. Locomotor defects could be rescued by Aprt re-expression in neurons and reproduced by knocking down Aprt selectively in the protocerebral anterior medial (PAM) dopaminergic neurons, the mushroom bodies, or glia subsets. Ingestion of allopurinol rescued uric acid levels in Aprt-deficient mutants but not neurological defects, as is the case in LND patients, while feeding adenosine or N6-methyladenosine (m6A) during development fully rescued the epileptic behavior. Intriguingly, pan-neuronal expression of an LND-associated mutant form of human HGPRT (I42T), but not the wild-type enzyme, resulted in early locomotor defects and seizure in flies, similar to Aprt deficiency. Overall, our results suggest that Drosophila could be used in different ways to better understand LND and seek a cure for this dramatic disease.