Emerging Technology: Concerning RNA-guided gene drives for the alteration of wild populations
Figures
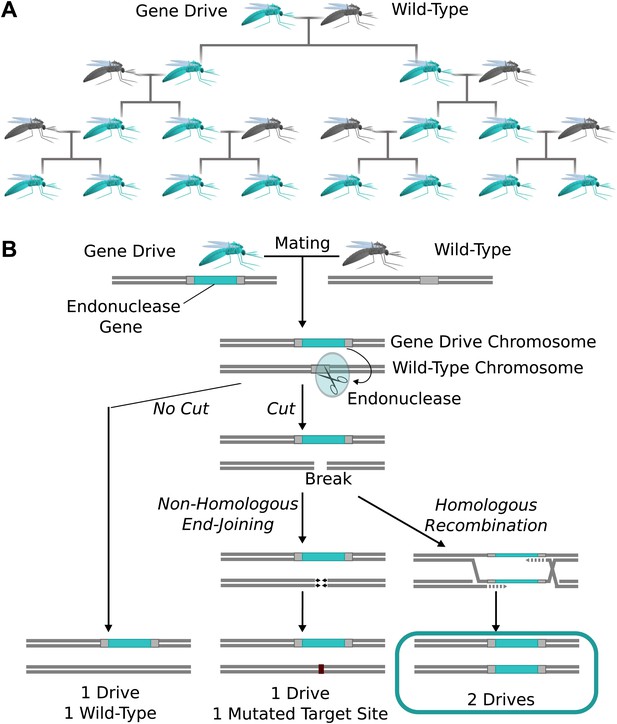
The spread of endonuclease gene drives.
(A) When an organism carrying an endonuclease gene drive (blue) mates with a wild-type organism (grey), the gene drive is preferentially inherited by all offspring. This can enable the drive to spread until it is present in all members of the population–even if it is mildly deleterious to the organism. (B) Endonuclease gene drives are preferentially inherited because the endonuclease cuts the homologous wild-type chromosome. When the cell repairs the break using homologous recombination, it must use the gene drive chromosome as a repair template, thereby copying the drive onto the wild-type chromosome. If the endonuclease fails to cut or the cell uses the competing non-homologous end-joining repair pathway, the drive is not copied, so efficient gene drives must reliably cut when homology-directed repair is most likely.
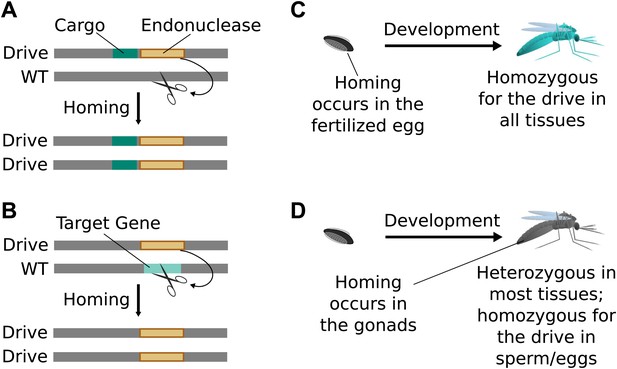
Consequences and timing of gene drive replication.
(A) Gene drives can carry other genes with them as cargo. For example, a transgene that blocks malaria transmission could be driven through wild mosquito populations. There is no selection to maintain the function of a cargo gene. (B) Gene drives can disrupt or replace other genes. For example, a drive might replace a mosquito gene important for malaria transmission. Because it cannot spread without disrupting the target gene, this strategy is evolutionarily stable. (C) If homing occurs in the zygote or early embryo, all organisms that carry the drive will be homozygous in all of their tissues. (D) If homing occurs in the late germline cells that contribute to sperm or eggs, the offspring will remain heterozygous in most tissues and avoid the consequences of drive-induced disruptions.
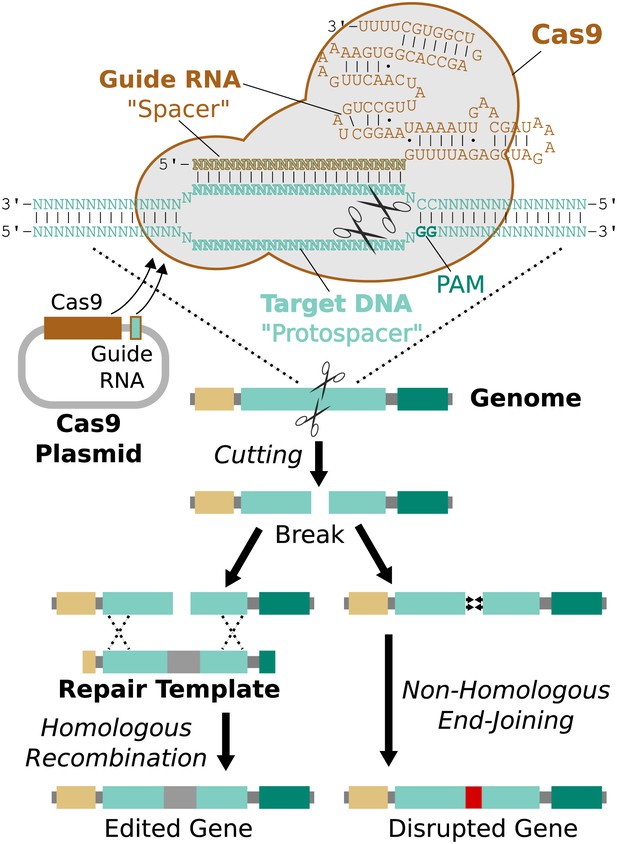
RNA-guided genome editing via Cas9.
The Cas9 nuclease protein and guide RNA must first be delivered into the target cell. This is often accomplished by transfecting DNA expression plasmids, but delivering RNA is also effective. The guide RNA directs Cas9 to bind target DNA ‘protospacer’ sequences that match the ‘spacer’ sequence within the guide RNA. Protospacers must be flanked by an appropriate protospacer-adjacent motif (PAM), which is NGG for the most commonly used Cas9 protein (Jinek et al., 2012). If the spacer and protospacer are identical or have only a few mismatches at the 5′ end of the spacer, Cas9 will cut both strands of DNA, creating a blunt-ended double-strand break. If supplied with a repair template containing the desired changes and homology to the sequences on either side of the break, the cell may use homologous recombination to repair the break by incorporating the repair template into the chromosome. Otherwise, the break will be repaired by non-homologous end-joining, resulting in gene disruption. Cas9 cutting is efficient enough to alter both chromosomes at the same time and/or to edit multiple genes at once (Li et al., 2013; Wang et al., 2013a). If the cell being edited is a germline cell that gives rise to eggs or sperm, the changes can be inherited by future generations.
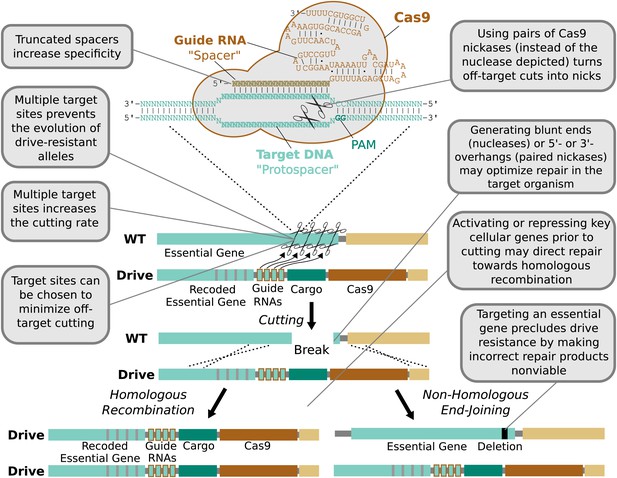
Technical advantages of RNA-guided gene drives.
Clockwise from lower left: The targeting flexibility of Cas9 permits the exclusive selection of target sequences with few potential off-targets in the genome. Targeting multiple sites increases the cutting frequency and hinders the evolution of drive resistant alleles, which must accumulate mutations at all of the sites. The Cas9 nuclease is can be quite specific in the sequences that it targets; fruit flies do not exhibit notable fertility or fitness defects resulting from off-target cutting when both Cas9 nuclease and guide RNAs are expressed in the germline (Kondo and Ueda, 2013). Choosing target sites with few or no close relatives in the genome, using truncated guide RNAs (Fu et al., 2014), employing paired Cas9 nickases (Mali et al., 2013a) instead of nucleases, or utilizing Cas9-FokI fusion proteins (Guilinger et al., 2014; Tsai et al., 2014) can further increase specificity. Several of these strategies can reduce the off-target mutation rate to borderline undetectable levels (Fu et al., 2014; Guilinger et al., 2014; Tsai et al., 2014). The frequency at which the drive is correctly copied might be increased by using Cas9 as a transcriptional regulator to activate HR genes and repress NHEJ genes (Gilbert et al., 2013; Mali et al., 2013a) (Figure 4—figure supplement 1). By choosing target sites within an essential gene, any non-homologous end-joining event that deletes all of the target sites will cause lethality rather than creating a drive-resistant allele, further increasing the evolutionary robustness of the RNA-guided gene drive. Other options include using distinct promoters and guide RNAs to avoid repetitiveness and increase stability (Figure 4—figure supplement 2) or employing newly characterized, engineered, or evolved Cas9 variants with improved properties (Esvelt et al., 2011; Mali et al., 2013b). These optimization strategies have also been summarized in tabular form with additional details (Figure 4—figure supplement 3).
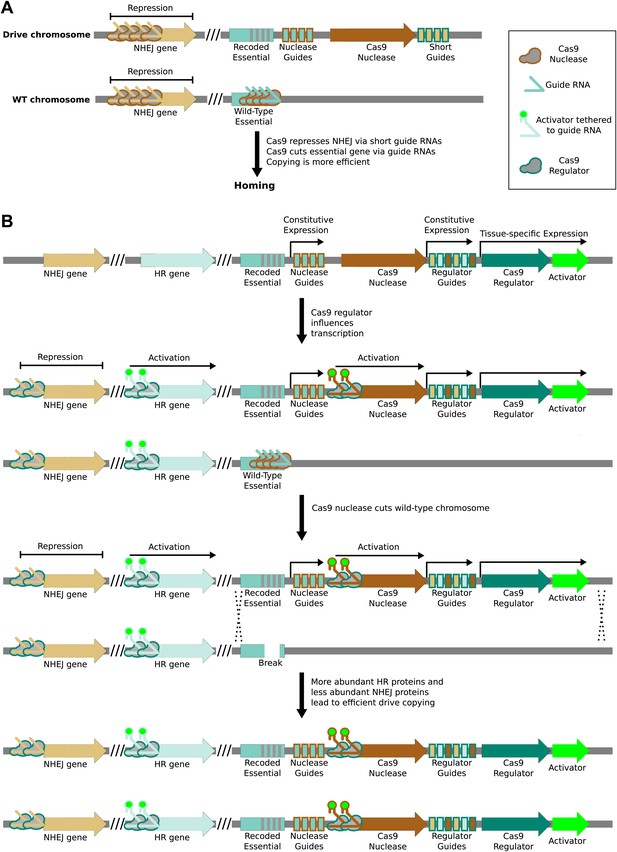
Enhancing drive copying by regulating endogenous genes.
(A) Very short guide RNAs direct nuclease-active Cas9 to bind but not cut the corresponding protospacer (Sternberg et al., 2014). When bound near the promoter of a gene, Cas9 has been shown to repress transcription (Gilbert et al., 2013). Using the drive nuclease to repress key genes required for the non-homologous end-joining (NHEJ) pathway may increase the rate at which the drive is copied by boosting the effective rate of the competing homologous recombination (HR) pathway. (B) NHEJ genes could be repressed and HR genes activated in advance of cutting by employing an orthogonal nuclease-null Cas9 protein as a transcriptional regulator. The regulator would be expressed in the desired germline stage using an appropriate tissue-specific promoter and repress NHEJ genes by simple binding. HR genes could be simultaneously activated using guide RNAs featuring additional 3′ hairpins that bind a transcriptional activator expressed separately (Mali et al., 2013a), or by using a separate orthogonal Cas9. To ensure that modulation of repair pathways is coincident with cutting, the regulator might similarly activate transcription of the drive nuclease. Regulation will be evolutionarily stable during the lifetime of the drive if the drive nuclease requires the regulator for proper expression.
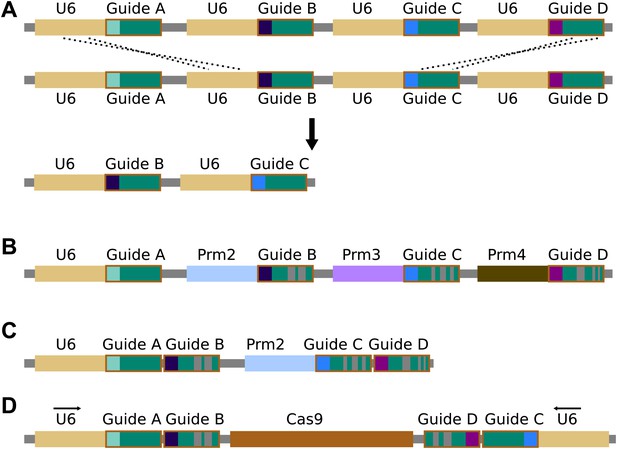
Repetitiveness and evolutionary stability of multiple guide RNAs.
(A) Homologous recombination between repetitive DNA sequences can lead to instability. Attempts to build zinc-finger and TALEN gene drives demonstrated that repeated components are severely unstable during drive copying, possibly due to the single-strand annealing pathway (Simoni et al., 2014). While the cas9 gene itself has no repeats, recombination between guide RNA cassettes or guide RNA promoters, most commonly the U6 promoter, is a possibility. (B) Using multiple guide RNAs with differing sequences that are known to retain function could reduce homology and thereby prevent instability. The length of the hairpin created by fusing the bacterial tracrRNA and crRNA can be varied by more than a dozen bases with equivalent activity (Esvelt et al., 2013), while tracrRNA equivalents from closely related bacteria can be substituted (Fonfara et al., 2013). Similar alterations could presumably be discovered through experimentation (Nishimasu et al., 2014). Together, these allow the creation of many different functional guide RNA sequences that do not share more than a few dozen bases of homology. Homology in the promoters used for guide RNA expression might be similarly reduced by employing different Polymerase III promoters; several of which are typically present in each species (Friedland et al., 2013; Dickinson et al., 2013; Nissim et al., 1016). (C) To reduce the requirement for multiple promoters, studies have shown that more than one guide RNAs can be expressed from a single promoter in various ways (Tsai et al., 2014; Nissim et al., 1016). If cutting is already highly efficient, strategies that utilize RNA polymerase II promoters that typically exhibit reduced cutting efficiency may represent alternatives (Nissim et al., 1016). (D) The total number of guides and promoters can be doubled without notably decreasing stability by arranging them in two inverted groups on opposite sides of the drive. Finally, guide RNA engineering and improvement is an extremely active area of research; if current solutions prove to be inadequate, it is likely that alternatives will soon become available.
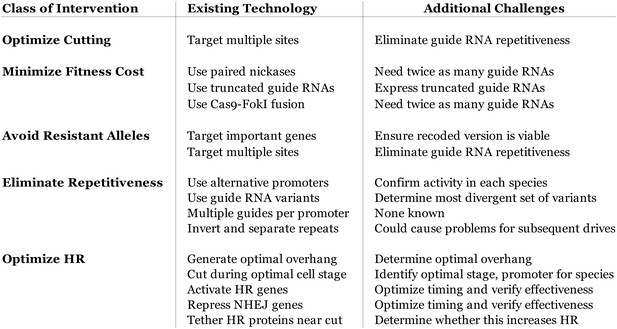
Table of known technological advances that might be adapted to optimize gene drive efficiency.
Off-target fitness cost, Cas9 cutting efficacy, and correct drive copying by homologous recombination (HR) are the primary determinants of drive fitness. The ideal standard gene drive confers an effective fitness benefit of 100%, as it is transmitted to twice as many progeny with no fitness cost. The fitness of suppression drives is more complicated due to species-specific density and resource-dependence and mating dynamics and must be considered on a case-by-case basis. The most challenging problem at the molecular scale concerns the highly variable rates of homologous recombination (HR) relative to non-homologous end-joining (NHEJ) in different species, cell types, and developmental stages. Cas9 cutting should ideally occur during a stage featuring efficient HR and minimal NHEJ to maximize fitness. Within the germline, homologous recombination rates are normally highest in the oocyte because NHEJ is nearly absent in that cell type. As development progresses, the incidence of NHEJ rises sharply even if the HR machinery remains active. For this reason, we anticipate that maternally transmitted drives that both cut and are copied into the paternal chromosome in the zygote will be among the most efficient. Paternally transmitted drives cannot cut the maternal chromosome until they are expressed, which occurs at different times in different species. Those species that initiate transcription comparatively early, such as mice, are likely to be more amenable to gene drives than those that begin late, such as Drosophila. However, this does not necessarily imply that HR occurs at low efficiency in Drosophila embryos; injecting Cas9 and guide RNA-encoding plasmids along with a template to be copied yielded correct insertions in 13/16 embryos (Gratz et al., 2013). In contrast, injecting Cas9 and guide RNAs targeting two genes along with templates for repair into mouse embryos yielded 7/10 pups with the first insertion and 8/10 with the second insertion, with six of those having both; all other mice utilized NHEJ. We suspect that while drive copying rates will be difficult to predict in advance in a given species, any drive constructed with a housekeeping, viral, or strong germline promoter is likely to function reasonably well due to the combination of highly efficient maternal copying and moderate paternal copying in the zygote and early embryo. Germline copying can also occur later in development for standard drives. The difference between achiasmate species with low HR rates during meiosis, at least in males, and chiasmate species is likely to be crucial. The difference is clearly demonstrated by comparing the Anopheles drive, which exhibited 97% HR and a fitness benefit over 50% (25% given its restriction to males) (Windbichler et al., 2011), to the equivalent Drosophila drive, which exhibited 71% HR and an effective fitness benefit of 32% (54% homing–22% NHEJ; a 16% benefit given its restriction to males) only after extensive optimization (Chan et al., 2013b). The latter was improved from an initial construct demonstrating only 35% HR (Chan et al., 2011), which is insufficient to generate a fitness benefit if targeting an essential gene and will generate more resistant alleles than copies of the drive if not. Limiting expression to the late germline may be very effective, but early germline or especially oocytic expression may be superior depending on the species. Finally, it may be possible to repress genes required for the competing non-homologous end-joining pathway using Cas9 in order to boost the effective homologous recombination rate in a particular germline stage (Figure 4—figure supplement 1). Genes responsible for homologous recombination might be activated in a similar manner. The main question is whether the resulting changes in protein abundance will occur quickly enough to influence drive copying.
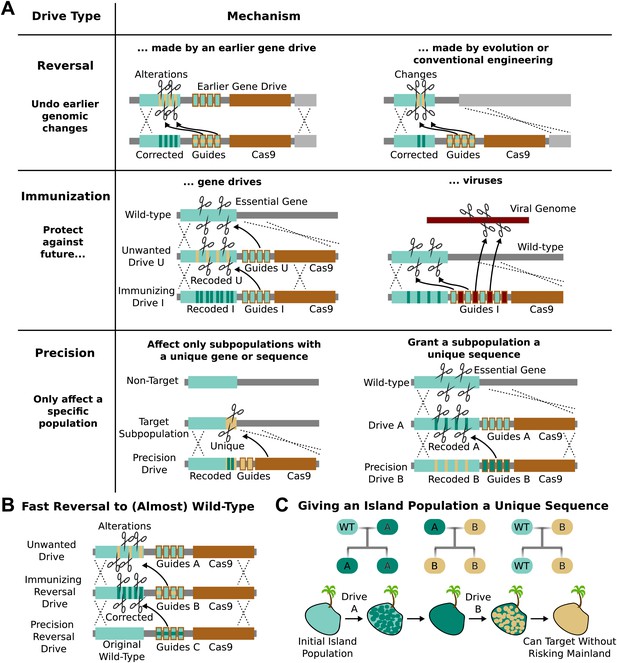
Methods of reversing, preventing, and controlling the spread and effects of gene drives.
(A) Reversal drives could correct or reverse genomic alterations made by an earlier drive with unexpected side effects. They might also be used to reverse conventionally engineered or evolved changes. Immunization drives could prevent other gene drives from affecting a specific population or provide a population with resistance to DNA viruses. Precision drives could exclusively spread through a subpopulation with a unique gene or sequence. (B) Together, these can quickly halt an unwanted drive and eventually restore the sequence to the original wild-type save for the residual Cas9 and guide RNAs. (C) Any population with limited gene flow can be given a unique sequence by releasing drives A and B in quick succession. So long as drive A does not escape into other populations before it is completely replaced by drive B, subsequent precision drives can target population B without risking spread into other populations.
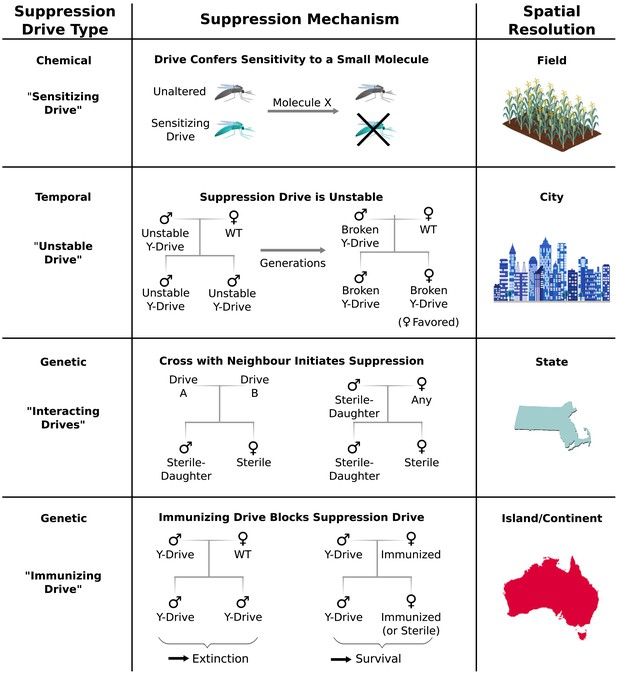
Controlling population suppression.
Previously proposed genetic load and meiotic suppression drives spread without limit and may incur a substantial risk of extinction. Alternative gene drive types might be used to grant finer control over the extent of suppression. ‘Sensitization drives’ would be harmless save for conferring vulnerability to a particular chemical, which could then be used as a population-specific pesticide. Evolutionarily ‘unstable drives’ would place a limit on the average number of drive copying events and thus the extent of population suppression. ‘Interacting drives’ would initiate suppression only upon encountering a specific genetic signature in the population, in this case a different gene drive. The combination would create a sterile-daughter effect (Figure 6—figure supplement 1) capable of continuing suppression for several generations. Finally, an immunizing drive could protect a subpopulation from a full genetic load or male-biasing suppression drive employed elsewhere. Interacting drive and immunizing drive approaches would be effective on very large populations spread across substantial geographic areas (Figure 6—figure supplement 2) while suffering from correspondingly reduced geographic resolution and greater ecological risk (Figure 6—figure supplement 3). Resolutions are approximations only and will vary with the specific drive utilized in each class.
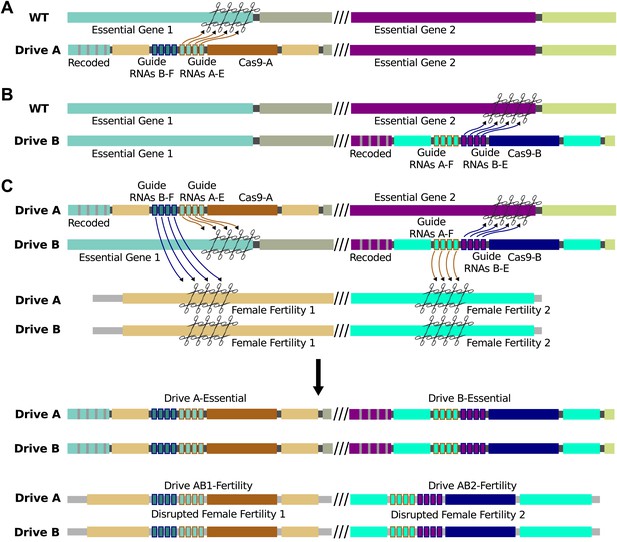
Sample interacting drives that produce a sterile-daughter effect.
(A) Drive A spreads through the wild-type population without any phenotypic effect using Cas9-A and guide RNAs A-E (essential). It also carries guide RNAs B-F (fertility), which cannot be utilized by Cas9-A. (B) Drive B spreads through the wild-type population without any phenotypic effect using Cas9-B and guide RNAs B-E (essential). It also carries guide RNAs A-F (fertility), which cannot be utilized by Cas9-B. Cas9-A and Cas9-B are orthogonal in that they do not recognize one another's guide RNAs. (C) When organisms bearing Drives A and B are crossed, offspring inherit one copy of each. Both drives are copied as normal. In addition, Cas9-A (from Drive A) uses guide RNAs A-F (from Drive B) to cut a gene essential for female fertility, while Cas9-B (from Drive B) does the same using guide RNAs-B-F (from Drive A) to cut a different gene. Drives A and B have internal homology appropriate to be copied into these cut fertility genes, disrupting them and causing infertility in females. If all repairs are conducted via homologous recombination, there are now four drives: A, B, AB1, and AB2. In reality, recombination between the non-homologous chromosomes is likely to be less efficient, causing loss of the female fertility genes but not explicit copying of AB1 and/or AB2. The phenotypic effect is identical.
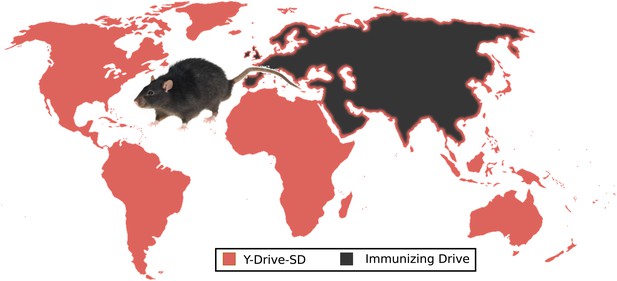
An extreme example of ecological management: the use of suppression and immunizing drives to control rat populations worldwide.
Releasing Y-Drive-SD (male-biasing sterile-daughter) rats into invasive rat populations would initiate local eradication while an immunizing drive protectively recoded the native rat populations of Eurasia. Stowaway-mediated gene flow, depicted as a border of Y-Drive-SD framing Eurasia, will result in local population suppression due to the sterile-daughter effect that would remain when the drives interacted, limiting the ability of either population to invade the other. This control process would have to be repeated with new drives once recoded stowaways successfully re-invade the rat-free habitats. Because of the complexity of gene flow patterns in rats, the uncertainty of whether a Y-Drive-SD would be effective, and the possibility for adverse human intervention, controlling rat populations in this way will not be feasible any time within the next decade and possibly not at all, but it provides a useful world-spanning example of a possible drive-based solution aiming to solve a serious global problem. Relying on precision drives to give specific invasive rat populations unique genetic markers (Figure 5C) for subsequent targeting with precision suppression drives or utilizing weaker suppression drive types (Figure 6) may represent more feasible alternatives.
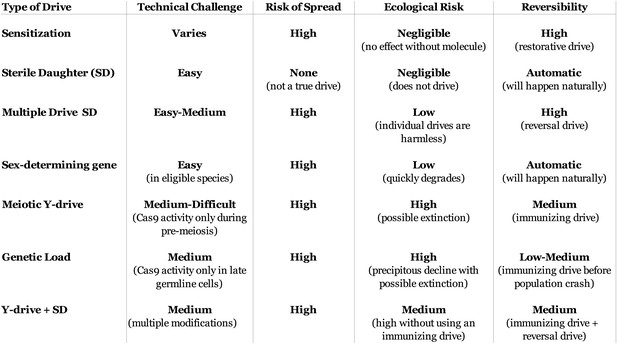
Characteristics of population suppression drives.
The optimal suppression drive for a given population will be highly species- and situation-dependent. In species with poorly understood genetics, more technically challenging drives may not be an option. While all true drives risk spreading into non-target populations with compatible sequences, the ecological risks to those populations vary. For some drives, a single individual escaping to a non-target population could cause widespread population suppression or even extinction, while others would require multiple sequential escapees of particular drive types. Some drives will be quickly eliminated by natural selection if not continually re-released, others would require a reversal drive to restore the wild-type sequence but should not have any phenotypic effects, and the most aggressive would require a suitable immunizing drive to be released within a certain timeframe in order to prevent a population crash. All of these risks could be mitigated by first recoding the target population to create a unique target sequence using successive standard drives (Figure 5A–B). ‘Sterile-daughter’ refers to a drive cassette that causes female infertility analogous to Drives AB1 and AB2 in Figure 6—figure supplement 1; because its fitness will be at most 50% of a standard gene, it cannot exhibit true drive.
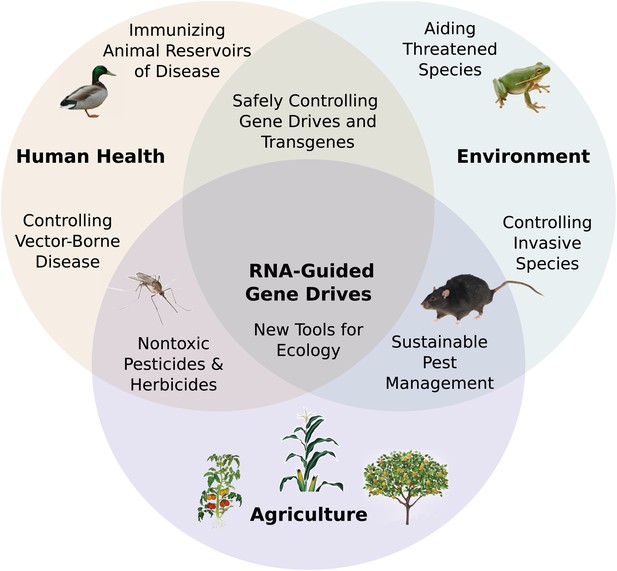
Potential applications of RNA-guided gene drives. Clockwise from left.
Disease vectors such as malarial mosquitoes might be engineered to resist pathogen acquisition or eliminated with a suppression drive. Wild populations that serve as reservoirs for human viruses could be immunized using Cas9, RNAi machinery, or elite controller antibodies carried by a gene drive. Reversal and immunization drives could help ensure that all transgenes are safe and controlled. Drives might quickly spread protective genes through threatened or soon-to-be-threatened species such as amphibians facing the expansion of chytrid fungus (Rosenblum et al., 2010). Invasive species might be locally controlled or eradicated without directly affecting others. Sensitizing drives could improve the sustainability and safety of pesticides and herbicides. Gene drives could test ecological hypotheses concerning gene flow, sex ratios, speciation, and evolution. Technical requirements for these applications vary with the drive type required (Figure 7—figure supplement 1).
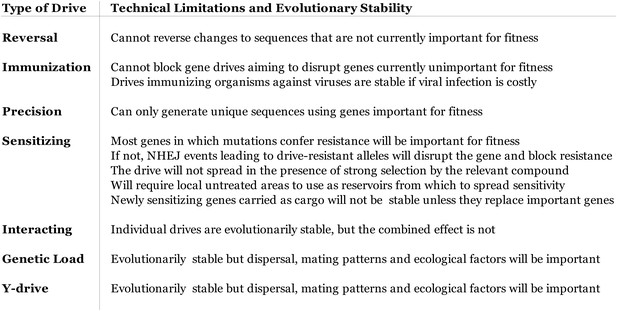
Technical limitations of different gene drive architectures with implications for various applications.
In addition to those listed above, an RNA-guided gene drive spreading through a population will be under selection to maintain the function of Cas9 and the guide RNAs, as any nonfunctional mutants will lose their inheritance advantage. This selective pressure is restricted to components that relate to drive function or efficiency and will only last for as long as the drive spreads. Once it reaches fixation, any mutations that can increase fitness by inactivating the drive components will be favored.
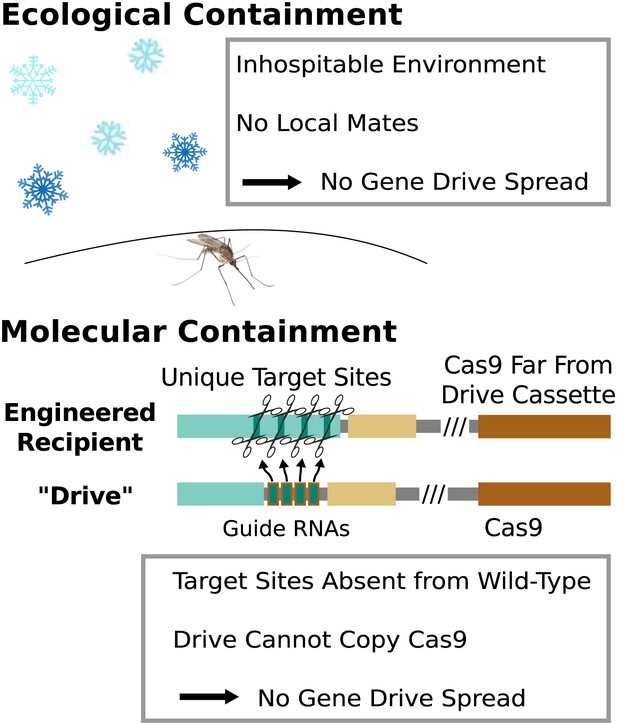
Containment strategies and ecological risk.
Ecological containment involves building and testing gene drives in geographic areas that do not harbor native populations of the target species. For example, most gene drive studies involving tropical malarial mosquitoes have been conducted in temperate regions in which the mosquitoes cannot survive or find mates. Molecular containment ensures that the basic requirements for drive are not met when mated with wild-type organisms. True drives must cut the homologous wild-type sequence and copy both the gene encoding Cas9 and the guide RNAs. Experiments that cut transgenic sequences absent from wild populations and copy either the gene encoding Cas9 or the guide RNAs - but not both - should be quite safe. Ecological or molecular containment should allow basic research into gene drive effectiveness and optimization to be pursued with negligible risk. Figure 8—figure supplement 1 categorizes these and many other possible experiments according to estimated risk.
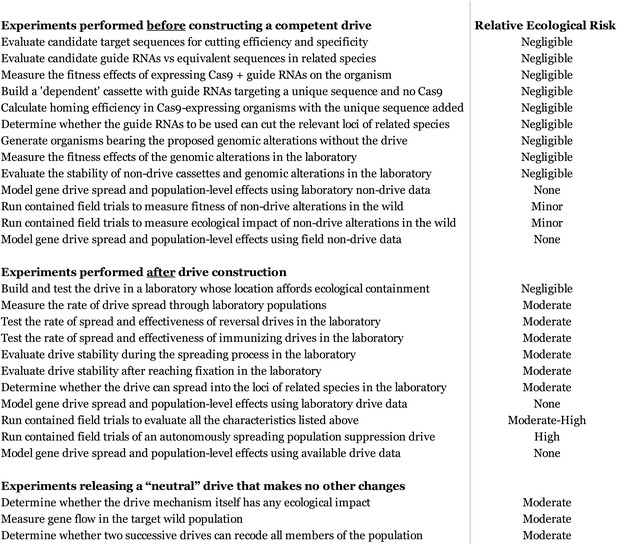
Estimated ecological risk of experiments during RNA-guided gene drive development.
This should not be considered an exhaustive list, but includes many relevant experiments that might be performed during the development of an RNA-guided gene drive of any type. The assessment of ecological risk is intended to be a guideline only and will vary with the purpose of the drive. For example, releasing a laboratory-tested ‘neutral’ drive that is intended to cause no changes in organism phenotype beyond spreading its cas9 gene, guide RNAs, and recoded essential gene through the population is listed as a moderate risk because it will certainly edit a wild population, but in a way that is comparatively unlikely to affect its interactions with the ecosystem. Contained field trials of drives that do cause phenotypic changes are listed as moderate-high risk due to the very real possibility of a containment breach and subsequent unintentional population engineering; a standard drive with few or no expected phenotypic effects would represent a moderate risk, while a suppression drive would represent a high risk.