Oxygen isotopes suggest elevated thermometabolism within multiple Permo-Triassic therapsid clades
Abstract
The only true living endothermic vertebrates are birds and mammals, which produce and regulate their internal temperature quite independently from their surroundings. For mammal ancestors, anatomical clues suggest that endothermy originated during the Permian or Triassic. Here we investigate the origin of mammalian thermoregulation by analysing apatite stable oxygen isotope compositions (δ18Op) of some of their Permo-Triassic therapsid relatives. Comparing of the δ18Op values of therapsid bone and tooth apatites to those of co-existing non-therapsid tetrapods, demonstrates different body temperatures and thermoregulatory strategies. It is proposed that cynodonts and dicynodonts independently acquired constant elevated thermometabolism, respectively within the Eucynodontia and Lystrosauridae + Kannemeyeriiformes clades. We conclude that mammalian endothermy originated in the Epicynodontia during the middle-late Permian. Major global climatic and environmental fluctuations were the most likely selective pressures on the success of such elevated thermometabolism.
https://doi.org/10.7554/eLife.28589.001eLife digest
School textbooks often refer to “cold-blooded” and “warm-blooded” animals, but these terms are misleading. Rather than being cold, animals like reptiles have body temperatures that are mostly determined by their external environment and can actually achieve high body temperatures, for example, by basking in the sun. By contrast, “warm-blooded” mammals produce their own heat and typically maintain a body temperature that is warmer than their environment. As such, so-called warm-blooded animals are more accurately referred to as “endotherms” and cold-blooded animals as “ectotherms”.
Endothermic animals share several characteristics, including insulating layers – like fur or feathers – that keep the body warm, and a secondary palate that separates the mouth and nose for continuous breathing, even while eating. Many of these traits are seen in fossils belonging to a group of animals called the therapsids. Also known as the “mammal-like reptiles”, these animals are descended from ectothermic reptiles but are the ancestors of the endothermic mammals. They dominated the land between 270 and 220 million years ago, during periods of time called the Permian and the Triassic. They also survived two major mass extinction events, including the most devastating mass extinction in all of Earth’s history. However, when the ancestors of mammals became truly endothermic remains an open question. Previous studies that have tried to determine this by focusing on the physical characteristics of therapsids have not yet given a consistent date.
Rey et al. took a new approach to answer when endothermy first evolved in the mammal-like reptiles, and instead looked at the chemical makeup of minerals in over 100 fossils. Oxygen can exist in different forms called stable isotopes: oxygen-16 and the rarer and heavier oxygen-18. The ratio of these two isotopes in a fossil will depend on, among other things, where the animal lived and, importantly, its body temperature. Therefore, Rey et al. compared oxygen-containing minerals in the bones and teeth of therapsids to those of other animals that lived alongside them to look for signatures that indicated differences in body temperature and how it was regulated.
It appears that two different branches of the therapsid’s family tree independently became endothermic. One branch includes the mammals and their direct ancestors, while the second is more distantly related to mammals. Both became endothermic towards the end of the Permian Period, between about 259 and 252 million years ago. Based on these findings, Rey et al. suggest that endothermy allowed these animals to better cope with fluctuating climates, which helped them to be among the few species that survived the mass extinction event at the end of the Permian.
Going forward, these new findings can help scientists to understand which physical characteristics were necessary for endothermy to first develop and which helped to optimize it afterwards. Furthermore, they also suggest that endothermic animals are more able to survive fluctuations in climate, which could guide efforts to protect modern-day endangered species that are most at risk from the ongoing effects of climate change.
https://doi.org/10.7554/eLife.28589.002Introduction
One key adaptation enabling tetrapods to cope with fluctuating climatic conditions was the acquisition of endothermy (Paaijmans et al., 2013). This character is defined here as the ability to actively produce body heat through metabolic activity (Cannon and Nedergaard, 2004). Its development and anchoring in populations constitutes a major step in vertebrate evolution because it modified the energy relationships between organisms and their environments. By actively raising and maintaining body temperature within a narrow range that allows optimal physiological and biochemical functioning, endothermic vertebrates are able to colonise environments with extreme thermal conditions, for example freezing at high latitudes and altitudes (Day et al., 2015). Endothermy is commonly associated with homeothermy, being the capacity to regulate the body heat through metabolic activity as well. This combination corresponds to one end of a gradient of thermoregulatory strategies observed in living animals. The other end of the spectrum is ectothermy combined with poïkilothermy which animals use as a thermoregulatory strategy to increase their body temperature toward optimal levels by using external heat sources. Their body temperature therefore traces that of their surroundings and is the most commonly occurring energy saving strategy. Amongst modern vertebrates, various thermoregulatory strategies have been adopted between these two end-members, such as regional endothermy (Bernal et al., 2001; Katz, 2002) or inertial homeothermy (McNab and Auffenberg, 1976), and only birds and mammals fall within the endothermy end of the spectrum. It has been proposed that bird thermoregulation originated within non-avian dinosaurs (Seebacher, 2003; Amiot et al., 2006; Grady et al., 2014), or even earlier within basal archosauriforms (Farmer and Carrier, 2000; de Ricqlès et al., 2003; Seymour et al., 2004; Summers, 2005; Gower et al., 2014; Legendre et al., 2016). Various approaches have been tried by many researchers to assess the origin of mammalian endothermy (McNab, 1978; Bennett and Ruben, 1986; Farmer, 2000; Hillenius, 1992, 1994; Kemp, 2006a; Khaliq et al., 2014; Owerkowicz et al., 2015; Benoit et al., 2016b; Crompton et al., 2017). Some consider the appearance of endothermy to have either occurred during the transition from basal synapsid ‘pelycosaurs’ to therapsids, and to be either due to a shift in foraging ecology (Hopson, 2012) or due to a response to the availability of a seasonally arid, savanna-like biome by the end of the Early Permian (Kemp, 2006b).
How and why endothermy evolved in mammals remains a contentious issue, mostly because of the very low fossilization potential of anatomical and behavioural features associated with thermoregulation. Amongst the latter features, the presence of hair as an insulating integument is unequivocally associated with endothermy in all extant mammals. The oldest synapsid fossils preserved with fur imprints are Castorocauda (Ji et al., 2006) and Megaconus (Zhou et al., 2013). These early relatives of mammals were recovered from the Middle-Late Jurassic of China, implying that hair and fur appeared before ~165 Ma. The occurrence of retracted, fully ossified and non-ramified infraorbital canals (a structure associated with the presence of maxillary vibrissae) within non-mammaliaform Prozostrodontia, implies an older age of approximately 240 to 246 Ma for the occurrence of fur and hair (Benoit et al., 2016b).
Another anatomical character interpreted as associated with endothermy is the bony secondary palate. This is a feature associated with efficient respiratory capabilities considered to be linked to the high energy required for elevated metabolic rates. In some Triassic non-mammaliamorph therapsids, bauriid therocephalians and cynodonts, a bony secondary palate is fully developed (Abdala et al., 2014). It is noteworthy that a complete bony secondary palate is also present in dicynodonts, however it is primarily formed by the premaxilla (King, 1988) and not the maxilla as documented in therocephalians, cynodonts and extant mammals. Although a secondary osseous palate is ubiquitous in mammals, it also occurs in a few ectotherms (crocodiles, scincid lizards), thus questioning its direct link to endothermy (Bennett and Ruben, 1986).
Almost all extant endotherms possess nasal turbinate bones covered with mucosa that reduce heat loss and moisten air during respiration (Owerkowicz et al., 2015). This feature, absent in extant ectotherms (Witmer, 1995), may have been present in therocephalian, cynodont and dicynodont therapsids, as postulated from bony ridges in the nasal cavities interpreted as supports for the turbinate complex (Hillenius, 1992, 1994; Crompton et al., 2017).
A peculiar histological structure of fast-growing bone associated with highly vascularised woven-fibred matrix and primary osteons known as fibrolamellar bone (FLB), is another feature often used as evidence of a high metabolic activity (Montes et al., 2010; Legendre et al., 2016). Accordingly, several bone palaeohistological studies have addressed the quest for the presence of FLB in therapsids (de Ricqlès, 1972, 1979; Botha, 2003; Botha and Chinsamy, 2001, 2004; Ray et al., 2004; Olivier et al., 2017). Ray et al. (2004) and Olivier et al. (2017) analysed several therapsid groups (anomodont, gorgonopsian, therocephalian, cynodont) and found FLB in some genera (Aelurognathus, Pristerognathus, Tritylodon, Oudenodon, Lystrosaurus, Moghreberia), suggesting sustained fast growth, and thus elevated metabolic activity. The presence of FLB has also been demonstrated in some earlier non-therapsid synapsids such as Sphenacodon, Dimetrodon or even Ophiacodon (Huttenlocker et al., 2010; Shelton et al., 2012; Shelton and Sander, 2017). However, FLB also occurs in a few ectotherms such as in some turtles and crocodilians, and is absent in small mammals and passerine birds (Bouvier, 1977), showing that FLB is mostly correlated with high growth rates, which does not always correlate to high metabolic rates. Therefore, these characters alone cannot be considered as definitive evidence of endothermy, leaving the question of the timing and selection pressure for the origin of mammal endothermy still heavily debated.
Because the oxygen isotope fractionation between bone or tooth phosphate and body fluids is temperature dependent, and phosphate has a strong resistance to diagenetic alteration, oxygen isotope compositions of therapsid apatite phosphate (δ18Op) has been used in this pilot study to investigate the origin of mammalian endothermy. Indeed the δ18Op value of vertebrate apatite (bone, tooth) reflects both the oxygen isotope composition of the animal body water (δ18Obw) and its body temperature (Tb). Body water derives mainly from drinking meteoric water or plant water (D’Angela and Longinelli, 1990; Kohn, 1996a), and the δ18O value of this water in turn depends on climatic parameters such as air temperature, hygrometry, and amount of precipitation (Dansgaard, 1964; von Grafenstein et al., 1996; Fricke and O'Neil, 1999).
Variations in the δ18O values of ectotherm apatite, along with increasing latitude, are expected to reflect decreasing air temperatures as their body temperatures follow those of the environment. In contrast endotherms, which have a constant body temperature, should not be affected by environmental temperatures changes. Moreover, physiological adaptation to specific habitat use (aquatic, semi-aquatic or terrestrial) affects the δ18Obw value by controlling the magnitude of body input and output oxygen fluxes, some of them being associated with oxygen isotopic fractionations (Amiot et al., 2010). Therefore, co-existing endotherms and ectotherms should have distinct apatite δ18Op values reflecting their body temperature and ecological differences. By comparing apatite δ18Op values of therapsids with those of co-existing ectotherms of known ecologies at various palaeolatitudes, it should be possible to infer therapsid thermophysiology, a methodology that has previously been applied to non-avian dinosaurs (Fricke and Rogers, 2000; Amiot et al., 2006).
Following the protocol of previous research undertaken to establish the Permo-Triassic climatic conditions that prevailed during which South African tetrapods, including therapsids, radiated (Rey et al., 2016), this study aims to investigate thermophysiological strategies developed by various Permo-Triassic therapsid groups using the stable oxygen isotope compositions of their phosphatic remains. Our results add new data to the discussion of the origin of mammalian endothermy and its link to global climatic change.
Results
Permian therapsids
The 13 sampled South African Permian therapsids come from three different assemblage zones (AZ) of the Beaufort Group: the lower Tapinocephalus AZ, the Tropidostoma AZ and the lower Daptocephalus AZ (Viglietti et al., 2016).
Oxygen isotope compositions of three therapsid genera (Dicynodon, Diictodon and Oudenodon) from the youngest assemblage zone (lower Daptocephalus AZ) and seven therapsid genera (Aelurosaurus, Diictodon, Ictidosuchoides, Oudenodon, Rhachiocephalus, Tropidostoma and a basal cynodont) from the Tropidostoma AZ were respectively compared with one co-occuring Rhinesuchus and one co-occuring rhinesuchid. Differences between all the Permian therapsids and ectothermic temnospondyl range from +1.1 ± 0.6‰ to +8.0 ± 0.9‰ (Figure 1A), encompassing the expected range for which therapsids are considered ectothermic.
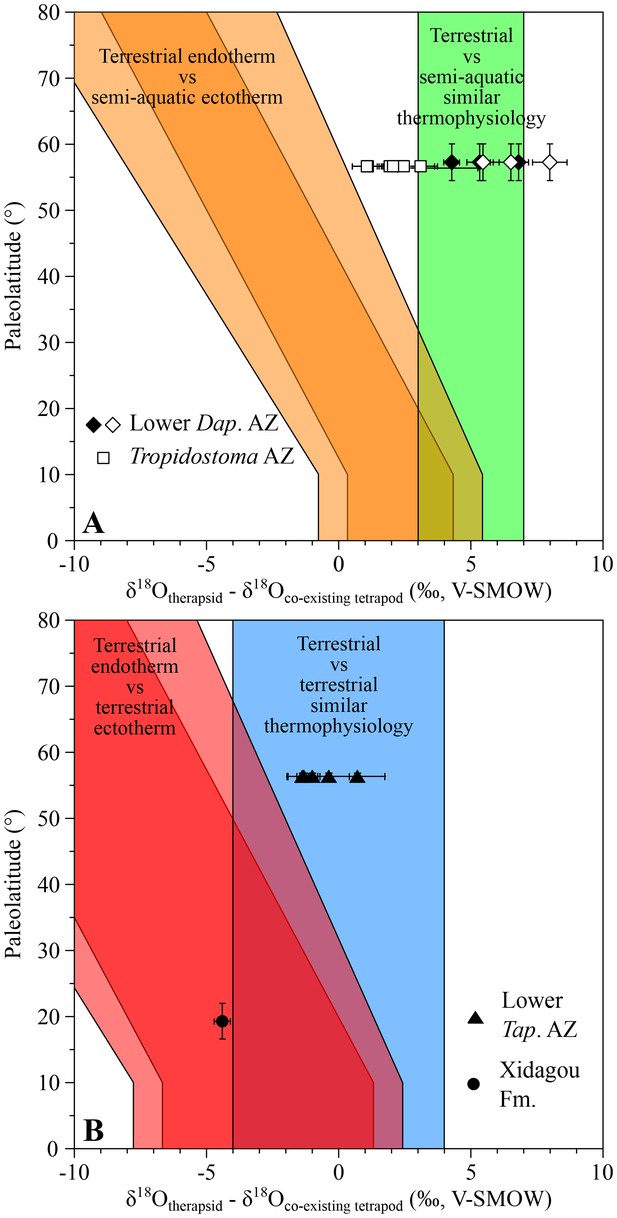
δ18Op differences between Permian therapsids and other tetrapods.
Differences in δ18Op values between therapsids and stereospondyls (white symbols) and between therapsids and parareptiles (black symbols) from the same localities are plotted against their corresponding palaeolatitude. A theoretical framework based on modern temperature gradient (0.6 ± 0.1°C/°Lat; see Appendix 1) and phosphate-water-temperature oxygen isotope fractionation (Lécuyer et al., 2013) predicts various δ18Op value differences. The lighter orange and red areas correspond to the uncertainty of the temperature gradient. Dap.: Daptocephalus; Tap.: Tapinocephalus.
In addition, δ18Op values of the therapsids Dicynodon, Diictodon and Oudenodon are compared to those of the supposedly semi-aquatic parareptile Pareiasaurus (Ivakhnenko, 2001; Kriloff et al., 2008) (see Appendix 1), with an observed range of +4.3 ± 0.4‰ to +6.8 ± 0.5‰ (Figure 1A) which is similar to that measured between therapsids and amphibians. This also supports the ectothermic status of Dicynodon, Diictodon and Oudenodon.
Anteosaurus, Criocephalosaurus, Struthiocephalus, Glanosuchus and a titanosuchid from the lower Tapinocephalus AZ have also been compared to two co-occuring basal pareiasaurs which are attributed to either Embrithosaurus, Nochelosaurus or Bradysaurus (Lee, 1997) and are considered to have been terrestrial animals (Canoville et al., 2014). From Figure 1B, the δ18Op differences range from −1.4 ± 0.6‰ to 0.7 ± 1.0‰, also supporting the ectothermic status of these therapsids.
From the Middle Permian of China, one anteosaurid Sinophoneus yumenensis from the low palaeolatitude locality of Dashankou has a δ18Op value 4.4 ± 0.3‰ lower than the co-existing bolosaurid parareptile Belebey chengi, which is considered to have been a terrestrial ectotherm (Berman et al., 2000; Müller et al., 2008). This difference between only two values would suggest that Sinophoneus was endothermic, but it is also very close to the expected ranges for ectothermic therapsids (Figure 1B). Considering Sinophoneus as semi-aquatic, as has been suggested for some anteosaurids (Boonstra, 1955, Boonstra, 1962), the δ18Op difference would imply an ectothermic thermophysiology for this therapsid. This hypothesis needs to be tested with a larger number of samples, which are not yet available.
Early to Middle Triassic therapsids
From the Cynognathus AZ (subzone B) of South Africa, differences between the therapsids Kannemeyeria, Cynognathus and Diademodon and the temnospondyl amphibians Xenotosuchus and Microposaurus range from −1.5 ± 1.1‰ to +0.9 ± 1.5‰ (Figure 2A), which fit within the range predicting endothermic therapsids. Interestingly, these therapsids have values ranging from 0.0 ± 1.6‰ to +1.8 ± 1.6‰ higher than the coexisting terrestrial archosauriform Erythrosuchus (Botha-Brink and Smith, 2011), a range suggesting that they shared a similar thermophysiology (Figure 2B). Therefore δ18Op values imply that, as in the case of the therapsids, Erythrosuchus was also endothermic which is consistent with the elevated growth rates implied by its palaeohistology (de Ricqlès et al., 2008; Botha-Brink and Angielczyk, 2010).
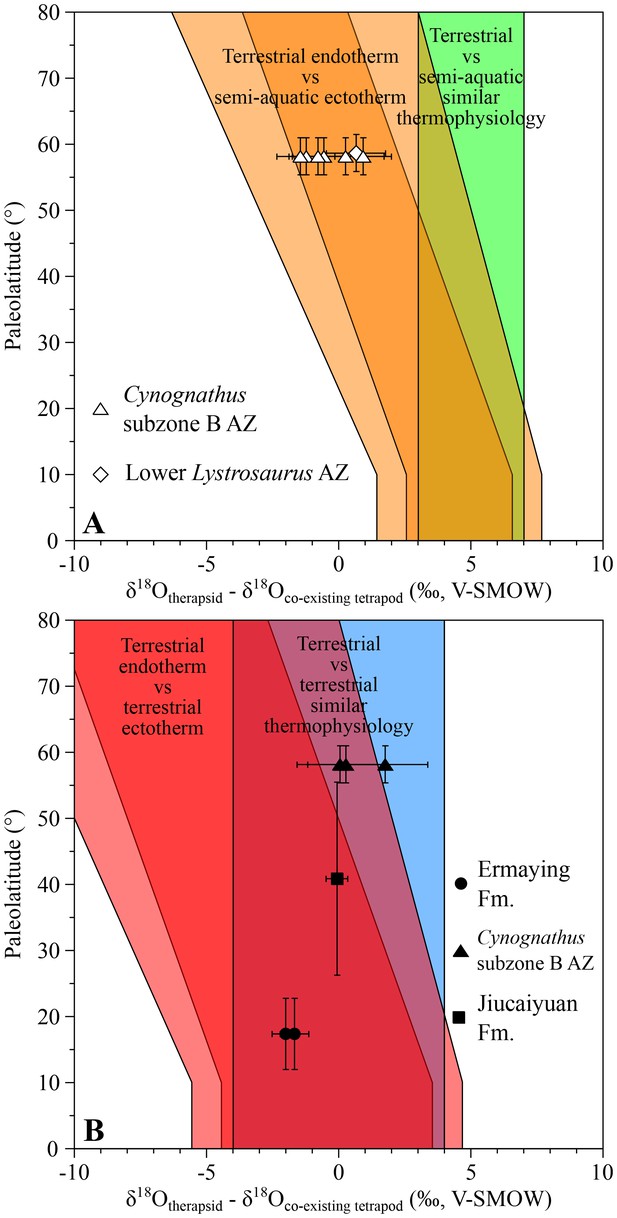
δ18Op differences between Early to Middle Triassic therapsids and other tetrapods.
Differences in δ18Op values between therapsids and stereospondyls (white symbols) and between therapsids and archosauriforms (black symbols) from the same localities are plotted against their corresponding palaeolatitude. A theoretical framework based on a lower-than-today thermal gradient (0.4 ± 0.1°C/°Lat; see Appendix 1) and phosphate-water-temperature oxygen isotope fractionation (Lécuyer et al., 2013) predicts various δ18Op value differences. The lighter orange and red areas correspond to the uncertainty of the temperature gradient.
Also from South Africa, five Lystrosaurus specimens from the lower Lystrosaurus AZ have δ18Op values similar to those of the co-existing semi-aquatic stereospondyl Lydekkerina (Schoch, 2008; Canoville and Chinsamy, 2015). In addition, an indeterminate lystrosaurid from the Induan Jiucaiyuan Formation of the Xinjiang Province has a δ18Op value similar (with a difference of −0.1 ± 0.6‰; Figure 2B) to that of the proterosuchid ‘Chasmatosaurus’ yuani, a basal archosauriform considered terrestrial and possessing an intermediate thermometabolsim based on a palaeohistological study (Botha-Brink and Smith, 2011). The combined results from South Africa and China suggest that the analysed lystrosaurids were terrestrial endotherms (Figure 2; see Appendix 1).
From the Ermaying Formation of the Shanxi Province (China), the therapsids Shansiodon wangi and Parakannemeyeria youngi have respectively δ18Op values of 2.0 ± 0.7‰ and 1.7 ± 0.7‰. These are both lower than the sampled erythrosuchid archosauriform Shansisuchus shansisuchus, which fall within two theoretical overlapping ranges (Figure 2B). As for the South African erythrosuchids, if we consider Shansisuchus as a terrestrial endotherm-like animal and the low palaeolatitude of this part of China region, then the two therapsids also fall within the range of endotherms.
Middle to Late Triassic therapsids
The late Anisian cynodont Diademodon and the kannemeyeriiform Kannemeyeria, from the Cynognathus AZ (subzone C), have both lower δ18Op values than those of the contemporary semi-aquatic stereospondyls Paracyclotosaurus and Xenotosuchus with differences ranging from −3.9 ± 2.7‰ to −0.5 ± 0.6‰ (Figure 3A). This pattern fits within the main range predicting endothermic therapsids.
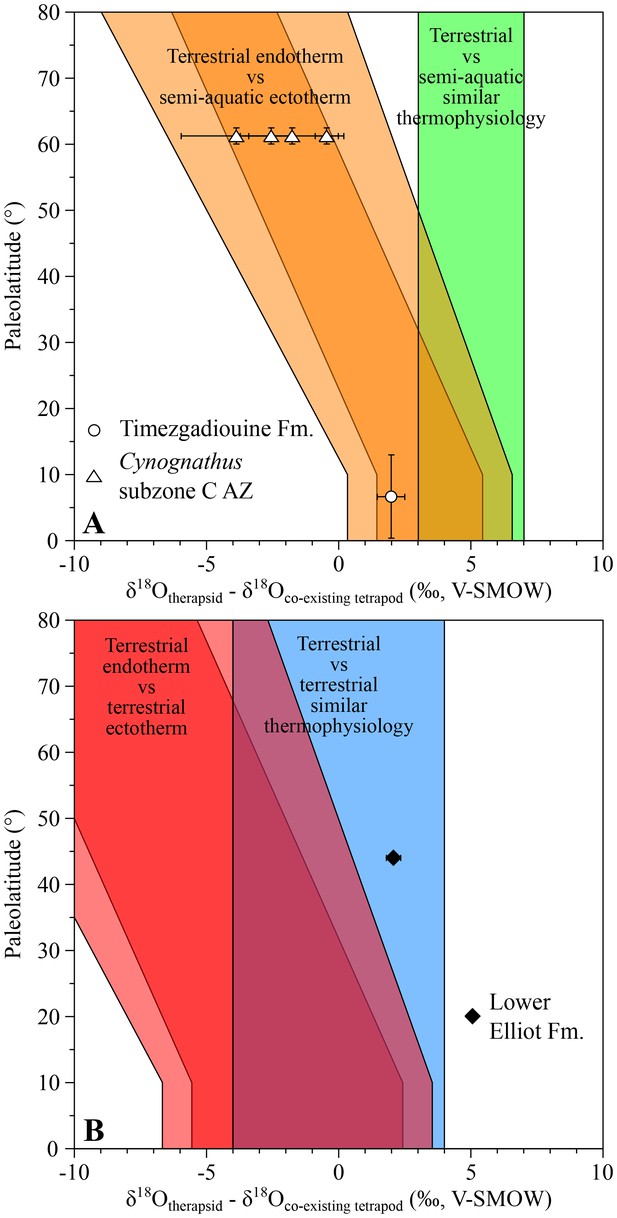
δ18Op differences between Middle to latest Triassic therapsids and other tetrapods.
Differences in δ18Op values between therapsids and stereospondyls (white symbols) and between therapsids and archosauriforms (black symbols) from the same localities are plotted against their corresponding palaeolatitude. A theoretical framework based on a lower-than-today thermal gradient (0.5 ± 0.1°C/°Lat; see Appendix 1) and phosphate-water-temperature oxygen isotope fractionation (Lécuyer et al., 2013) predicts various δ18Op value differences. The lighter orange and red areas correspond to the uncertainty of the temperature gradient.
The Moroccan kannemeyeriiform Moghreberia nmachouensis from the early middle Carnian of the Argana Basin has a mean δ18Op value 2.0 ± 0.5‰ higher than the co-existing aquatic stereospondyl Almasaurus habbazi (Figure 3A), thus implying that Moghreberia nmachouensis was also endothermic.
An indeterminate cynodont from the Rhaetian Lower Elliot Formation of Lesotho has a δ18Op value 2.1 ± 0.3‰, higher than that of an indeterminate basal sauropodomorph. The suspected endothermy and terrestriality of both dinosaurs (Amiot et al., 2006; D'Emic, 2015) and cynodonts are in agreement with their δ18Op difference that falls within the expected range predicting similar thermophysiology between the two (Figure 3B).
Discussion
According to the δ18Op value differences observed between therapsids and co-existing non-therapsid tetrapods, elevated thermometabolism seems to have been acquired by at least two therapsid clades: the unnamed dicynodont clade comprising Lystrosauridae + Kannemeyeriiformes, abbreviated the ‘L+K’ clade, and the Eucynodontia (Figure 4).
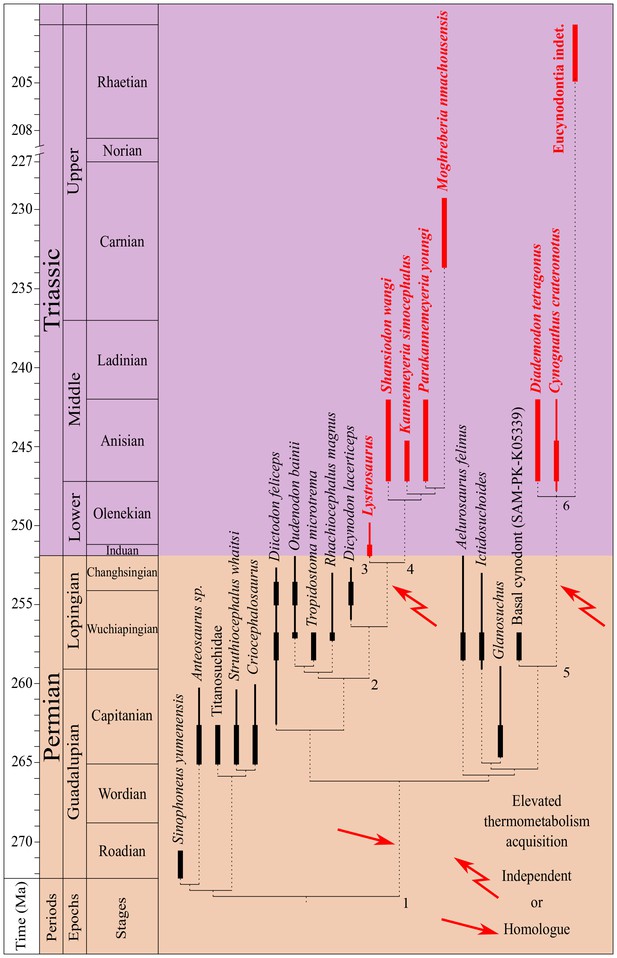
Phylogeny of sampled therapsids.
Phylogeny of the sampled therapsids plotted alongside a stratigraphic scale, based on proposed therapsid phylogenies (Ruben and Jones, 2000; Hillenius and Ruben, 2004; Gebauer, 2007; Cisneros et al., 2012; Liu, 2013; Ruta et al., 2013) and their biostratigraphic ranges (Kammerer et al., 2011; Kemp, 2012; Ruta et al., 2013; Huttenlocker, 2014; Day et al., 2015; Viglietti et al., 2016). The thickest parts of the bold lines represent the age range uncertainty of the localities where the samples come from. Species identified as endotherm-like are written in bold and red. Node numbers refer to clades quoted in the text: 1: Neotherapsida; 2: Dicynodontoidea; 3: Lystrosauridae; 4: Kannemeyeriiformes; 5: Epicynodontia; 6: Eucynodontia.
Among the interpreted endothermic therapsids, six belong to the L+K clade (Figure 4): Moghreberia nmachouensis, Parakannemeyeria youngi, Kannemeyeria simocephalus and Shansiodon wangi belong to the Kannemeyeriiformes clade, whereas the Lystrosauridae clade comprises Lystrosaurus and the Chinese indeterminate lystrosaurid. The interpretation of these taxa as endothermic-like animals is better supported for the African Kannemeyeriiformes, M. nmachouensis and K. simocephalus, where more individuals were sampled, than for the Chinese species, S. wangi and P. youngi. Concerning the lystrosaurids, Viglietti et al. (2013) demonstrated aggregating behaviour in the Early Triassic L. declivis and interpreted this as a means to keep warm under extreme climatic conditions. This is in agreement with our endothermic interpretation for the genus.
Based on the above interpretations, Dicynodon seems to have been ectothermic, a fact which would suggests the rise of endothermy amongst the dicynodont L+K clade during the latest Permian (Lopingian). This can be investigated in the future through the isotopic study of a basal dicynodontoid such as Daptocephalus from the Lopingian of South Africa (Kammerer et al., 2011; Viglietti et al., 2016) or Peramodon from the Salarevo Formation of Russia (Sushkin, 1926).
Based on our interpretations, the monophyletic group Eucynodontia (Ruta et al., 2013) (including Cynognathus, Diademodon, an indeterminate cynodont from Lesotho, and extant mammals) possessed endothermic-like metabolism. A parsimonious interpretation would imply rooting the origin of mammal endothermy within non-Eucynodontia Epicynodontia, between the end-Permian and the earliest Triassic. According to our results, the closest sampled relative of Eucynodontia, the late Permian basal cynodont (SAM-PK-K05339), was probably ectothermic. Therefore the origin of mammal endothermy could have taken place among ‘intermediate’ groups belonging to the Epicynodontia (such as Cynosaurus from the latest Permian or Thrinaxodon from the Early Triassic of South Africa) that have in the past been considered to have been endothermic, based on anatomical features (Hillenius and Ruben, 2004; Benoit et al., 2015, 2016a).
In agreement with recent phylogenies (Kemp, 2012; Ruta et al., 2013), endothermic-like body temperature regulations seem to root sometime during the late Permian (Lopingian) independently within the L+K and Epicynodontia clades, the latter being at the origin of mammal endothermy.
An alternative hypothesis considers that both the L+K and Epicynodontia clades possessed a homologous endothermy inherited from their direct common ancestors, the basal Neotherapsida (Figure 4). This suggests that biochemical and physiological mechanisms enabling mammal endothermy, appeared at the base of the neotherapsids during the middle Permian, which is a conclusion recently published based on the paleohistology of some dicynodonts (Olivier et al., 2017). In our case, the absence of an endothermic signal in the sampled Permian therapsids could be due to an endothermy being only seasonal, linked to the presence of a cold season or to the reproduction period (as observed today in some reptile species; Tattersall et al., 2016), which would not be visible in a bulk signal. Therefore, effective acquisition of mammal ‘true endothermy’ was expressed independently within these two lineages, possibly as a result of extrinsic factors.
Global and regional palaeoclimate reconstructions show a cooling trend toward the end-Permian, followed by an abrupt and intense warming at the Permian-Triassic Boundary (Chen et al., 2013; Rey et al., 2016). Interestingly, most of the therapsid clades which survived the end-Permian mass extinction were supposedly endothermic. It thus appears that climatic fluctuations may have acted as selective pressures which favoured or ‘activated’ elevated thermometabolic capabilities within therapsids, at the origins of mammal endothermy. A possible explanation could be the acquisition of a fast growth rate due to the high metabolic rate of the endothermy. According to a recent palaeohistology study (Botha-Brink et al., 2016), Early Triassic therapsids, such as Lystrosaurus or even therocephalians and cynodonts, had a high growth rate allowing them to reach reproductive maturity within a few seasons and compensate their shortened life expectancies. This adaptation might have enabled certain therapsids to survive the intense climatic change of that time and conquer the newly vacant niches.
Concluding remarks
In order to investigate the origin of mammal endothermy amongst the Permo-Triassic therapsids, stable oxygen isotope compositions of apatite phosphate and carbonate from therapsids and associated taxa recovered from several palaeolatitudes were analysed. The following results are highlighted:
Assuming that analysed samples have preserved their original isotope composition of phosphate, all the Permian therapsids analysed appear to have ectotherm-like thermoregulation and representatives of two Triassic therapsid clades are considered to have had endotherm-like thermoregulation: the Lystrosauridae + Kannemeyeriiformes and the Eucynodontia.
It is proposed that constant elevated thermometabolism appeared independently, at least twice during therapsid evolution. Following the principles of parsimony and phylogenetic systematics, both evolutionary events occured during the late Permian.
It seems that the timing of the acquisition of elevated thermometabolism among amniotes coincides with major global climatic and environmental fluctuations at the Permo-Triassic boundary and may had a selective advantage to survive the extinction event and result ultimately in mammalian endothermy.
Material and methods
Sample collection
Request a detailed protocolNineteen new fossil apatite samples were analysed to determine stable oxygen isotope compositions of apatite phosphate and carbonate, along with 89 samples for which oxygen isotope compositions have already been published (Rey et al., 2016; Supplementary file 1). This sample total comprises 41 teeth and 65 bones of 90 individual tetrapods (Therapsida, Archosauriformes, Parareptilia and Stereospondyli) recovered from Permian and Triassic deposits of South Africa, Lesotho, Morocco and China. All the sample localities are correlated to the marine biostratigraphic stages using the absolute ages accepted by the International Commission on Stratigraphy (Cohen et al., 2013); updated 12/2016), with the Permo-Triassic and Guadalupian-Lopingian boundaries now respectively considered to be at 251.90 ± 0.02 Ma (Burgess et al., 2014) and 259.1 ± 0.5 (Zhong et al., 2014) Ma.
South African samples comprise Permian and Triassic bones and teeth of therapsids, pareiasaurs, archosauriforms and stereospondyls recovered from 10 localities in the Beaufort Group (Karoo Supergroup), and housed in the collections of the Iziko South African Museum, Cape Town (SAM, Supplementary file 1) and at the Evolutionary Studies Institute, University of the Witwatersrand, Johannesburg (ESI, Supplementary file 1). Permian biozone ages of South African localities were taken from (Rubidge et al., 2013; Day et al., 2015), whereas Triassic age determination has been achieved by biostratigraphic correlation with Laurasian sequences (Hancox et al., 1995; Rubidge, 2005; Abdala and Ribeiro, 2010).
Lesotho samples comprise a cynodont therapsid and a basal sauropodomorph dinosaur from a Triassic locality near the town of Pokane, and are part of the Paul Ellenberger Collection at the Institut des Sciences de l’Evolution, University Montpellier, France (ISEM, Supplementary file 1). The locality belongs to the ‘Red Beds inférieurs a or b’ of the lower Elliot Formation which is currently regarded as latest Triassic (late Rhaetian) (Knoll, 2004).
Moroccan samples comprise therapsid and stereospondyl bones recovered from the ‘Locality 11’ of the Argana Group (Jalil, 1999) near the village of Alma, and housed at the Museum National d’Histoire Naturelle, Paris, France (MNHN, Supplementary file 1). The locality is biostratigraphically correlated to the upper Timezgadiouine Formation, considered to be Middle to early Late Carnian (Jalil, 1999).
Chinese samples are from Permian and Triassic localities situated in Gansu, Shanxi and Xinjiang provinces and comprise therapsids found in association with archosauriforms or parareptiles. These remains are curated at the Institute of Vertebrate Paleontology and Paleoanthropology in Beijing, China (IVPP, Supplementary file 1). The Dashankou locality, from Gansu Province, is biostratigraphically dated as Early Roadian (Liu et al., 2009; Liu, 2010). From Shanxi Province, sampled fossils originate from three localities in the Ermaying Formation which is considered to be Anisian (Liu et al., 2013). From Xinjiang Province, two localities in the Jiucaiyuan Formation have been sampled and are considered Early Triassic (Metcalfe et al., 2009).
Calculation of palaeogeographic coordinates of the sampling sites was performed after careful selection of the magnetic poles of West Gondwana (Muttoni et al., 2001), North China ( et al., 1992), South Jungar (Choulet et al., 2013) and the Alashan terrane (Meng, 1992; Yuan and Yang, 2015). The Apparent Polar Wander Path (APWP) of South Africa (Torsvik et al., 2012) was used to constrain the palaeolatitudinal position of the South African and Lesotho fossil sites. Palaeolatitudes and associated uncertainties (A95) are shown in Supplementary file 1.
Analytical techniques
Request a detailed protocolTo measure the oxygen isotope composition of the apatite phosphate group, the phosphate ions were isolated using acid dissolution and anion-exchange resin applying a standard protocol (Lécuyer, 2004). Silver phosphate was quantitatively precipitated in a thermostatic bath set at a temperature of 70°C. After filtration, washing with double deionized water and drying at 50°C, an aliquot of 300 μg of Ag3PO4 was mixed with 300 μg of nickelised carbon in a silver reaction capsule. Silver phosphate was then reduced into CO to measure its 18O/16O ratio (Lécuyer et al., 2007; Fourel et al., 2011). Each sample was heated at 1450°C by pyrolysis using a VarioPYROcube EA system (Elementar) interfaced to an IsoPrime isotope ratio mass spectrometer working in continuous flow mode at the UMR CNRS 5276 LGLTPE, University Claude Bernard Lyon 1.
Isotopic compositions are quoted in the standard δ notation relative to V-SMOW. Silver phosphate precipitated from standard NBS120c (natural Miocene phosphorite from Florida) was repeatedly analysed (δ18O = 21.71 ± 0.20‰; n = 30) along with the silver phosphate samples derived from the tetrapod remains. For the oxygen isotope analysis of apatite carbonate, about 10 mg of tooth or bone powder was pre-treated (Koch et al., 1997). Powders were washed with a 2% NaOCl solution to remove organic matter, then rinsed five times with double deionized water and air-dried at 40°C for 24 hr. Potential secondary carbonate was removed by adding 0.1 M acetic acid and leaving for 24 hr, after which the powder was again rinsed five times with double deionized water and air-dried at 40°C for 24 hr. The powder/solution ratio was kept constant at 0.04 g mL−1 for both treatments. Stable isotope ratios were determined by using a Thermo Finnigan Gasbench II at the geochemistry laboratory of the Institute of Geology and Geophysics (Chinese Academy of Sciences, China). For each sample, an aliquot of 2 mg of pre-treated apatite was reacted with 5 drops of supersaturated orthophosphoric acid at 72°C for one hour under a He atmosphere before starting 10 measurement cycles of the isotopic composition of the CO2 produced with a Finnigan MAT 253 continuous flow isotope ratio mass spectrometer. The measured oxygen isotopic compositions were normalized relative to the NBS-19 calcite standard and have a reproducibility index better than ±0.2‰. Isotopic compositions are quoted in the standard δ notation relative to V-SMOW.
Robustness of the stable isotope record
Request a detailed protocolAnalysed materials consist of bone or tooth dentine, which is more porous than enamel with small and less densely inter-grown apatite crystals (Mills, 1967). Thus, their original stable isotope compositions are more prone to diagenetic alteration that may have taken place through precipitation of secondary minerals within and at the surface of bioapatite crystals, adsorption of ions on the surface of apatite crystals, or dissolution and recrystallization with isotopic exchange. The samples from South Africa have been previously tested for primary preservation through comparison between their δ18Op values, δ18Oc values and carbonate content on the basis of the following considerations: (1) the carbonate content in apatite of modern vertebrates typically ranges from less than 1% up to 13.4%. Thus, samples that have a carbonate content exceeding 13.4 wt% likely contain additional inorganic carbonate precipitated from diagenetic fluids, and would result in potentially biased δ18Oc values of apatite carbonate (Figure 5); (2) In modern vertebrates, the oxygen isotope composition of apatite carbonate is higher than that of co-occurring apatite phosphate (7–9 ‰ in mammals), and up to 14.7‰ in sharks (Vennemann et al., 2001). Experimental ( et al., 1967) and empirical studies (Zazzo et al., 2004b) have shown that microbially-mediated diagenetic alteration of apatite phosphate results in a greater difference between δ18Oc and δ18Op values. Therefore, fossil samples exhibiting δ18Oc-δ18Op differences larger than 14.7‰ are most likely altered and can be disregarded (Figure 5). Inorganic alteration at low temperature has little effect on the δ18Op values of phosphates, even at geological time scales (Lecuyer et al., 1999), so samples affected by inorganic diagenetic alteration of carbonates, (resulting either in a high overall carbonate content or anomalous δ18Oc-δ18Op differences), may still preserve the original oxygen isotope composition of their phosphate (Figure 5). Using these two assessments, newly measured δ18Op values are considered to have preserved their original isotopic signatures and can be interpreted in terms of ecologies and physiologies.
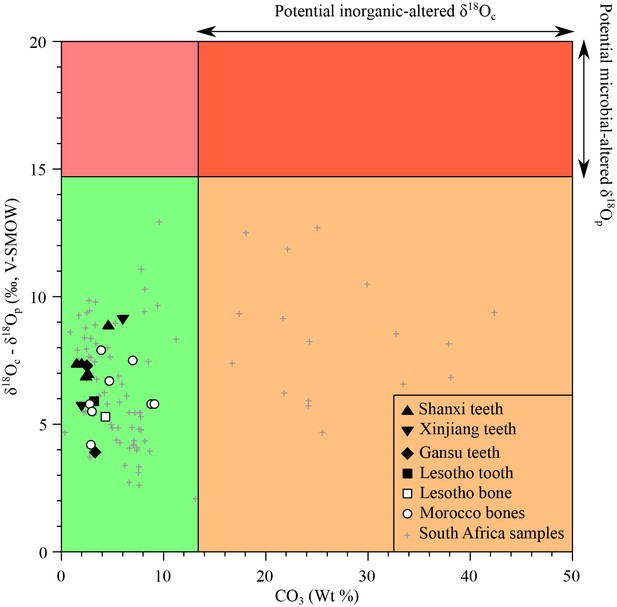
Isotopic preservation assessment.
δ18Oc-δ18Op differences between teeth and bones plotted against the structural carbonate content (wt%) of apatite. Samples that have δ18Oc-δ18Op differences higher than 14.7‰ are considered doubtful as regards potential diagenetic alteration (see text). For carbonate contents (wt%) higher than 13.4%, the δ18Oc values are considered to be inherited from inorganic diagenetic processes. A high difference between δ18Oc and δ18Op is interpreted as the result of a microbially-mediated alteration of the apatite phosphate or too high δ18Oc values resulting from the addition of inorganic carbonate or isotopic exchange with an external source of inorganic carbon. The grey crosses refer to previously published South African bone and tooth samples (Rey et al., 2016).
Assessment of therapsid thermophysiology
Request a detailed protocolFor all localities, average δ18Op values were calculated for each tetrapod species. Differences in δ18Op values between therapsid species and co-occurring non-therapsid tetrapods (amphibians, parareptiles or archosauriforms) were calculated and plotted against their corresponding palaeolatitude for three time intervals: the middle to late Permian (Figure 2), the Early to Middle Triassic (Figure 3) and the Middle Triassic to latest Triassic (Figure 4). These differences were compared to the following four theoretical areas of values represented as coloured areas in Figures 1–3. To construct those theoretical areas, both the phosphate-water temperature scale from Lécuyer et al., 2013 and the differences of stable oxygen compositions between mammals of various ecologies from Cerling et al. (2008) have been used. (see Appendix 1 for their construction details).
Orange and green areas in Figures 1A, 2A and 3A represent expected δ18Op value differences between terrestrial therapsids and semi-aquatic stereospondyls (white symbols) or parareptiles (black symbols); red and blue areas in Figures 1B, 2B and 3B represent expected δ18Op value differences between terrestrial therapsids and terrestrial Permian parareptiles or Triassic archosauriforms (black symbols). Oblique orange and red areas in Figures 1–3 represent expected δ18Op value differences between an endotherm and an ectotherm. Vertical green and blue areas in Figures 1–3 represent expected δ18Op value differences between animals having similar thermophysiology.
Appendix 1
Theoretical curves construction
In order to infer therapsid thermophysiologies based on the δ18Op differences between therapsids and the associated fauna, the following theoretical framework is proposed and represented in Figures 1, 2 and 3 as colored areas. These areas are based on the two main factors influencing animal δ18Op values: their thermometabolism (here simplified as ectotherm and endotherm) and their lifestyle (here terrestrial and semi-aquatic).
The δ18Op value ranges are estimated based on the phosphate-water temperature scale (Lécuyer et al., 2013):
Where Tb corresponds to body temperature, δ18Op correspond to the oxygen isotope composition of apatite phosphate, and δ18Obw correspond to the oxygen isotope composition of body fluids. For endothermic vertebrates, Tb is assumed to be at 37°C, the average Tb of most extant placentals and possibly of the common ancestor of all extant mammals (Watson and Graves, 2013). For ectothermic vertebrates, Tb is assumed here to reflect immediate environmental temperature (Te). According to Equation 1, the δ18Op difference between an endotherm and an ectotherm can be expressed as:
Because vertebrate δ18Obw value depend on their ecology that affects the input-output balance of body water (Luz et al., 1984; Bryant and Froelich, 1995; Kohn, 1996a), the difference will mainly reflects that of their lifestyle (terrestrial, semi aquatic or aquatic), as well as their dependency on the surface water they ingest. Cerling et al., 2008, the δ18Op difference between water-dependent and water-independent terrestrial mammals can be up to 4‰. This is illustrated by the ranges 1 and 3 in Appendix 1—Figure 1 and by the red and blue ranges in the main text Figures 1A, 2A and 3A).
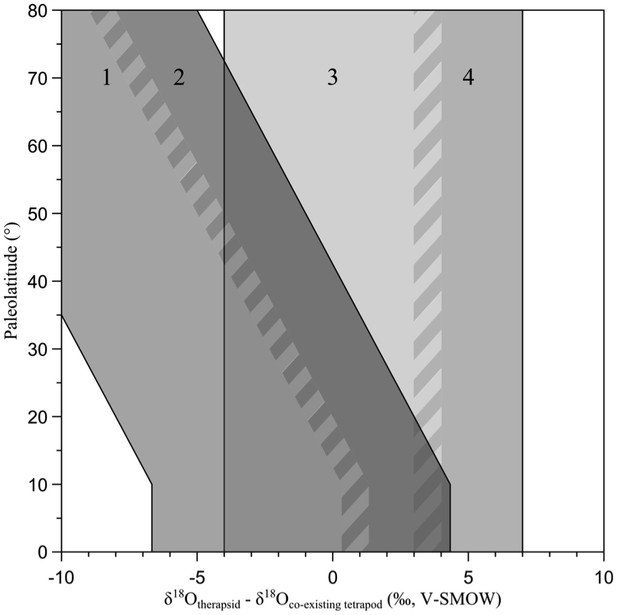
Expected latitudinal variation of the δ18Op difference between vertebrate taxa of various physiologies and ecologies.
Based on modern relationships between climate and phosphate-water-temperature oxygen isotope fractionation (Lécuyer et al., 2013) the following δ18Op values differences are predicted: (1) corresponds to a terrestrial endotherm compared to a terrestrial ectotherm; (2) corresponds to a terrestrial endotherm compared to a semi-aquatic ectotherm; The vertical range (3) corresponds to terrestrial animals having similar thermometabolism; (4) corresponds to the difference between a terrestrial and a semi-aquatic animals having a similar thermometabolism.
This variation in δ18Op values can be overprinted by the animal lifestyle, a semi aquatic animal having δ18Op value 3‰ lower than that of co-existing water-dependent terrestrial species, and 7‰ lower than that of co-existing water-independent terrestrial one (Appendix 1—figure 1, ranges 2 and 4, and main text Figures 1B, 2B and 3B, ranges orange and green).
The environmental temperature (Te) used to approximate ectotherm body temperature (Tb) is estimated based on the present-day relationship between mean air temperature and latitude. Because this latitude-temperature relationship is not valid for low latitudes below about 10° (corresponding to the thermal equators of present-day Earth), we assume a constant temperature between 0° and 10° of latitude.
As a simplification, three periods are considered:
The Middle to Late Permian with equatorial sea surface temperatures close to the modern ones (Chen et al., 2013) (~25°C) for which we assume a present-day thermal gradient of 0.6 °C/°Latitude (Amiot et al., 2004; Williams et al., 2007) (Main text Figure 1).
The Early to Middle Triassic having globally warmer temperatures and a flatter temperature gradient. Based on (Trotter et al., 2015), mean equatorial sea surface temperatures were about 10°C higher than Late Permian ones (Trotter et al., 2015) (~35°C). For this time period, we assume a flatter thermal gradient that we arbitrarily set at 0.4 °C/°Latitude (Main text Figure 2).
The Middle Triassic to Early Jurassic having globally intermediates temperatures (Trotter et al., 2015) that we set to 30°C with an intermediate gradient of 0.5 °C/°Latitude (Main text Figure 3).
In order to take into account the possible variations of the thermal gradient within the selected periods, an interval of ±0.1 °C/°L is added for each Main text figures.
Consequently, latitudinal thermal gradients will lead to δ18Op-endotherm - δ18Op-ectotherm differences varying along with latitude (Appendix 1—figure 1, ranges 1 and 2). This simplified framework is used in this study to predict the following scenarios based on δ18Op differences between therapsids and co-existing other tetrapods (Appendix 1—figure 1): Endothermic and terrestrial therapsid vs ectothermic and terrestrial tetrapod (range 1); Endothermic and terrestrial therapsid vs ectothermic and semi-aquatic tetrapod (range 2); Therapsids and other tetrapods having similar thermophysiologies and lifestyle (range 3); Terrestrial therapsid and semi-aquatic tetrapods having similar thermophysiologies (range 4).
Ecology of sampled tetrapods and its impact on stable oxygen isotope compositions
Prior to interpreting differences between therapsid and associated non-therapsid tetrapod δ18Op values in terms of differences in thermophysiologies, ecological traits must be considered as they also affect the oxygen isotope compositions of apatite phosphate. Indeed, δ18Op values recorded in phosphatic tissues depend on the animal body temperature, as well as on the oxygen isotope composition of body water, the latter being affected by water turnover rate and isotopic fractionations associated with water loss (Bryant and Froelich, 1995). Indeed, water loss through transcutaneous evaporation, sweat and exhaled water vapour tends to 18O-enrich the remaining body water (Kohn, 1996a). This isotopic enrichment is amplified or mitigated depending on the rate of water turnover, which depends itself on the animal ecology. Aquatic and semi-aquatic animals regularly ingest and release large amounts of their environmental water compared to terrestrial ones, which in turn reduces the magnitude of body water 18O-enrichment relative to that of environmental water (Kohn, 1996a).
Stereospondyl amphibians
The sampled stereospondyl amphibian clade (Almasaurus habbazi, Lydekkerina, Microposaurus, Paracyclotosaurus, Rhinesuchus and Xenotosuchus) includes semi-aquatic to aquatic animals (Schoch, 2008). A study dedicated to Rhinesuchus palaeohistology concluded that it had a fully aquatic lifestyle (McHugh, 2014), whereas Lydekkerina, a basal stereospondyl, was amphibious with a tendency to be terrestrial (Canoville and Chinsamy, 2015).
Pareiasaurid and bolosaurid parareptiles
The sampled parareptiles include the Chinese Bolosauridae, Belebey chengi, and the South African Pareiasauria, Pareiasaurus and basal pareiasaurs (Embrithosaurus, Nochelosaurus or Bradysaurus; (Lee, 1997). The Chinese taxon is considered to have been terrestrial (Berman et al., 2000; Müller et al., 2008), but interpretations of the life habits and habitats of the South Africa pareiasaurs still lack a consensus. According to authors, they are variously considered fully aquatic (Ivakhnenko, 2001), semi-aquatic (Kriloff et al., 2008) or fully terrestrial (Voigt et al., 2010; Canoville et al., 2014). The low δ18Op values measured in both Pareiasaurus and Rhinesuchus are similar to each other, Pareiasaurus having slightly higher δ18Op values than Rhinesuchus (~1‰), but significantly lower than those measured in therapsids (about 4‰ to 8‰). Considering Rhinesuchus as an aquatic ectothermic amphibian (McHugh, 2014), it is suggested that the ectothermic Pareiasaurus was also aquatic or semi-aquatic as previously suggested (Ivakhnenko, 2001; Kriloff et al., 2008). In contrast to the semi-aquatic Pareiasaurus from the Lower Daptocephalus AZ, the Tapinocephalus AZ pareiasaurs may have been terrestrial as recently proposed in a study on pareiasaur ecology and based on oxygen and carbon isotope compositions of apatite carbonate(Canoville et al., 2014). Moreover, those authors found no significant differences between therocephalians and pareiasaurs, agreeing with our dataset, and a ~ 3‰ overlap between pareiasaur and dinocephalian δ18Oc values, even though most dinocephalian values are several per mil lower than those of pareiasaurs.
Archosauriforms
Archosauriformes are represented by the Erythrosuchidae Erythrosuchus and Shansisuchus shansisuchus, by the Proterosuchidae ‘Chasmatosaurus’ yuani, as well as by an indeterminate basal sauropodomorph dinosaur. Proterosuchid lifestyle is still unresolved, but Botha-Brink and Smith, 2011 favoured a terrestrial rather than an aquatic lifestyle, whereas the erythrosuchids are considered as the largest terrestrial predators of their time (Botha-Brink and Smith, 2011).
Lystrosaurid therapsids
Therapsids are generally considered as terrestrial dwellers (Kemp, 2012) with a few uncertainties such as Anteosaurus considered as riparian (Boonstra, 1955, Boonstra, 1962) and Lystrosaurus considered by some as semi-aquatic (Germain and Laurin, 2005; Ray et al., 2005; Botha-Brink and Angielczyk, 2010; Canoville and Laurin, 2010).
Because the Lystrosaurus-Lydekkerina δ18Op difference falls within the same range as the Kannemeyeria-Xenotosuchus and Kannemeyeria-Microposaurus values from the Cynognathus subzone B assemblage, Lystrosaurus could be considered as having the same thermophysiology and lifestyle as Kannemeyeria, i.e. a terrestrial endotherm (Figure 2A). Because Lystrosaurus may have either been terrestrial (Botha and Smith, 2006) or semi-aquatic (Canoville and Laurin, 2010), it cannot be totally excluded that Lystrosaurus shared a similar lifestyle and thermometabolism with the amphibian Lydekkerina. If Lystrosaurus was indeed semi aquatic, then it could also be predicted to have been an ectotherm.
Given that the thermophysiology of the terrestrial archosauriform ‘Chasmatosaurus’ remains unclear, the two similar δ18Op values observed in the lower Triassic Jiucaiyuan Fm. of Xinjiang province of China may indicate either that both the lystrosaurid and the proterosuchid shared similar thermometabolism and lifestyle (terrestrial endotherms or terrestrial ectotherms; Figure 2), or that one of them may have had a lower body temperature. Indeed, compared to low latitudes where ectotherms have slightly lower δ18Op values than co-existing endotherms due to differences in metabolic activity and body temperatures, mid latitude ectotherms have even lower body temperatures (reflecting the environmental thermal gradient) resulting in higher δ18Op values that mimic those of co-existing endotherms (Amiot et al., 2004). It is worth noting that based on histological features, Proterosuchus, had intermediate growth rates, suggesting an intermediate thermometabolism (Botha-Brink and Smith, 2011) that could apply to the Chinese ‘Chasmatosaurus’. Considering a terrestrial lifestyle for the Chinese lystrosaurids (see above), and the conflicting hypotheses of Lystrosaurus from South Africa (terrestrial endotherm vs. semi-aquatic ectotherm), the hypothesis for terrestrial endothermy for the lystrosaurids is favoured.
References
-
BookNew Material of Microgomphodon oligocynus (Eutherapsida, Therocephalia) and the Taxonomy of Southern African BauriidaeIn: Kammerer C. F, Angielczyk K. D, Fröbisch J, editors. Early Evolutionary History of the Synapsida, Vertebrate Paleobiology and Paleoanthropology. Netherlands: Springer. pp. 209–231.
-
Distribution and diversity patterns of Triassic cynodonts (Therapsida, Cynodontia) in GondwanaPalaeogeography, Palaeoclimatology, Palaeoecology 286:202–217.https://doi.org/10.1016/j.palaeo.2010.01.011
-
Oxygen isotopes from biogenic apatites suggest widespread endothermy in cretaceous dinosaursEarth and Planetary Science Letters 246:41–54.https://doi.org/10.1016/j.epsl.2006.04.018
-
Latitudinal temperature gradient during the Cretaceous Upper Campanian–Middle Maastrichtian: δ18O record of continental vertebratesEarth and Planetary Science Letters 226:255–272.https://doi.org/10.1016/j.epsl.2004.07.015
-
BookThe Metabolic and Thermoregulatory Status of Therapsids. the Ecology and Biology of Mammal-Like ReptilesWashington, DC: Smithsonian Institution Press.
-
Review: analysis of the evolutionary convergence for high performance swimming in lamnid sharks and tunasComparative Biochemistry and Physiology Part A: Molecular & Integrative Physiology 129:695–726.https://doi.org/10.1016/S1095-6433(01)00333-6
-
The girdles and limbs of the South African DeinocephaliaAnnals of the South African Museum 42:185–326.
-
The dentition of the titanosuchian dinocephaliansAnnals of the South African Museum 46:57–112.
-
Growth patterns deduced from the bone histology of the cynodonts diademodon and CynognathusJournal of Vertebrate Paleontology 20:705–711.https://doi.org/10.1671/0272-4634(2000)020[0705:GPDFTB]2.0.CO;2
-
Growth and life habits of the triassic cynodont Trirachodon, inferred from bone histologyActa Palaeontologica Polonica 49:619–627.
-
Rapid vertebrate recuperation in the Karoo Basin of South Africa following the End-Permian extinctionJournal of African Earth Sciences 45:502–514.https://doi.org/10.1016/j.jafrearsci.2006.04.006
-
Biological aspects of the Permian dicynodont Oudenodon (Therapsida: dicynodontia) deduced from bone histology and cross-sectional geometryPalaeontologia Africana 39:37–44.
-
Do extraordinarily high growth rates in Permo-Triassic dicynodonts (Therapsida, Anomodontia) explain their success before and after the end-Permian extinction?Zoological Journal of the Linnean Society 160:341–365.https://doi.org/10.1111/j.1096-3642.2009.00601.x
-
Osteohistology of the Triassic archosauromorphs Prolacerta, Proterosuchus, Euparkeria, and Erythrosuchus from the Karoo Basin of South AfricaJournal of Vertebrate Paleontology 31:1238–1254.https://doi.org/10.1080/02724634.2011.621797
-
Brown adipose tissue: function and physiological significancePhysiological Reviews 84:277–359.https://doi.org/10.1152/physrev.00015.2003
-
Evolution of humeral microanatomy and lifestyle in Amniotes, and some comments on palaeobiological inferencesBiological Journal of the Linnean Society 100:384–406.https://doi.org/10.1111/j.1095-8312.2010.01431.x
-
Stable isotope ecology of the common hippopotamusJournal of Zoology 276:204–212.https://doi.org/10.1111/j.1469-7998.2008.00450.x
-
Structure of the nasal region of non-mammalian cynodonts and mammaliaforms: speculations on the evolution of mammalian endothermyJournal of Vertebrate Paleontology 37:e1269116.https://doi.org/10.1080/02724634.2017.1269116
-
Oxygen isotopes in living mammal’s bone phosphate: Further resultsChemical Geology: Isotope Geoscience Section 86:75–82.
-
A model of oxygen isotope fractionation in body water of large mammalsGeochimica Et Cosmochimica Acta 59:4523–4537.https://doi.org/10.1016/0016-7037(95)00250-4
-
When and how did the terrestrial mid-Permian mass extinction occur? evidence from the tetrapod record of the Karoo Basin, South AfricaProceedings of the Royal Society B: Biological Sciences 282:20150834.https://doi.org/10.1098/rspb.2015.0834
-
BookRecherches Paléohistologiques Sur Les Os Longs Des Tétrapodes: Titanosuchiens, Dinocéphales Et DicynodontesMasson.
-
Quelques remarques sur l’histoire évolutive des tissus squelettiques chez les Vertébrés et plus particulièrement chez les TétrapodesAnnée Biologique 18:1–35.
-
On the bone histology of some triassic pseudosuchian archosaurs and related taxaAnnales De Paléontologie 89:67–101.https://doi.org/10.1016/S0753-3969(03)00005-3
-
Ventilation and gas exchange during treadmill locomotion in the american Alligator (Alligator mississippiensis)The Journal of Experimental Biology 203:1671–1678.
-
Parental care: the key to understanding endothermy and other convergent features in Birds and MammalsThe American Naturalist 155:326–334.https://doi.org/10.1086/303323
-
18o/16O ratio measurements of inorganic and organic materials by elemental analysis-pyrolysis-isotope ratio mass spectrometry continuous-flow techniquesRapid Communications in Mass Spectrometry 25:2691–2696.https://doi.org/10.1002/rcm.5056
-
Phylogeny and evolution of the Gorgonopsia with a Special Reference to the Skull and Skeleton of GPIT/RE/7113 ('Aelurognathus?’parringtoni)Universität Tübingen.
-
A threefold subdivision of the Cynognathus Assemblage Zone (Beaufort Group, South Africa) and its palaeogeographical implicationsSouth African Journal of Science 91:143–144.
-
The evolution of endothermy in terrestrial vertebrates: who? when? why?Physiological and Biochemical Zoology 77:1019–1042.https://doi.org/10.1086/425185
-
The role of foraging Mode in the origin of Therapsids: implications for the origin of mammalian endothermyFieldiana Life and Earth Sciences 5:126–148.https://doi.org/10.3158/2158-5520-5.1.126
-
Tetrapods from the east european placket—Late Paleozoic natural territorial complexTr. Paleontol. Inst. Ross. Akad. Nauk 283:1–200.
-
Continental Permian and Triassic vertebrate localities from Algeria and Morocco and their stratigraphical correlationsJournal of African Earth Sciences 29:219–226.https://doi.org/10.1016/S0899-5362(99)00091-3
-
Design of heterothermic muscle in fishThe Journal of Experimental Biology 205:2251–2266.
-
The origin of mammalian endothermy: a paradigm for the evolution of complex biological structureZoological Journal of the Linnean Society 147:473–488.https://doi.org/10.1111/j.1096-3642.2006.00226.x
-
The origin and early radiation of the therapsid mammal-like reptiles: a palaeobiological hypothesisJournal of Evolutionary Biology 19:1231–1247.https://doi.org/10.1111/j.1420-9101.2005.01076.x
-
BookThe origin and radiation of TherapsidsIn: Chinsamy-Turan A, editors. Forerunners of Mammals: Radiation, Histology, Biology. Bloomington: Indiana University Press. pp. 3–28.
-
Global variation in thermal tolerances and vulnerability of endotherms to climate changeProceedings of the Royal Society B: Biological Sciences 281:20141097.https://doi.org/10.1098/rspb.2014.1097
-
Review of the tetrapod fauna of the “Lower Stormberg Group” of the main Karoo Basin (southern Africa) : implication for the age of the Lower Elliot FormationBulletin De La Societe Geologique De France 175:73–83.https://doi.org/10.2113/175.1.73
-
The effects of Sample treatment and diagenesis on the Isotopic integrity of Carbonate in Biogenic HydroxylapatiteJournal of Archaeological Science 24:417–429.https://doi.org/10.1006/jasc.1996.0126
-
Predicting animal δ18O: Accounting for diet and physiological adaptationGeochimica Et Cosmochimica Acta 60:4811–4829.https://doi.org/10.1016/S0016-7037(96)00240-2
-
Evolution of bone microanatomy of the tetrapod tibia and its use in palaeobiological inferenceJournal of Evolutionary Biology 21:807–826.https://doi.org/10.1111/j.1420-9101.2008.01512.x
-
A taxonomic revision of pareiasaurian reptiles: implications for Permian terrestrial palaeoecologyModern Geology 21:231–298.
-
Palaeohistological evidence for ancestral high metabolic rate in ArchosaursSystematic Biology 65:989–996.https://doi.org/10.1093/sysbio/syw033
-
High-precision determination of 18O/16O ratios of silver phosphate by EA-pyrolysis-IRMS continuous flow techniqueJournal of Mass Spectrometry 42:36–41.https://doi.org/10.1002/jms.1130
-
Oxygen isotope analysis of phosphateHandbook of Stable Isotope Analytical Techniques 1:482–499.
-
SHRIMP U-Pb zircon dating of the Triassic Ermaying and Tongchuan formations in Shanxi, China and its stratigraphic implicationsVert PalAs 51:162–168.
-
New basal synapsid supports laurasian origin for therapsidsActa Palaeontologica Polonica 54:393–400.https://doi.org/10.4202/app.2008.0071
-
Dashankou Fauna: a unique window on the early evolution of therapsidsBulletin of the Chinese Academy of Sciences 24:83–85.
-
Fractionation of oxygen isotopes between mammalian bone-phosphate and environmental drinking waterGeochimica Et Cosmochimica Acta 48:1689–1693.https://doi.org/10.1016/0016-7037(84)90338-7
-
Paleohistology and histovariability of the Permian stereospondyl RhinesuchusJournal of Vertebrate Paleontology 34:59–68.https://doi.org/10.1080/02724634.2013.787429
-
The effect of large body size on the temperature regulation of the Komodo Dragon, Varanus komodoensisComparative Biochemistry and Physiology Part A: Physiology 55:345–350.https://doi.org/10.1016/0300-9629(76)90058-X
-
The evolution of Endothermy in the Phylogeny of MammalsThe American Naturalist 112:1–21.https://doi.org/10.1086/283249
-
Paleomagnetic results from the Late Permian redbeds along the Hexi Corridor, ChinaChinese Science Bulletin = Kexue Tongbao pp. 1452–1456.
-
Motion of Africa and Adria since the Permian: paleomagnetic and paleoclimatic constraints from northern LibyaEarth and Planetary Science Letters 192:159–174.https://doi.org/10.1016/S0012-821X(01)00439-3
-
First palaeohistological inference of resting metabolic rate in an extinct synapsid, moghreberia nmachouensis (Therapsida: anomodontia)Biological Journal of the Linnean Society 121:409–419.https://doi.org/10.1093/biolinnean/blw044
-
Great Transformations in Vertebrate Evolution143–165, Respiratory turbinates and the evolution of endothermy in mammals and birds, Great Transformations in Vertebrate Evolution, Chicago, University of Chicago Press.
-
Temperature variation makes ectotherms more sensitive to climate changeGlobal Change Biology 19:2373–2380.https://doi.org/10.1111/gcb.12240
-
Bone histology and growth patterns of some nonmammalian therapsidsJournal of Vertebrate Paleontology 24:634–648.https://doi.org/10.1671/0272-4634(2004)024[0634:BHAGPO]2.0.CO;2
-
BookForerunners of Mammals: Radiation, Histology, BiologyChinsamy-Turan A, editors. Bloomington: Indiana University Press.
-
Selective factors associated with the origin of Fur and FeathersAmerican Zoologist 40:585–596.https://doi.org/10.1093/icb/40.4.585
-
27th Du Toit Memorial Lecture: Re-uniting lost continents - Fossil reptiles from the ancient Karoo and their wanderlustSouth African Journal of Geology 108:135–172.https://doi.org/10.2113/108.1.135
-
The radiation of cynodonts and the ground plan of mammalian morphological diversityProceedings of the Royal Society B: Biological Sciences 280:20131865.https://doi.org/10.1098/rspb.2013.1865
-
The Capitosauria (Amphibia): characters, phylogeny, and stratigraphyPalaeodiversity 1:189–226.
-
Evidence for endothermic ancestors of crocodiles at the stem of archosaur evolutionPhysiological and Biochemical Zoology 77:1051–1067.https://doi.org/10.1086/422766
-
Long bone histology indicates sympatric species of Dimetrodon (Lower Permian, Sphenacodontidae)Earth and Environmental Science Transactions of the Royal Society of Edinburgh 103:217–236.https://doi.org/10.1017/S175569101300025X
-
Seasonal reproductive endothermy in tegu lizardsScience Advances 2:e1500951.https://doi.org/10.1126/sciadv.1500951
-
Phanerozoic polar wander, palaeogeography and dynamicsEarth-Science Reviews 114:325–368.https://doi.org/10.1016/j.earscirev.2012.06.007
-
Long-term cycles of triassic climate change: a new δ18o record from conodont apatiteEarth and Planetary Science Letters 415:165–174.https://doi.org/10.1016/j.epsl.2015.01.038
-
Isotopic composition of recent shark teeth as a proxy for environmental conditionsGeochimica Et Cosmochimica Acta 65:1583–1599.https://doi.org/10.1016/S0016-7037(00)00629-3
-
Origin and palaeoenvironmental significance of lystrosaurus bonebeds in the earliest Triassic Karoo Basin, South AfricaPalaeogeography, Palaeoclimatology, Palaeoecology 392:9–21.https://doi.org/10.1016/j.palaeo.2013.08.015
-
Tetrapod footprints from the uppermost level of the Permian Ikakern formation (Argana Basin, Western High Atlas, Morocco)Journal of African Earth Sciences 57:470–478.https://doi.org/10.1016/j.jafrearsci.2009.12.003
-
Monotreme Cell-Cycles and the evolution of HomeothermyAustralian Journal of Zoology 36:573–584.https://doi.org/10.1071/ZO9880573
-
BookDeep-Time Perspectives on Climate Change: Marrying the Signal From Computer Models and Biological ProxiesGeological Society of London.
-
New mesozoic apparent polar wander path for south China: tectonic consequencesJournal of Geophysical Research: Solid Earth 106:8493–8520.https://doi.org/10.1029/2000JB900338
-
CA-TIMS zircon U–Pb dating of felsic ignimbrite from the Binchuan section: Implications for the termination age of Emeishan large igneous provinceLithos, Special Issue Permian Large Igneous Provinces: Characteristics, Mineralization and Paleo-Environment Effects 204:14–19.
Article and author information
Author details
Funding
Institut National des Sciences de l'Univers, Centre National de la Recherche Scientifique
- Romain Amiot
Palaeontological Scientific Trust
- Bruce S Rubidge
National Research Foundation
- Bruce S Rubidge
Centre of Excellence in Palaeosciences
- Bruce S Rubidge
National Basic Research Program of China (2012CB821900)
- Romain Amiot
- Xu Wang
National Basic Research Program of China (2012CB821902)
- Jun Liu
Institut Universitaire de France
- Christophe Lécuyer
The funders had no role in study design, data collection and interpretation, or the decision to submit the work for publication.
Acknowledgements
The authors thank the MNHN, the IVPP, Iziko SA Museum and the ESI for granting access to the fossils, and the South African Heritage Resources Agency (SAHRA) for their authorizations to sample and export fossils for stable isotope analysis (PermitID: 1858). We also acknowledge S Jiquel (ISEM) for the access to the Ellenberger’s collection of the University Montpellier 2, J Falconnet (MNHN) for his identification, L. Cui (IGGCAS) for laboratory help and J Cubo (UPMC) for constructed discussions that improved the manuscript. This work was supported by a French project INSU of the CNRS, the Palaeontological Scientific Trust (PAST) and its Scatterlings of Africa programmes, National Research Foundation (NRF) and DST/NRF Centre of Excellence in Palaeosciences. AR and WX were supported by the National Basic Research Program of China (2012CB821900), and LJ by the National Basic Research Program of China (2012CB821902). We thank the four reviewers, K Angielczyk, J Eiler and two anonymous ones, for their constructive comments.
Copyright
© 2017, Rey et al.
This article is distributed under the terms of the Creative Commons Attribution License, which permits unrestricted use and redistribution provided that the original author and source are credited.
Metrics
-
- 3,307
- views
-
- 455
- downloads
-
- 45
- citations
Views, downloads and citations are aggregated across all versions of this paper published by eLife.
Citations by DOI
-
- 45
- citations for umbrella DOI https://doi.org/10.7554/eLife.28589