Dynamic filopodia are required for chemokine-dependent intracellular polarization during guided cell migration in vivo
Figures
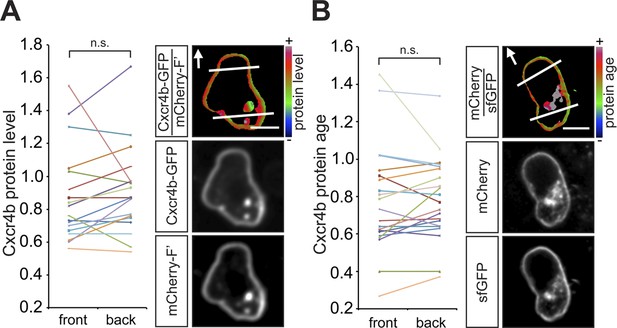
In wild type embryos the Cxcr4b receptor is uniformly distributed on the migrating PGC membrane, and its turnover is uniform around the cell circumference.
(A) A graph showing the Cxcr4b-GFP protein level measured at the front and the back (normalized to the mCherry-F') of individual migrating PGCs under conditions of endogenous Cxcl12a distribution in the embryo (A, n = 18). A representative cell is shown, with the areas defined as front and back indicated by lines and the arrows designate the migration direction. Scale bars signify 10 µm. (B) A graph showing the protein age (lifetime ratio, see Figure 1—figure supplement 1) measured at the front and the back of individual migrating PGCs under conditions of endogenous Cxcl12a distribution in the embryo (n = 20). A representative cell is shown, with the areas defined as front and back indicated by lines and the arrows designate the migration direction. Scale bars signify 10 µm.
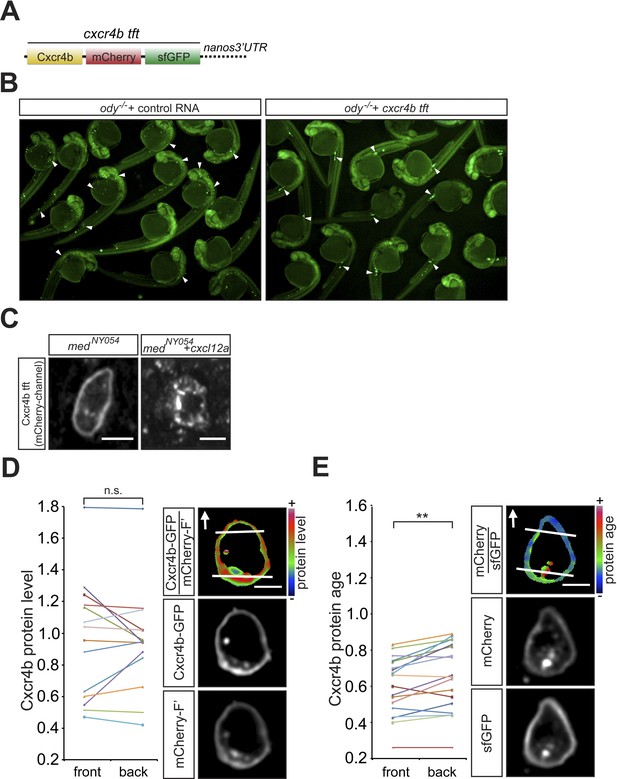
Functionality of the Cxcr4b tandem fluorescent timer (tft) in the context of PGC migration.
(A) Schematic representation of the cxcr4b tft RNA, showing the cxcr4b open reading frame cloned upstream to the slow maturing mCherry and to the fast maturing sfGFP, followed by nanos3′UTR that drives preferential expression of the protein within the PGCs. The Red to Green intensity ratio reflects the protein age such that the higher the ratio, the more mature the protein is. (B) The Cxcr4b tft protein can guide PGCs to their target in embryos lacking functional Cxcr4b (ody−/−). Arrowheads point at PGCs found in ectopic positions in 24 hr post fertilisation (hpf) ody−/− mutants (left panel) and at the target position following introduction of cxcr4b tft RNA (right panel). (C) The Cxcr4b tft protein expressed in PGCs is internalized upon exposure to Cxcl12a (right panel). The functionality of the Cxcr4b tandem fluorescent timer (tft) is evidenced by the fact that despite the uniform distribution of Cxcr4b on the cell membrane (D, n = 14), cells located within an artificially-generated steep Cxcl12a gradient exhibit reduced age at their cell front (E, n = 18). Scale bars signify 10 µm.
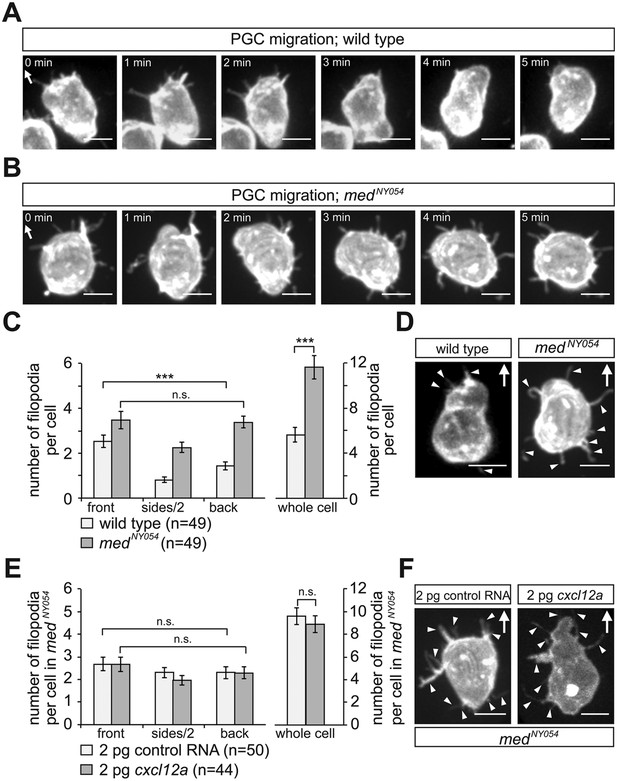
The polar positioning and number of filopodia are determined by the Cxcl12a gradient.
(A) A PGC extending filopodia during migration in wild type embryos (Video 1), and (B) in medusa (medNY054) mutant embryos lacking Cxcl12a (Video 3). (C) Filopodia distribution and number in PGCs migrating within wild type and medNY054 homozygous embryos (for segmentation see Figure 2—figure supplement 2C for more details). ‘n’ indicates the number of cells analysed. (D) Examples of a wild type (left) and a medNY054 PGC in which the characteristic distribution of the filopodia is indicated by arrowheads. (E) Filopodia number and distribution in PGCs migrating within in medNY054 homozygous embryos knocked down for Cxcr7b and that express either uniform levels of control RNA (light bars) or Cxcl12a-encoding RNA (dark bars, see also Video 4). (F) Examples of PGCs migrating within an environment lacking (left), or containing uniform Cxcl12a (right) in which the characteristic distribution of the filopodia is indicated by arrowheads. In A, B, D and F the membrane of the PGCs is labelled with EGFP-F' and arrows indicate direction of movement. Scale bar is 10 µm.
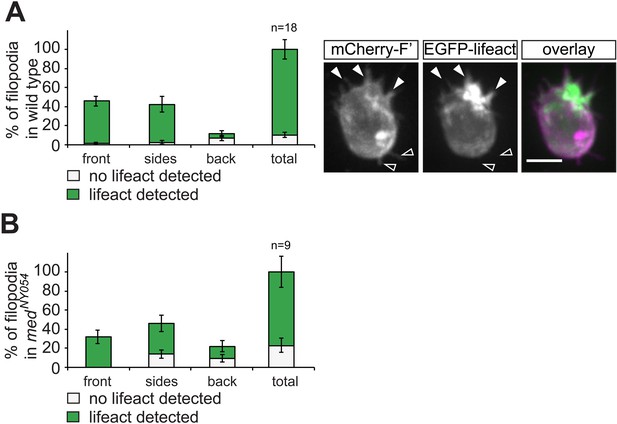
F-actin content in filopodia extended by PGCs.
(A) A graph showing the frequency of F-actin-containing filopodia in PGCs of transgenic embryos co-expressing Lifeact-EGFP and mCherry-F'. In the cell presented on the right F-actin can be detected in the filopodia formed at the front (filled arrowheads), while the empty arrows point at filopodia at the back of the cell where F-actin is not detected. ‘n’ represents the number of cells analysed. (B) A graph showing the frequency of F-actin-containing filopodia (determined as presented in A) that are formed by PGCs in medNY054 embryos. ‘n’ represents the number of cells analysed.
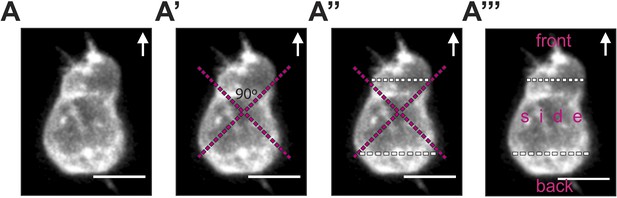
The procedure for cell segmentation, defining the front, back and sides of the cell.
(A) The segmentation process is performed on a 3D reconstructed image of a polarized PGC labelled with EGFP-F' and imaged by a spinning-disk microscope. (A′) Two orthogonal lines are drawn, with their cross positioned at the centre of the cell. The orientation of the cross relates to the direction of migration and to the morphological axis of the cell, such that one right angle points at the front and the other to the back. (A″) The positions where the lines of the cross meet the cell membrane are connected by the white segmentation lines at the front and the back. (A‴) 3D analysis of filopodia distribution and number in the three areas (front, side and back) is conducted while turning the cell in all dimensions. Arrows indicate the direction of movement. Scale bars signify 10 µm.
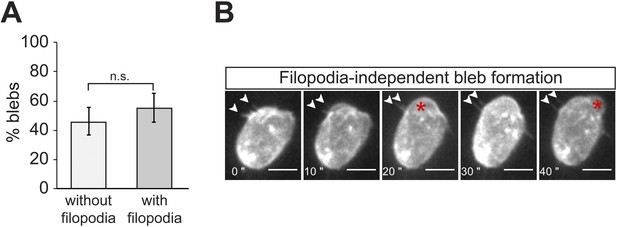
Filopodia do not appear to play an essential direct role in the generation of blebs.
(A) A graph showing the percentage of blebs forming relative to filopodia position (without filopodia = no filopodia on top or immediately next to bleb; with filopodia = filopodia on top or immediately next to bleb). (B) Panels presenting individual snapshots from Video 2, where a first bleb (asterisk) is formed in a close proximity to filopodia (arrowheads, at 10–20″) and a second is formed in a region devoid of filopodia (40″). Filopodia persist as a bleb inflates in the immediate vicinity (arrowheads, 10–20″). Scale bar is 10 µm.
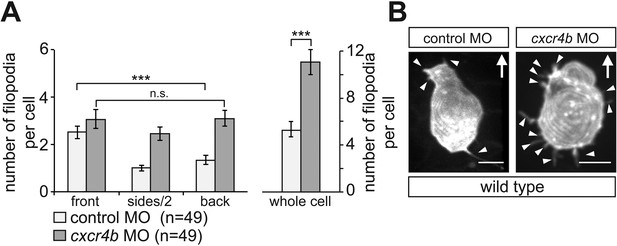
Filopodia distribution and number in PGCs knocked down for Cxcr4b.
(A) PGCs in embryos knocked down for Cxcr4b (dark bars) show apolar distribution of filopodia and an overall increased formation of these cellular protrusions as compared with control cells (light bars). (B) Representative examples of a control (left) and Cxcr4b-knocked down PGCs. Arrowheads mark filopodia and ‘n’ indicates the number of cells analysed. Arrows show the direction of movement. Scale bars signify 10 µm.
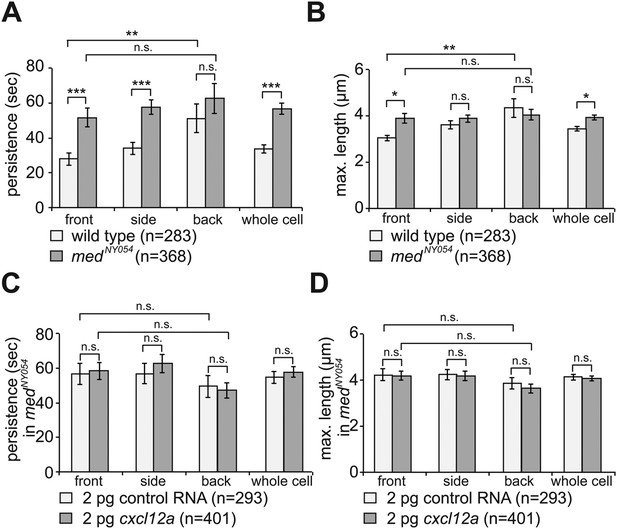
The dynamics of filopodia at the cell front are determined by the distribution of Cxcl12a.
(A) Persistence and (B) maximum length of filopodia in PGCs migrating within wild type (light bars) and medNY054 homozygous embryos (dark bars). ‘n’ indicates the number of filopodia analysed in 10 wild type cells and 8 medNY054cells over 10 min. (C) Persistence and (D) maximum length of filopodia in PGCs migrating within medNY054 homozygous embryos knocked down for Cxcr7b and injected with 2 pg control mRNA (light bars) or 2 pg cxcl12a mRNA (dark bars). ‘n’ indicates the number of filopodia in 10 cells over 10 min.
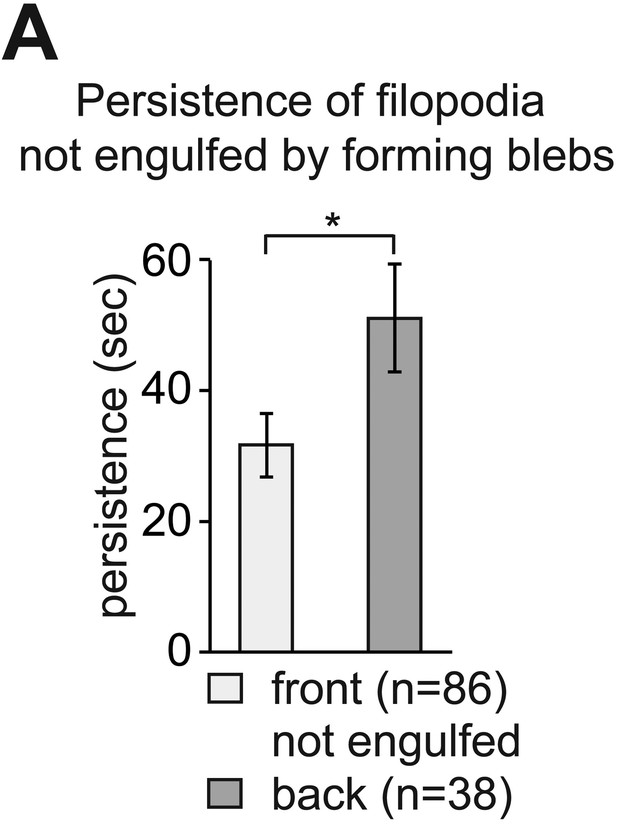
The enhanced dynamics of filopodia at the cell front is independent of bleb formation at this aspect of the cell.
(A) Persistence of filopodia at the cell front, which are not engulfed by blebs, compared to that of filopodia at the back of PGCs migrating within wild type embryos (from dataset used in Figure 3). ‘n’ represents number of filopodia analysed.
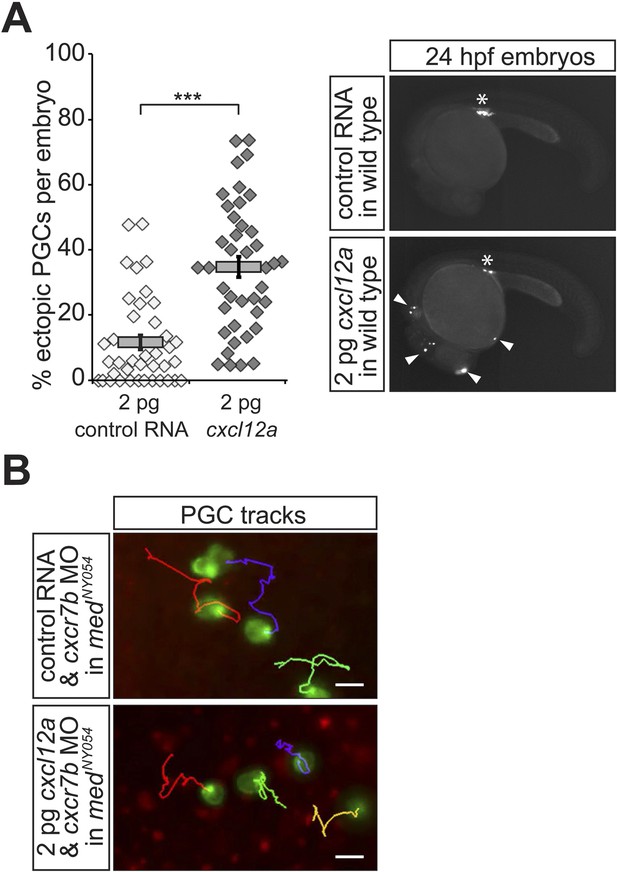
Properties of PGCs migrating in embryos expressing a low concentration of uniform Cxcl12a.
(A) Injection of 2 pg of Cxcl12a-encoding RNA into embryos results in an increase in the proportion of the ectopic PGCs per embryo at 24 hpf. Representative embryos are shown on the right. An asterisk labels the site where the gonad develops; ectopic PGCs are labelled with arrowheads. 39 control and 40 embryos expressing 2 pg Cxcl12a were analysed. (B) Migration tracks of PGCs in medNY054 homozygous embryos knocked down for Cxcr7b and injected with 2 pg control mRNA (upper panel) or 2 pg cxcl12a mRNA (lower panel). Tracks represent 58 min of PGC migration in 7 hpf embryos acquired at a 2 min interval (Video 4).
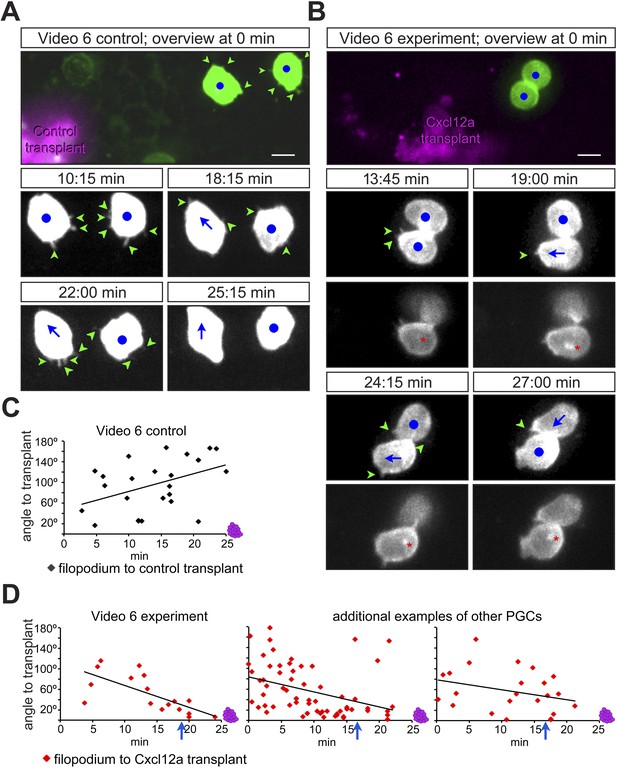
PGCs extend filopodia towards the chemokine source prior to cell polarization and directed migration towards the attractant.
(A, B) The cellular behaviour of PGCs (green) in response to transplanted control cells (magenta in A) or to Cxcl12a-expressing cells (magenta in B). Upper panels show the cells immediately after transplantation and lower panels show snapshots from Video 6 presenting the behaviour of the cells in the following 28 min. In B, additional images present the polar position of the golgi (red asterisk, labelled by EGFP-F', as defined in Figure 4—figure supplement 1A) at the back of the cell. Green arrowheads mark filopodia, blue dots indicate no migration and blue arrows show the direction of PGC movement. Scale bar is 10 µm. (C, D) The angle of filopodia orientation relative to the position of transplanted cells (located at 0°, see also Figure 4—figure supplement 1B) in the case of a control transplant (C) and with respect to Cxcl12a expressing transplant (D, three examples) over 25 min. Blue arrows signify the time of morphological cell polarization and movement.
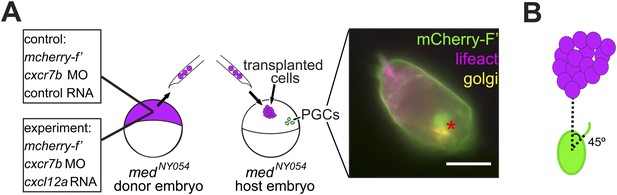
Polarization of PGCs encountering an artificially generated Cxcl12a gradient.
(A) Schematic experimental setup. Cells from 4 hpf medNY054 homozygous embryos expressing Cxcl12a and mCherry-F', in which Cxcr7b expression was inhibited, were transplanted into 6 hpf medNY054 homozygous embryos to examine the response of PGCs to the chemokine gradient. Control cells were similarly labelled but lacked Cxcl12a expression. The image to the right shows a polarized PGC with F-actin at the cell front labelled by Lifeact-EGFP (magenta) and the golgi apparatus at the cell back labelled with ECFP- tagged human beta1,-4-galactosyltransferase (yellow). The mCherry-F' labels the cell membrane, as well as the area of the golgi at the back of the cell. (B) A schematic representation of the angle measurements presented in Figure 4C,D for filopodia orientation relative to the position of the transplanted cells (located at 0°).
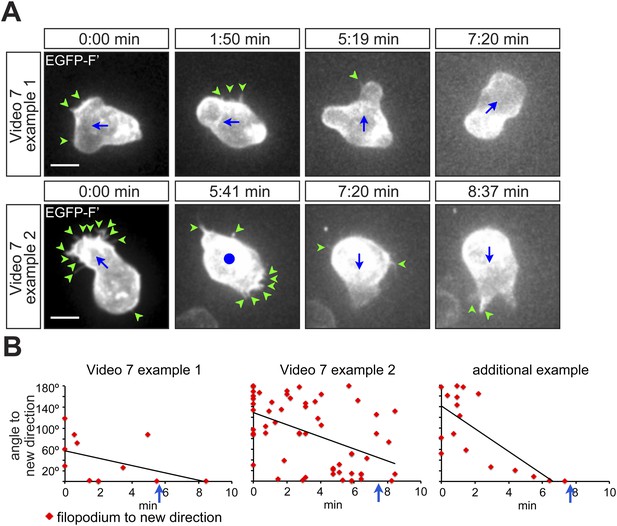
PGCs extend filopodia in the direction of migration prior to polarization and actual onset of migration.
(A) Snapshots from Video 7 presenting the behaviour of a migrating cell, which makes a 90° turn (upper panel) and of a cell, which depolarizes and then migrates in the opposite direction (lower panel). Green arrowheads mark filopodia; blue dot indicates no migration and blue arrows show the direction of PGC movement. Scale bar signifies 10 µm. (B) Filopodia orientation relative to the forthcoming direction of migration (located at 0°) in the two cells presented in Video 7. An additional example is presented on the right. Blue arrows signify the time of morphological cell polarization and migration.
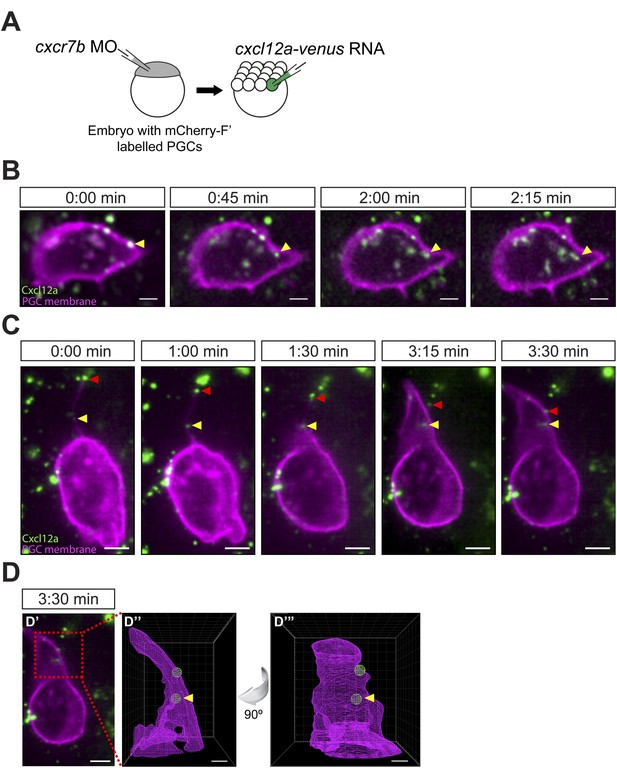
Cxcl12a internalization and interaction with filopodia.
(A) Schematic experimental setup. Cxcr7b function was knocked down in embryos, in which PGCs express mCherry on their membrane. At 16-cell stage, these embryos were injected with cxcl12a-venus RNA directed into a corner cell for a mosaic expression of the chemokine. (B) Cxcl12a (green) is bound to the PGC membrane (magenta) and internalizes into the cell. Snapshots from Video 8, showing an optical section of a PGC (a Z-projection of two 1-µm-slices). An arrowhead points at an internalizing Cxcl12a spot. Scale bar is 5 µm. (C) Snapshots from Video 9, showing an optical section of a PGC (a Z-projection of 4 1-µm-slices) with Cxcl12a (green) interaction seen on the filopodium (magenta). The red arrowhead indicates a Cxcl12a spot bound to the tip of the retracting filopodium and the yellow arrowhead points at Cxcl12a, which is bound to the filopodium closer to the cell body and is then engulfed by the cell (1:30–3:30 min). Scale bar is 5 µm. (D) PGC from panel C at 3:30 min (D′) where the area magnified in D″ and D‴ is delineated in a red box. (D″ and D‴) A 3D wire presentation of the magnified cell surface area (magenta) and the relevant Cxcl12 foci (green). (D″) A 3D image orientated as the original panel in C and (D‴) is horizontally rotated (90° clockwise) to visualize internalization of Cxcl12a. Scale bar is 2 µm.
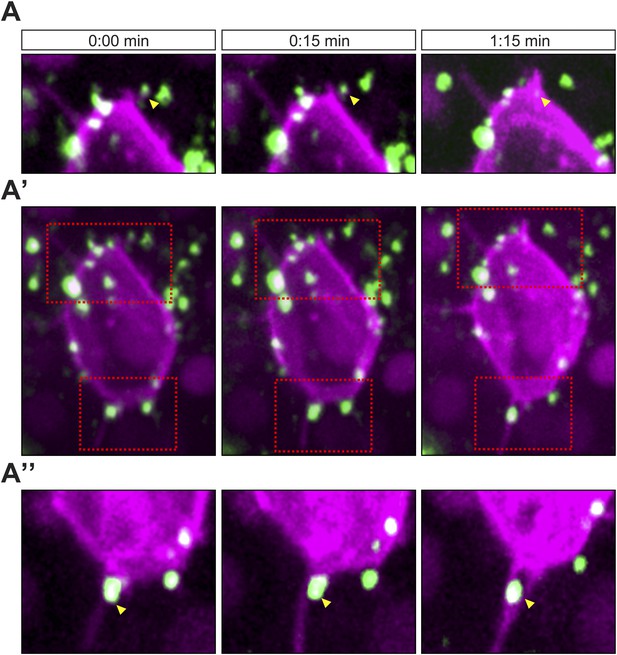
Cxcl12a interaction with filopodia at the front and back of the cell.
(A–A″) Cxcl12a (Venus-tagged, green) interacts with filopodia of a PGC (mCherry-F, magenta). (A′) An optical section of a PGC (a Z-projection of 4 1-µm-slices) with Cxcl12a (green) interaction with filopodia (magenta) observed over 1:15 min. (A and A″) Magnified insets marked in A′ as red squares. The yellow arrowheads point at Cxcl12a spots along filopodia.
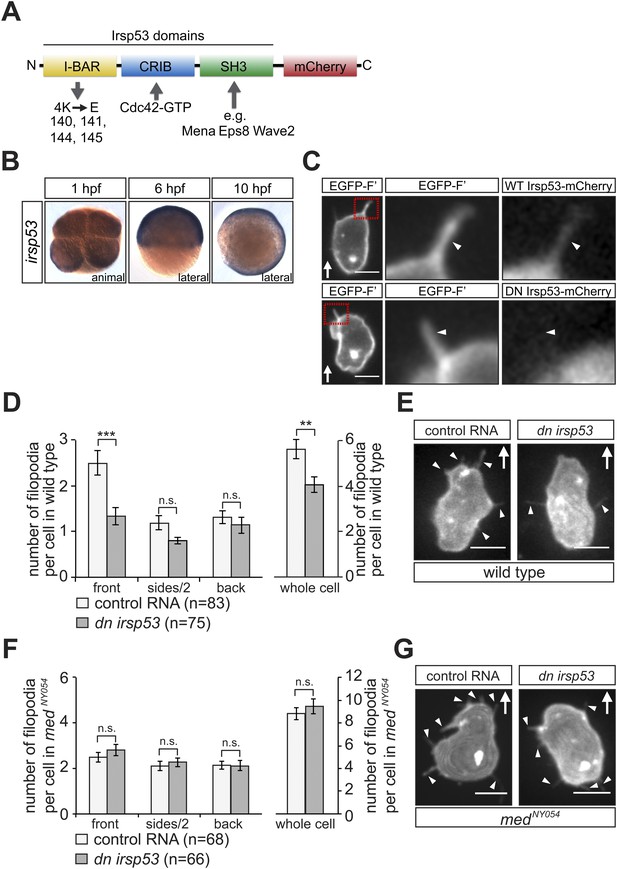
irsp53 RNA expression, Irsp53 protein localization and the role of the protein in filopodia formation.
(A) Schematic structure of the Irsp53 protein domains. The position of the mCherry fluorophore fusion at the C-terminus of the protein is presented, proteins interacting with the SH3 and CRIB domains are indicated and the mutations introduced into the I-BAR domain to generate the dominant-negative (DN) Irsp53 version are marked. (B) Ubiquitous expression of the irsp53 RNA in 1, 6 and 10-hpf embryos. (C) A single plane of PGCs expressing EGFP-F' and an Irsp53-mCherry protein fusion showing localization of Irsp53 to filopodia (upper panel), while the dominant-negative Irsp53 protein is not found in the filopodia (lower panel). Rectangles delineate the area of magnification shown in the right panels. (D) Reduction of filopodia number at the cell front in PGCs in wild type embryos expressing the DN Irsp53 protein (dark bars) relative to control PGCs (light bars). ‘n’ indicates the number of cells analysed. (E) Examples of a control (left) and a dn irsp53-expressing (right) PGCs in wild type embryos. (F) Expression of dn irsp53 in PGCs of medNY054 homozygous embryos shows no effect on filopodia number and distribution around the cell perimeter (dark bars) as compared with control PGCs (light bars). (G) Examples of a control (left) and a dn irsp53-expressing (right) PGCs in medNY054 homozygous embryos. ‘n’ indicates the number of cells analysed, arrows show direction of cell migration and arrowheads mark filopodia. Scale bar is 10 µm.
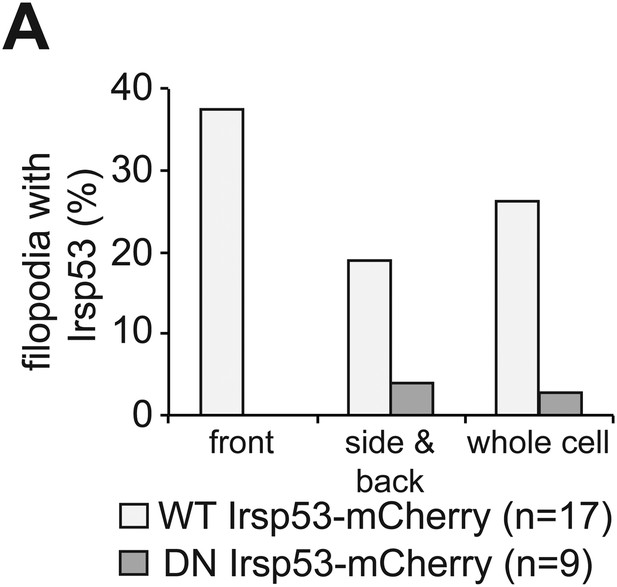
Irsp53 localization within filopodia.
(A) Irsp53 can be detected in a higher proportion of filopodia at the cell front than within filopodia at the side and back of the cell. The dominant-negative version of Irsp53-mCherry cannot be detected within front filopodia and can be observed in a small proportion of side and back filopodia. ‘n’ signifies the number of cells analysed.
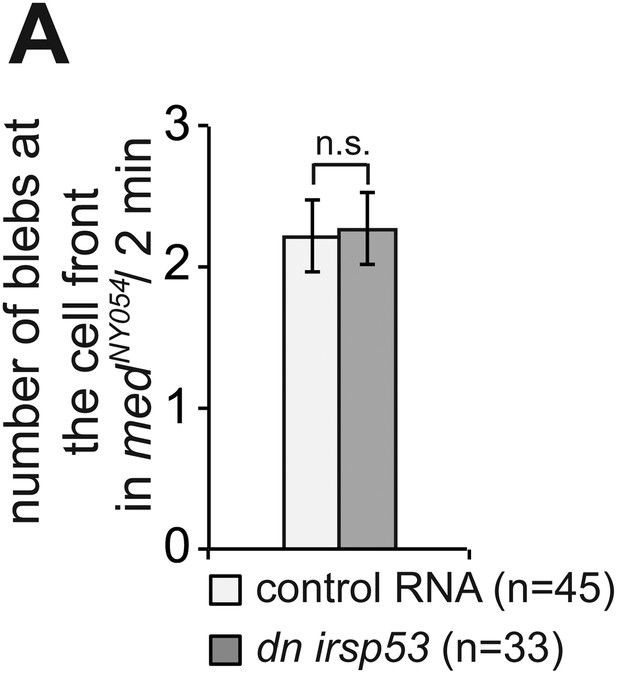
Inhibition of Irsp53 does not affect bleb formation at the cell front.
(A) Number of Cxcl12a-independent blebs formed by PGCs expressing a dominant-negative version of irsp53, as compared with the number of blebs formed by control cells in medNY054 homozygous embryos. ‘n’ signifies the number of cells analysed.
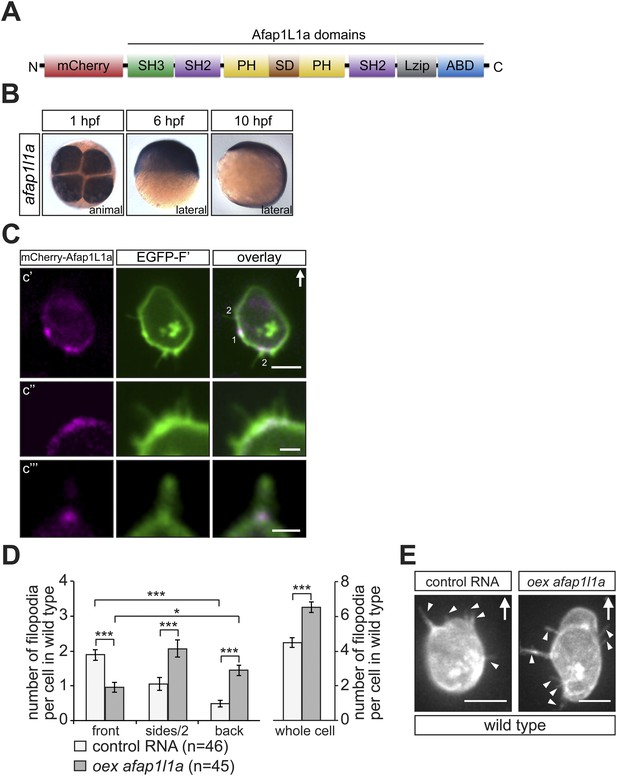
afap1l1a RNA expression, Afap1L1a protein localization and the role of the protein in filopodia formation.
(A) Schematic structure of the Afap1L1a protein domains including a serine-threonine-rich substrate domain (SD) flanked by two PH domains, a leucine zipper (Lzip), an actin-binding domain (ABD), two SH2 and one SH3 domains. mCherry fluorophore was fused to the N-terminus of Afap1L1a to determine the subcellular localization of the protein. (B) Ubiquitous expression of the afap1l1a RNA in 1, 6 and 10-hpf embryos. (C) A single plane of PGCs expressing EGFP-F' and the mCherry-Afap1L1a fusion protein reveals weak expression of the protein around the cell perimeter (C′) and a predominant strong association with the base of filopodia (C′–C‴). In C′, one marks the site of a retracted filopodium, two the sites of extended filopodia and arrow indicates the direction of migration. (D) The number and distribution of filopodia in migrating PGCs overexpressing afap1l1a (dark bars) as compared with control PGCs (light bars). ‘n’ indicates the number of cells analysed. (E) Representative images of control (left) and of afap1l1a-overexpressing PGCs. Arrows indicate the direction of movement and arrowheads point at filopodia. Scale bars signify 10 µm except for C″ and C‴, where the scale is 3 µm.
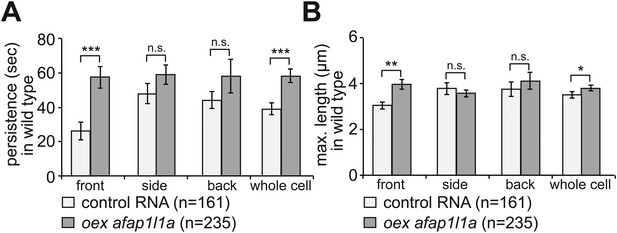
The effect of Afap1L1a overexpression on filopodia dynamics in PGCs migrating within a wild type environment.
(A) Persistence and (B) maximum length of filopodia in PGCs that express control RNA (light bars) or overexpress afap1l1a (dark bars). ‘n’ signifies the number of filopodia analysed in six cells during 10 min.
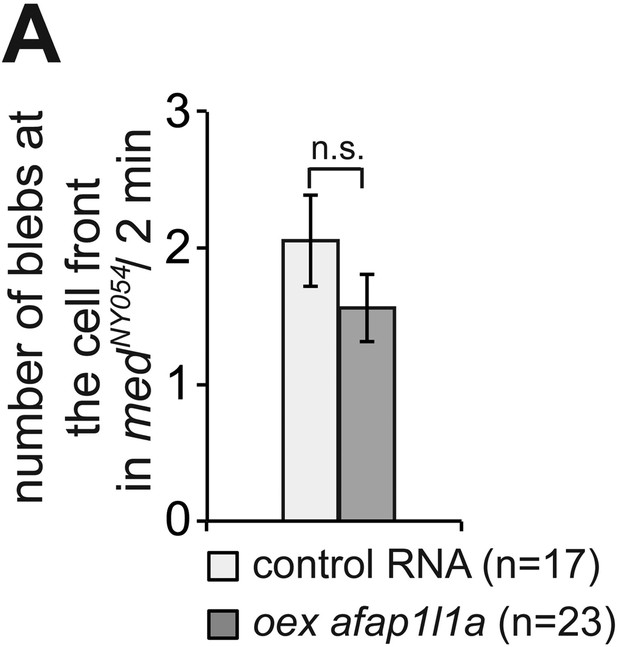
Afap1L1a overexpression does not affect bleb formation at the cell front.
(A) Number of Cxcl12a-independent bleb formation by PGCs overexpressing afap1L1a, as compared with control cells in medNY054 homozygous embryos. ‘n’ signifies the number of cells analysed.
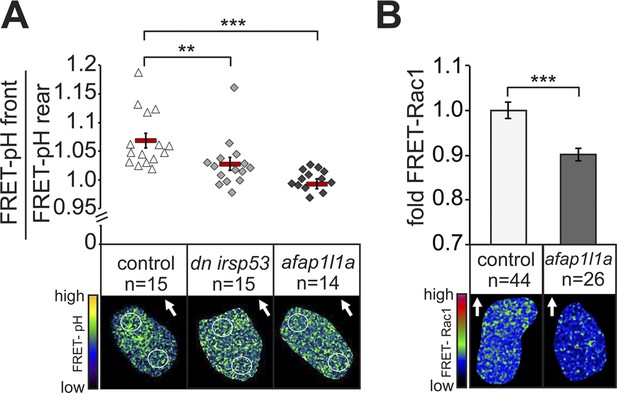
Filopodia are required for cellular response to polarized Cxcl12a distribution.
(A) 6–7 hpf control PGCs exhibit polarized distribution of intracellular pH as determined by the FRET efficiency of the pH sensor pH-lameleon5 protein in the cells (left). Expression of the dominant negative form of Irsp53 or overexpression of the Afap1L1a abrogates the formation of high pH in the front. The graph represents average pH-FRET ratios between the front and the rear (as indicated by the circles). The values for each cell are averages of 20 time points. (B) 8–9 hpf PGCs overexpressing Afap1L1a exhibit a decrease in Rac1 activity, as determined by differences in FRET generated by a Rac1-FRET activity reporter. Arrows indicate the direction of movement. ‘n’ indicates the number of cells analysed.
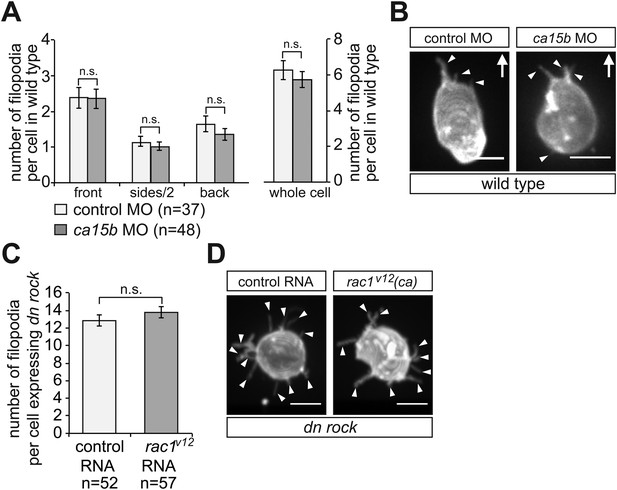
Filopodia formation is independent of the elevated pH at the cell front and of Rac1 activity in migrating PGCs.
(A) PGCs in embryos knocked down for ca15b (dark bars) show similar distribution and number of filopodia to that of control cells (light bars). (B) Examples of a control (left) and ca15b -knocked down PGCs. Arrows indicate the direction of movement. (C) The number of filopodia in control (light bar) and in PGCs expressing constitutive active (ca) rac1 (rac1V12) (dark bar) is similar. Since cells expressing the activated version of Rac1 are immotile, we immobilized both control and experimental cells by DN Rock expression, so the cells can be compared with respect to filopodia formation. (D) Representative images of a control (left) and a rac1V12 expressing PGCs. Arrowheads indicate filopodia. ‘n’ indicates the number of cells analysed. Scale bars signify 10 µm.
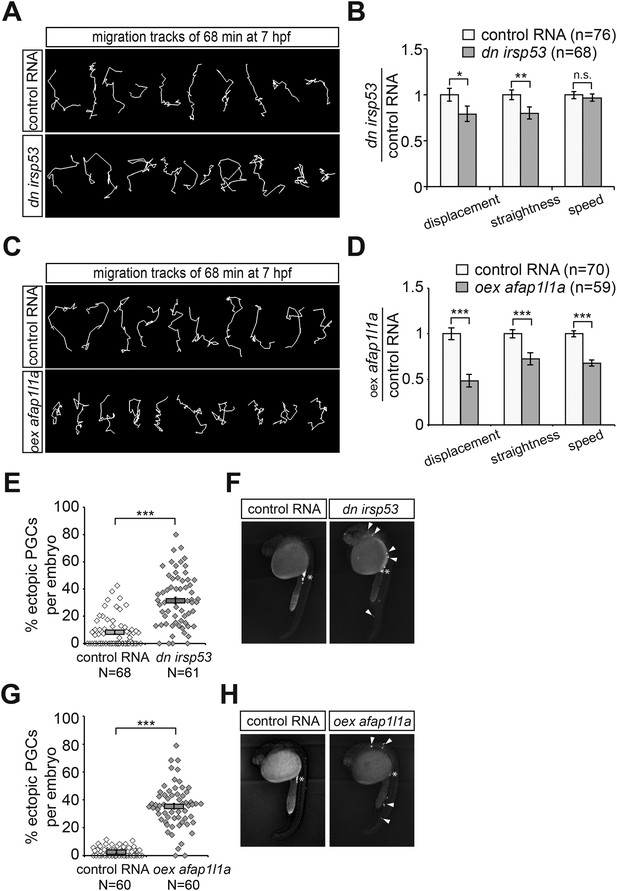
Manipulations of filopodia formation lead to PGC migration defects.
(A) Representative migration tracks of control PGCs (upper panel) and PGCs expressing the dominant-negative (dn) irsp53 version (lower panel). (B) Analysis of PGC migration tracks assessing displacement, straightness and migration speed, comparing control cells (light bars) with dn Irsp53-expressing cells (dark bars). ‘n’ indicates the number of migration tracks analysed. (C) Representative migration tracks of control PGCs (upper panel) and PGCs overexpressing (oex) afap1L1a (lower panel). (D) Analysis of PGC displacement, track straightness and migration speed, comparing control cells (light bars) with afap1l1a overexpressing cells (dark bars). ‘n’ indicates the number of migration tracks analysed. (E–H) Expression of the dn irsp53 (E, F) or overexpressing afap1l1a (G, H) in PGCs results in an increase of ectopic cells at 24 hpf as compared with embryos whose PGCs express a control RNA. ‘n’ indicates the number of embryos analysed. Arrowheads point at ectopic PGCs and asterisks mark the site of the developing gonad.
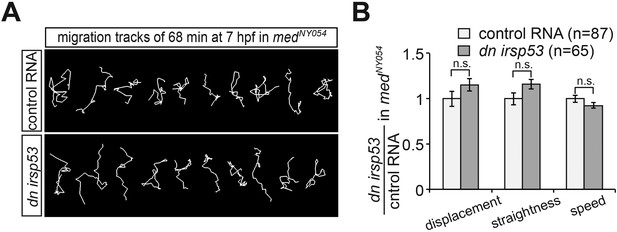
Reducing the activity of Irsp53 has no effect on cell migration in the absence of Cxcl12a.
(A) Representative migration tracks of control PGCs (upper panel) and PGCs expressing the dominant-negative Irsp53 version (lower panel) in medNY054 homozygous embryos. (B) Analysis of PGC migration tracks assessing displacement, track straightness and speed. Control cells (light bars) are compared with dn irsp53-expressing cells (dark bars) migrating in medNY054 homozygous embryos. ‘n’ indicates the number of migration tracks analysed.
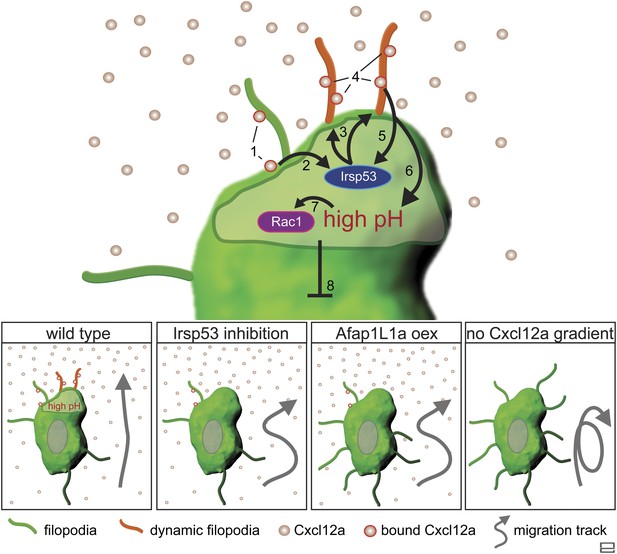
Regulation of dynamic filopodia at the cell front and their role in cell polarization and directed cell migration.
Following the activation of Cxcr4b by Cxcl12a (1), the scaffold protein Irsp53 is activated (2) and promotes the formation of dynamic filopodia at the cell front (3). The dynamic filopodia that extend in the direction of higher Cxcl12a concentration increase the surface for chemokine binding (4), thereby enhancing signalling. The enhanced signalling at the cell front results in further Irsp53 activation (5) and an elevation of pH (6). The increase in pH in turn, leads to an elevation in Rac1 activity at this aspect of the cell (7). Once established, the front inhibits other parts of the cell from assuming front characteristics including dynamic filopodia formation, elevated pH and Rac1 activation (8). Lower panels—Irsp53 inhibition or Afap1L1a overexpression result in loss of dynamic filopodia at the cell front, abrogating the local increase in pH. As a consequence, PGCs migrate less directionally. Lack of a Cxcl12a gradient leads to the formation of numerous, evenly distributed, long and persistent filopodia in cells that migrate in random directions.
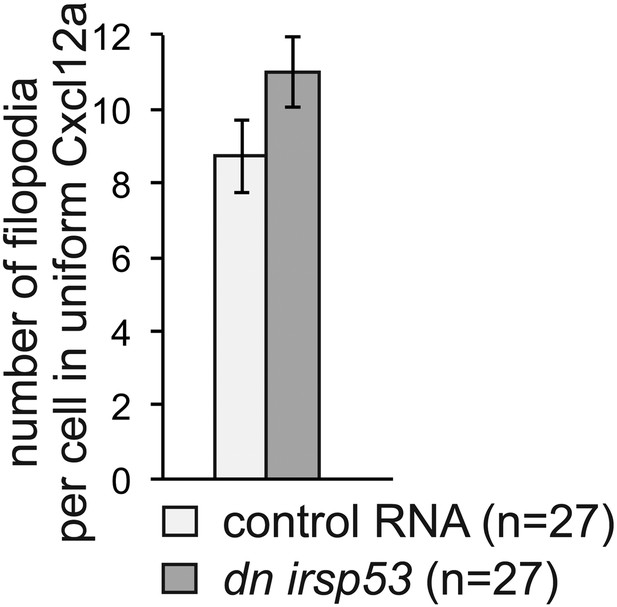
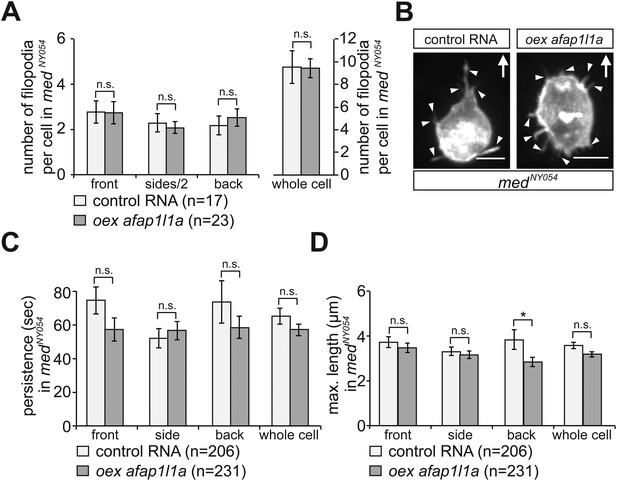
Videos
Migrating PGCs in wild type embryos show enhanced formation of filopodia in the direction of migration.
A 10 min time-lapse video of a PGC in kop-egfp-f'nos3′UTR embryo was captured using a 63× objective on a Zeiss AxioImager.M2 microscope equipped with a Photometrics camera (Cascade II) and VS-Laser Control. Z-stacks include 29 planes per time point at focal planes 1 µm apart, 10 s interval, 300 ms exposure time with binning one. Time in minutes and seconds. Scale bar represents 10 µm.
Filopodia are not directly controlling bleb formation.
Bleb-formation (asterisk) can be observed first in close proximity to filopodia (arrowheads, 10–20″) and then in a region devoid of filopodia (40″). A 40-s time-lapse video of a membrane-labelled PGC (in a kop-egfp-f'nos3′UTR embryo) using a 63× objective on a Zeiss AxioImager.M2 microscope equipped with a dual view filter (MAG Biosystems), Photometrics camera (Cascade II) and VS-Laser Control. Z-stacks were captured with 29 planes at focal planes per time point, 1 µm apart at 10 s interval, 300 ms exposure time and with binning one. Scale bar represents 10 µm.
Migrating PGCs in medusa (medNY054) mutant embryos that lack Cxcl12a show enhanced apolar filopodia formation.
A 10 min time-lapse video of a PGC in medNY054 homozygous embryo was captured using a 63× objective on a Zeiss AxioImager.M2 microscope equipped with a Photometrics camera (Cascade II) and VS-Laser Control. Z-stacks include 29 planes per time point at focal planes 1 µm apart, 10 s interval, 300 ms exposure time with binning one. Time in minutes and seconds. Scale bar represents 10 µm.
Low concentration of uniformly expressed Cxcl12a affects cell migration, but does not immobilize the cells.
Migration tracks of PGCs in medNY054 homozygous embryos knocked down for Cxcr7b, injected with mcherry_h2b_globin3′UTR mRNA (mCherry labelling nuclei of all cells) and either with 2 pg control mRNA (first video) or 2 pg cxcl12a mRNA (second video). Tracks represent 58 min of development in 7 hpf embryos. Snapshots captured at 2-min intervals with 300 ms exposure time at three focal planes (15 µm apart) using a 10× objective to generate the Z-stacks on a Zeiss AxioImager.M1 microscope equipped with a Photometrics camera (CoolSNAP ES2). Time in minutes and scale bar represents 50 µm.
Uniform Cxcl12a in the environment induces apolar formation of numerous long and persisting filopodia on the PGC surface.
A 10 min time-lapse video of a PGC in medNY054 homozygous embryo knocked down for Cxcr7b and that expresses uniform levels of Cxcl12a-encoding RNA was captured using a 63× objective on a Zeiss AxioImager.M2 microscope equipped with a Photometrics camera (Cascade II) and VS-Laser Control. Z-stacks include 29 planes per time point at focal planes 1 µm apart, 10 s interval, 300 ms exposure time with binning one. Time in minutes and seconds. Scale bar represents 10 µm.
PGCs extend filopodia towards the chemokine source, prior to their morphological polarization and directed migration towards cells producing the attractant.
Cells from 4 hpf medNY054 homozygous embryos, in which Cxcr7b expression was inhibited and which express mCherry-F' (red) and either control RNA (part control transplant) or express cxcl12a RNA (part Cxcl12a transplant) were transplanted into 6 hpf host medNY054 homozygous embryos. The PGCs in the host embryos were labelled with EGFP-F' and their reaction to the transplant was monitored on a Zeiss AxioImager.M1 microscope equipped with a dual view filter (MAG Biosystems), Photometrics camera (Cascade II) and VS-Laser Control. Acquisition of the 28 min time-lapse video started immediately following the transplantation using a 40× objective. Z-stacks were captured with 24 planes per time point, at focal planes 4 µm apart, 15 s interval, 300 ms exposure time with binning one. Green arrowheads mark the position of filopodia, red asterisks mark the position of the golgi, blue dots indicate no migration and blue arrows indicate morphological PGC polarization and movement. Time in minutes and scale bar represents 10 µm.
PGCs extend filopodia in the direction of migration, prior to polarization and actual onset of migration.
10 min time-lapse videos of PGCs in wild type embryos were captured using a 63× objective on a Zeiss AxioImager.M2 microscope equipped with a Photometrics camera (Cascade II) and VS-Laser Control. Z-stacks include 29 planes per time point at focal planes 1 µm apart, 11 s interval, 300 ms exposure time with binning one. Time in minutes and seconds. Scale bar represents 10 µm.
Cxcl12a internalization.
Cxcl12a (green) is bound to the PGC membrane (magenta) and internalizes into the cell. The video was captured using a 63× objective on a Zeiss AxioImager.M2 microscope equipped with a Photometrics camera (Cascade II) and VS-Laser Control at 15 s intervals for 2:15 min and shows an optical section of a PGC (a Z-projection of two 1-µm-slices). Arrows indicate a bound and an internalizing Cxcl12a spot. Scale bar is 5 µm.
Cxcl12a interaction with filopodia.
Cxcl12a (green) is bound to a filopodium and internalizes into the cell. An optical section of a PGC (a Z-projection of 4 1-µm-slices) is shown with Cxcl12a (green) interaction observed on the filopodium (magenta). The video was captured with a Lightsheet Z1 microscope (Zeiss, Germany) at 15 s intervals for 3:30 min The red arrowhead indicates a Cxcl12a spot bound to the tip of the retracting filopodium and the yellow arrowhead points at Cxcl12a, which is bound to the filopodium more proximally and is then engulfed by the cell. Wire presentation visualizes Cxcl12a inside the cell. Scale bar is 5 µm.
Some filopodia adhere to somatic cells and appear to be under tension.
Laser-induced cut of a filopodium was performed using a Zeiss LSM710 confocal microscope equipped with a Zeiss W Plan-Apochromat 63× objective controlled by the ZEN software (Zeiss, Germany). Following cut, the tip of the filopodium remained in contact with the distant somatic cell, while the part of the protrusion attached to the cell rapidly retracted, signifying tension. The video spans 7 s with snapshots captured at a 200 ms time-interval (pinhole 208 µm, 128 × 128). Time indicated in seconds and scale bar represents 5 µm.
Additional files
-
Supplementary file 1
Experimental design, additional materials and protocols.
- https://doi.org/10.7554/eLife.05279.039