Angular velocity integration in a fly heading circuit
Figures
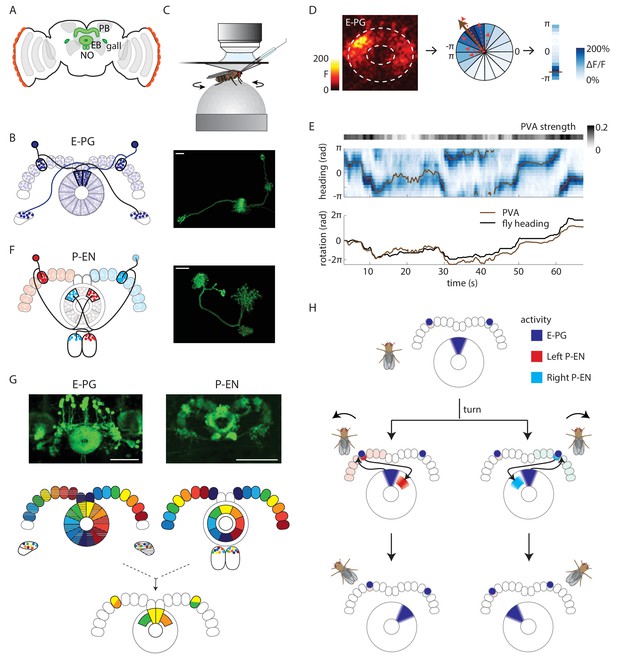
Anatomy suggests a potential circuit mechanism to update a compass representation.
(A) Schematic of the fly brain. Highlighted in green are the ellipsoid body (EB), protocerebral bridge (PB), and the paired gall and noduli (NO). (B) (Left) Schematic of the morphology of two E-PG neurons innervating different sides of the protocerebral bridge. The probable direction of information flow is from their predominantly spiny arbors in the ellipsoid body (‘E-’) to their predominantly bouton-like projections in the protocerebral bridge and gall (‘-PG’). (Right) A single GFP-labeled E-PG neuron. Scale bar: 10 µm. (C) Schematic of the head-fixed walking fly preparation used for two-photon calcium imaging and single cell electrophysiology. (D) For a given two-photon imaged volume (Left, maximum intensity projection of the ellipsoid body), the population vector average (PVA, brown arrow, Center) for a given time point is computed by summing vectors representing the instantaneous calcium activity of each sector of the ellipsoid body (for example, the dotted red arrows shown here for a few sectors). Each sector is a 22.5o slice of the ellipsoid body, defined manually, and each sector’s vector points radially outward along its half angle. PVA strength is the normalized amplitude of the summed vector. (E) (Top) E-PG calcium activity (blue) in the ellipsoid body as the fly turns in darkness. The 16 ellipsoid body sectors are shown unwrapped from –π to π. The PVA is shown in brown, PVA strength is at the top. (Bottom) Comparison of the fly’s heading (black) with the PVA shows a tight correlation of the two, albeit with some drift. This example shows a trial in which the PVA closely matches the heading. We also observed larger, low frequency shifts between the two, as reported previously (Seelig and Jayaraman, 2015). (F) (Left) Schematic of the morphology of two P-EN neurons innervating different sides of the protocerebral bridge. P-EN neurons arborizing in the same protocerebral bridge glomeruli as their E-PG counterparts send processes to offset (neighboring) sectors of the ellipsoid body. Processes in the protocerebral bridge are overwhelmingly spiny and likely dendritic (Wolff et al., 2015). Processes in the ellipsoid body and noduli are predominantly bouton-like and suggestive of presynaptic specializations. (Right) A single GFP labeled P-EN neuron. Scale bar: 10 µm. (G) (Top left) GFP-labeled E-PG neurons in the R60D05 Gal4 line (maximum intensity projection, reproduced with permission from Janelia FlyLight Image Database (Jenett et al., 2012). (Top right) GFP-labeled P-EN neurons in the R37F06 Gal4 line (maximum intensity projection reproduced from Janelia FlyLight Image Database [Jenett et al., 2012]). (Middle left) Ellipsoid body to protocerebral bridge connectivity map for E-PG neurons. Single neurons that arborize in one wedge of the ellipsoid body arborize in the glomerulus of the same color and shading in the bridge. Arborizations in the gall do not exhibit a stereotyped pattern. (Middle right). Protocerebral bridge to ellipsoid body connectivity map for P-EN neurons. Single neurons that arborize in one glomerulus in the bridge arborize in the ellipsoid body tile with the corresponding color. Arborizations in the noduli do not exhibit stereotyped patterns. (Bottom) Single E-PG and P-EN neurons that arborize in the same protocerebral bridge glomerulus have non-overlapping processes in the ellipsoid body with a stereotyped angular shift between the two. All scale bars are 50 µm. (H) Overview of an anatomically motivated mechanism to update a heading representation. E-PG neurons are assumed to make excitatory connections onto P-EN neurons in the protocerebral bridge. P-EN neurons, in turn, are assumed to make excitatory connections onto E-PG neurons in the ellipsoid body. A bump of activity in E-PG neurons (dark blue) represents the fly’s heading in the ellipsoid body. This bump of activity would result in two bumps of E-PG activity in the protocerebral bridge, one on either side. If the two sides of the bridge were to receive asymmetric input dependent on the fly’s angular velocity and turning direction, P-EN neurons with dendrites in the bridge columns that also receive E-PG input would be activated. The anatomical shift between the P-EN and E-PG neurons in the ellipsoid body (compare B and F) would then cause the P-EN neurons to excite E-PG neurons nearby, shifting the E-PG activity bump and updating the heading representation. Note also that the mirror-symmetric activation of the two sides of the bridge would also be visible in activity differences in the noduli (see F and Figure 2A), each of which only receives P-EN projections from the opposite side of the brain.
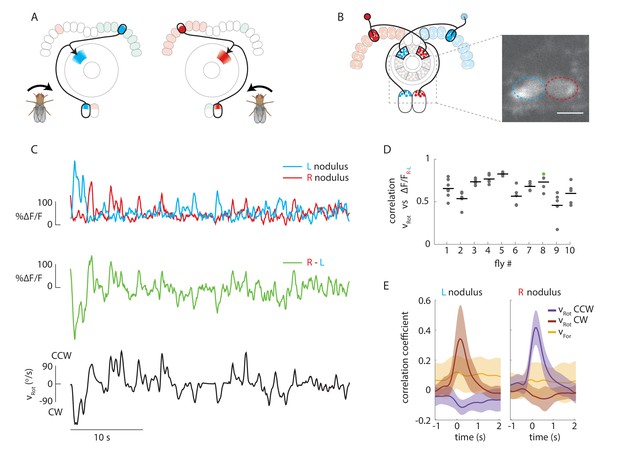
Calcium activity of P-EN neurons in the paired noduli correlates with fly’s rotational velocity in darkness.
(A) Schematic showing how a conceptual model (Figure 1H) to update heading representation would influence calcium activity in the paired noduli. Asymmetric activation of one or the other side of the bridge when the fly turns would, because of P-EN neurons’ projection to the contralateral nodulus, result in activation of the opposite nodulus. (B) (Left) Schematic of the P-EN neurons, highlighting the left and right P-EN populations in red and light blue, respectively. Dashed rectangles mark the imaged region shown at right. (Right) Average of sample two-photon calcium imaging stack showing P-EN GCaMP6f signal in the left and right nodulus. ROIs used to calculate ΔF/F values are outlined by dotted ovals. For this and all other imaging experiments, F is defined as the lowest 10% of fluorescence levels during the trial. The scale bar is 20 μm. (C) P-EN calcium transients in the right (red) and left (blue) nodulus, and the difference between activity levels in the two noduli (green), compared to the fly’s rotational velocity when walking in darkness (black). The velocity trace is convolved with the GCaMP6f time constant (see Materials and methods). (D) Correlation between the convolved rotational velocity and the difference between right and left noduli calcium activity across flies and across trials. Bars show mean values (N = 10 flies). The green point marks the example shown in C. (E) Linear regression analysis for calcium imaging in the noduli shows coefficients for the correlation of P-EN calcium activity in each nodulus with forward as well as clockwise and counter-clockwise rotational velocity.
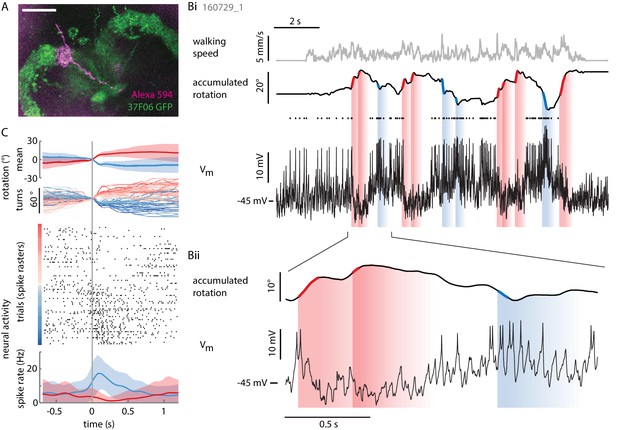
Individual P-ENs are tuned to the fly’s rotations.
(A) Z-projection of confocal image of a single P-EN neuron filled with Alexa594 (magenta) during intracellular whole-cell patch clamp recording in R37F06-GAL4 flies expressing GFP (green). Scale bar: 20 µm. (B) Example recording of a P-EN with soma location and protocerebral bridge innervation in the right hemisphere. The fly was walking in darkness. (Bi) Plotted are the fly’s translational velocity (top row, gray) and accumulated rotation (second row, black), along with the cell’s membrane potential (third row, black). Spike times are indicated with dots. Epochs of fast rotations are highlighted in red and blue on the rotation trace (left and right rotations, respectively). Since the change in P-EN activity often outlasted the duration of a turn, the vertical blue and red shaded regions indicate a time window of ~500 ms in which the neuron’s activity reflected these prior fast rotations. (Bii) Expanded view, highlighting the P-EN neuron’s hyperpolarization in response to rotations in the contralateral direction (for this neuron, left, red) and depolarization with spiking in response to rotations in the ipsilateral direction (for this neuron, right, blue). (C) All of the epochs during which the fly’s angular velocity exceeded 80°/s were temporally aligned and color-coded by turn amplitude (right: red, left: blue). The top panel shows the mean rotation in each direction, the second panel the individual turns. Note that the time point ‘0’ denotes when the angular velocity exceeds 80°/s, which is not the time of turn onset, since rotational velocities vary smoothly and continuously. The neural activity is plotted as a raster plot for individual turns (third panel) and as averaged spike rates (bottom panel).
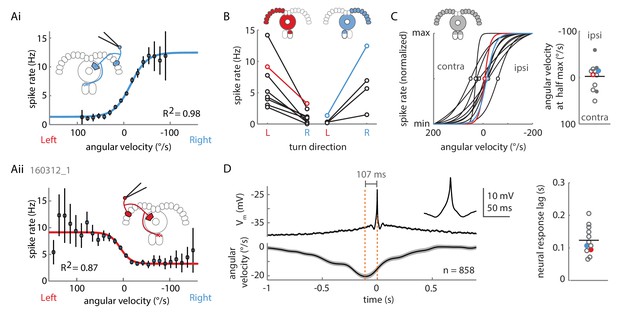
Two P-EN subpopulations mirror-symmetrically encode the fly’s rotational velocity.
(A) Example tuning curves of P-EN spike rate to the fly’s rotations as the fly walks in darkness. Angular velocities were binned in 12°/s bins and a sigmoid was fitted with bin counts used as weights (see Materials and methods for details). Mean spike rate and 95% confidence interval in black, sigmoidal fits in blue and red. (Ai) Tuning curve for a right P-EN neuron (same as in Figure 3). P-EN membrane potential changes were similar to the observed changes in spike rates (see Figure 4—figure supplement 1C for Vm tuning curve). (Aii) Tuning curve for a left P-EN neuron (see Figure 4—figure supplement 2A for example trace). (B) Fitted spike rates for the flies’ turns to the left versus right illustrate mirror-symmetric tuning properties of the left and right P-EN subpopulations. Spike rates were computed either at saturation or at an absolute rotational velocity of 200°/s, whichever was lower. Example cells from panel A are color coded. (C) Encoding of rotations by the two subpopulations is mirror-symmetric yet overlapping, that is, each P-EN subpopulation encodes rotations in both directions. Left: Normalized tuning curve fits for all P-EN neurons. Left hemisphere P-EN curves have been reflected for simplicity. Neurons plotted in A are color coded for comparison. Open circles mark each sigmoid’s half-maximum (inflexion point). Right: On average, the P-EN neurons’ receptive field for rotations is centered around 0°/s, where P-EN’s are half-maximally activated. Closed and open circles in the right subplot represent inflexion points of right and left P-EN tuning curves, respectively. Example cells from panel A are in blue and red. (D) Spike-triggered averages of angular velocities were constructed to characterize P-EN timing. Since P-EN neurons tend to spike at rest, all spikes with rotational velocities not exceeding 40°/s at any time in a one second window around the spike were excluded. Left: Membrane potential is plotted at top, angular velocity at bottom. Inset shows a magnification of the average spike shape. Right: The peak of the angular velocity precedes the spike in all P-ENs recorded. Example cells from panel A are in blue and red.
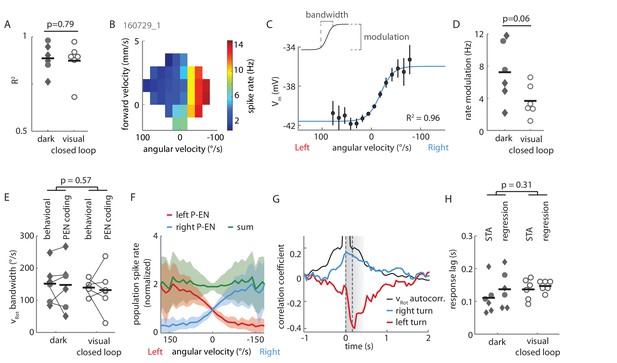
Extended data on P-EN rotational velocity tuning.
(A) Tuning curves for recordings in both experimental conditions (‘dark’ and ‘visual closed loop’) were well fit by a sigmoidal function. P value refers to unpaired t-test across conditions. Cells in the lower spectrum of R2 values (<0.8) showed a higher variance around the mean, but no systematic deviation from the sigmoidal shape of the tuning curve. (B) Heat map of spike rate as a function of forward and angular velocity for the neuron plotted in Figure 3B. (C) Angular velocity tuning curve of the P-EN neuron plotted in Figure 4Ai, but relating membrane potential, rather than spike rate, to angular velocity. Mean membrane potential and 95% confidence intervals in black, sigmoidal fit in blue. (D) Each P-EN’s rate modulation was quantified as the absolute difference of fitted spike rates at saturation or at an absolute rotational velocity of 200°/s, whichever was lower (see inset in panel C). P-EN modulation appeared stronger in flies walking in darkness than in those with visual closed loop. P value refers to unpaired t-test across conditions. (E) Comparison of the fly’s dynamic range of rotations (‘behavioral bandwidth’) to the P-EN’s dynamic range for angular velocity encoding (‘P-EN coding bandwidth’, see inset in Panel C) for the two experimental conditions. Behavioral bandwidth was defined, for each fly, as the range between the 10th and 90th percentile of its rotational velocity distribution (in 50 ms bins, excluding velocities between −5 and 5°/s). P-value refers to a two-way ANOVA result across conditions (p-value for comparison of behavior to neural response: p=0.82). (F) Simulation of P-EN population activity based on electrophysiological recordings of 12 P-EN neurons. Spike rates of individual P-EN neurons were normalized to their respective mean spike rate across all bins. P-EN neurons from the left hemisphere were flipped so that population activity of ‘right P-ENs’ (blue) represents the mean of all cells. The tuning profile of ‘left P-ENs’ (red) was assumed to be the same, except mirror-symmetric around the y-axis (Figure 4C). The population activity (the sum of left and right P-EN neurons, green) was calculated as the linear sum of all 24 simulated P-EN neurons. (G) Generalized linear regression analysis of P-EN instantaneous spike rate relative to the fly’s turns, shown for the experiment plotted in Figure 3. The autocorrelation of the rotational velocity is plotted in black, the correlation coefficients for right and left turns with the neuron’s spike rate are plotted in blue and red, respectively. In brief, P-EN spike rates were regressed with rotations to the left and right as predictors, yielding a single pair of coefficients. A series of temporal shifts was introduced between rotations and spike train, giving rise to the temporal sequence plotted along the x-axis. The temporal shift that produced the maximum difference in left minus right turn coefficients was defined as the neurons response lag. This lag was positive for all P-EN neurons (that is, the neural response followed the change in rotational velocity). See Materials and methods for details. (H) P-EN response lag grouped by recording conditions. ‘STA’ refers to timing information extracted from spike triggered averages (see Figure 4D). ‘Regression’ refers to timing information obtained through fitting a generalized linear regression to the P-EN spike rate (see panel F and Materials and methods for details). P-value refers to a two-way ANOVA result across conditions (p-value for comparison of STA to regression: p=0.57). Timing information extracted from linear regression analysis of the membrane potential was similar to that extracted from generalized linear regression of spike rates (response lag Vm: 125 ± 21 ms, spike rates: 138 ± 83 ms, N = 8 for both groups, paired t-test: p=0.69).
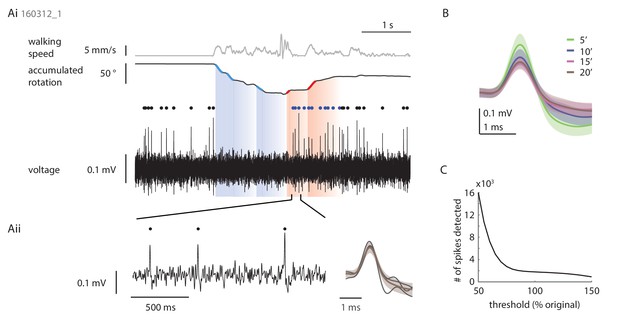
Loose patch recordings.
(A) Example loose patch recording of a P-EN neuron located in the left hemisphere. (Ai) Plotted are the translational velocity (top row, gray), the accumulated rotation (second row, black) and the band-pass filtered extracellularly recorded potential changes (third row, black; see Materials and methods). Epochs of fast turning are highlighted in red and blue for left and right turns, respectively. Spike times are indicated with dots following the same color code. (Aii) Expanded view highlighting the spike shape of three events (left) in comparison to the average of all spikes recorded in this trial (right). Y-axis scale bar is the same for both panels. (B) Average spike shape across all events recorded in four consecutive epochs of five minute length. The traces in panel A are taken from the last epoch and illustrate the minimum quality criterion for inclusion of a recording in the final data set. In most recordings, spike amplitude decreased over time, and spike shape, particularly the post-spike hyperpolarization component, became less distinctive. Usually, but not always, this was accompanied by a slow increase in spike frequency. Experiments were terminated (and/or late trials excluded post-hoc) if the spike frequency increased notably, be it due to decreased signal-to-noise ratio and increased uncertainty in spike detection or, in rare cases, a partial break-in into the cell. (C) Threshold-based spike detection for trials included in the final dataset is robust, i.e. the number of detected spikes is largely constant in a ± 20% window around the chosen threshold.
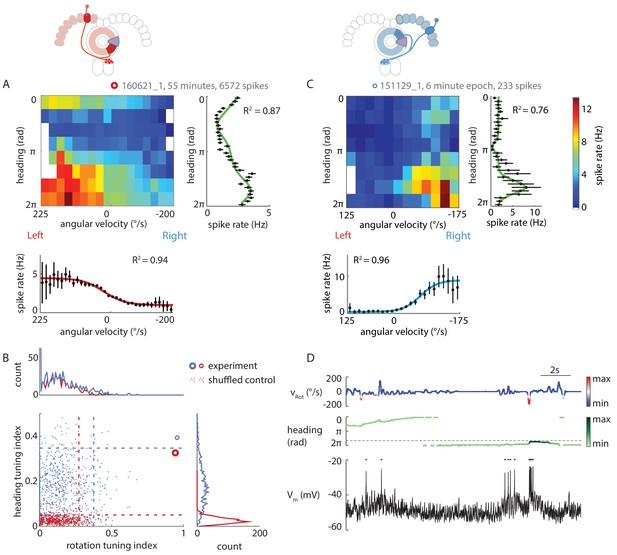
P-ENs are conjunctively tuned to angular velocity and heading.
(A) Two-dimensional tuning properties of a P-EN’s spike output averaged over the entire duration of the experiment. The cell was located in the left hemisphere (schematic at top). The fly walked with closed loop visual feedback. The heat map (upper left) shows spike rates as a function of angular velocity and virtual heading. A tuning curve was fitted to the fly’s virtual heading angle (upper right) as well as the fly’s angular velocity (lower left) (see Materials and methods). Black: mean rate and 95% confidence intervals. Tuning curves are least squares fit to the sum of two von Mises functions for heading (green) and to a sigmoidal function for rotations (red). (B) Quantification of the heading tuning index (mean vector length of spike rates across virtual heading angles) and the rotation tuning index (R2 values for sigmoidal tuning curve fit) for the two example cells plotted in A and C (bold red open circle and blue open circle, respectively). Shuffled controls (see Materials and methods) are plotted as dots and dotted lines mark 95th percentile boundaries (plotted in red and blue for the two example cells). Histograms of shuffled data indices are plotted at top and at right following the same color code. Tuning indices for all cells are shown in Figure 5—figure supplement 1C. (C) Two-dimensional tuning properties of a P-EN’s spike output during a brief epoch that still sufficiently sampled the two-dimensional parameter space. The cell was located in the right hemisphere (schematic at top). The fly walked with a closed loop visual feedback. The heat map (upper left) shows spike rates as a function of angular velocity and virtual heading. Colorbar at right. Tuning curves to the fly’s virtual heading angle and angular velocity are plotted analogous to A. (D) Example traces for the epoch plotted in C illustrating tuning to rotational velocity as well as virtual heading. The two parameters are color coded according to the neural activity expected from the tuning curves plotted in panel C.
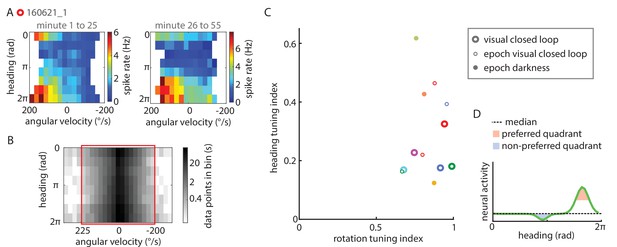
Extended data on P-EN conjunctive tuning.
(A) Tuning properties are consistent over time. Heat map showing spike rate as a function of angular velocity and virtual heading angle for the neuron shown in Figure 5A, plotted separately for the first (left) and second half of the experiment (right). (B) Grayscale map of sample sizes in individual angular velocity ∪ heading angle bins, expressed in seconds of experimental data, plotted with a logarithmic scale color code. To minimize biases in the sampling of particular angular velocity ∪ heading angle combinations, only data within a well-sampled rectangle were included in the analysis of conjunctive tuning properties (indicated by the red box). For the cell plotted here (the same one as plotted above and in Figure 5A), angular velocities <-200°/s and >225°/s were excluded from the analysis. (C) Quantification of the heading tuning index (mean vector length of spike rates across virtual heading angles) and the rotation tuning index (R2 values for sigmoidal tuning curve fit) for all data used to analyze P-EN conjunctive tuning properties. Bold open circles represent closed loop experiment averages, thin open circles in matching colors mark short epochs analyzed from those experiments. Filled circles represent short epochs analyzed from flies walking in darkness. Shuffled controls are omitted for clarity. Color code for individual experiments is matched to Figure 6. (D) Schematic illustrating how preferred and non-preferred heading quadrants were derived from heading tuning curves.
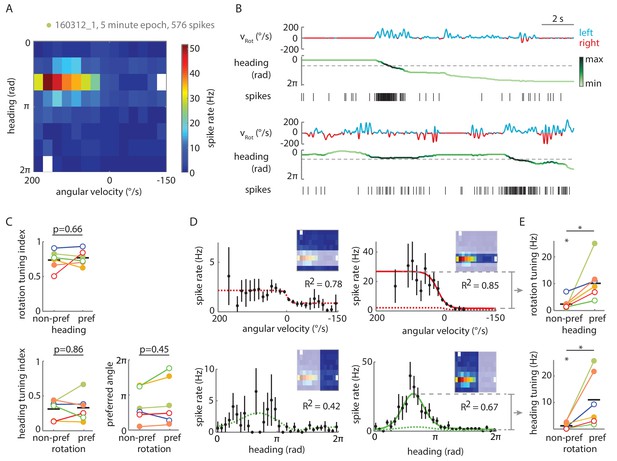
Tuning of P-EN spiking activity is evident even for non-preferred heading and rotational velocities.
(A) Heat map shows conjunctive tuning of a P-EN’s spike output to angular velocity and virtual heading. The fly walked in darkness. (The recording is the same as shown in Figure 4Aii and Figure 4—figure supplement 2). (B) Example traces from that recording illustrating conjunctive tuning to rotational velocity and virtual heading. The fly’s rotational velocity is color-coded by preferred (left, blue) and non-preferred (right, red) turn direction. The fly’s heading is color coded according to the neural activity expected from the heading tuning curve computed from A (see Materials and methods, light green: low, black: high). (C) P-EN spike output is tuned to rotations irrespective of the fly’s heading (top, R2 value of sigmoidal tuning curve fit) and informative about the fly’s heading irrespective of rotations, as evident both from the heading tuning index (bottom left, mean vector length of spike rates across virtual heading angles) and the relative stability of the preferred heading angle (mean vector angle). P-values are results of paired two-sample t-tests. (D) Conditional tuning curves for the cell plotted in A. Schematics illustrate the subset of data used to construct the tuning curves. Mean and 95% confidence intervals are plotted in black, fits for preferred conditions in solid and for non-preferred conditions in dotted lines. Top: Tuning to angular velocity given heading (left: rotations in non-preferred heading quadrant, right: rotations in preferred heading quadrant). Bottom: Tuning to heading given turn direction (left: non-preferred turn direction, right: preferred turn direction). (E) Strong P-EN conjunctive tuning is apparent from the increased rate modulation for tuning to one parameter in the preferred range of the other. Top: Difference in fitted spike rates at −150 and 150°/s for each condition (two sample t-test: p=0.049, N = 6). Bottom: Difference in fitted spike rates at the heading angles corresponding to the peak and the trough of the unconditional heading tuning curve (two sample t-test: p=0.042, N = 6). Tuning to rotations in non-preferred heading quadrants (top left) and tuning to heading during non-preferred rotations (bottom left) is, however, significantly different from zero (one sample t-test: p=0.026 and p=0.043, respectively). Filled circles are recordings in darkness; open circles, in visual closed loop. Color code is the same as used in Figure 5—figure supplement 1C. p<0.05.
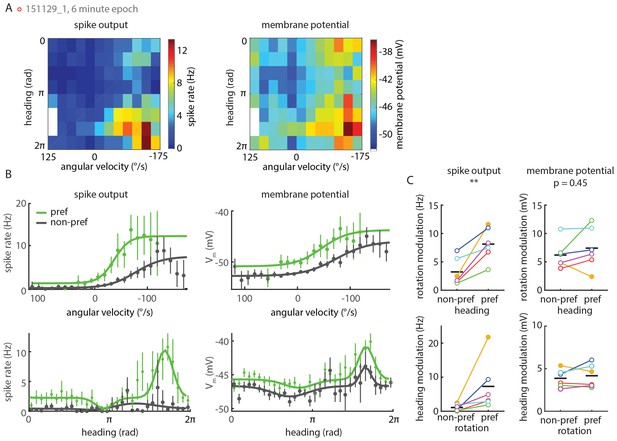
P-EN spiking activity shows stronger conjunctive tuning than membrane potential.
(A) Heat maps show tuning of a P-EN neuron’s spike rate (left) and membrane potential (right) to angular velocity and virtual heading. Left panel replotted from Figure 5C. (B) Conditional tuning curves for the recording plotted in A. Top: Tuning to angular velocity given heading. Bottom: Tuning to heading given turn direction. Tuning to one parameter in the second parameter’s preferred and non-preferred range is plotted in green and gray, respectively. Tuning curves within one subpanel show more pronounced differences on the level of spike rates (left) than subthreshold changes in membrane potential (right). Individual data points show mean and 95% confidence intervals. (C) Rate modulation (maximum minus minimum spike rate along the fitted curve) for all whole-cell recordings (N = 6) shows strong conjunctive tuning at the level of spike rates (left). Conjunctive tuning is much less pronounced at the level of the cell’s membrane potential (right). In a two-way ANOVA, quantitative modulation of membrane potential tuning was indistinguishable for preferred versus non-preferred conditions (p=0.454) whereas quantitative modulation of spike rates was significantly different (p=0.009). Closed circles represent recordings in darkness, open circles recordings in visual closed loop. The color code for individual neurons is the same as in Figure 5—figure supplement 1D.
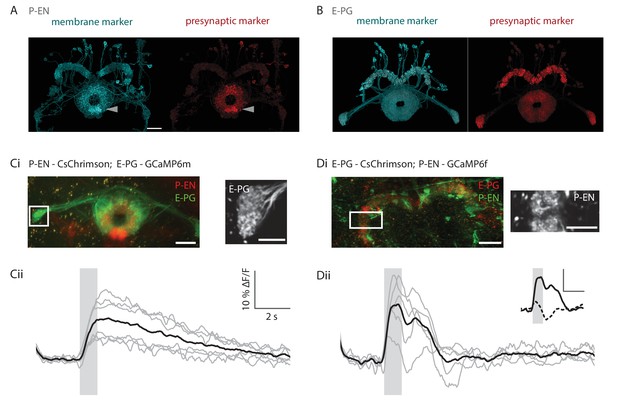
Functional connectivity of E-PG and P-EN neurons.
(A) P-EN neuron membranes and presynaptic sites are labeled by expressing myr-sm::GFP and synaptotagmin in VT008135-GAL4, a second genetic line that drives expression in the P-EN neurons. While additional central complex and central brain cell types are targeted in this line, only the P-EN neuron arborizes in the protocerebral bridge (see Materials and methods). Expression is evident in the protocerebral bridge, the ellipsoid body and the paired noduli (behind the ellipsoid body, labelled by arrowhead). Intensity of presynaptic labeling was strongest in the ellipsoid body. The scale bar is 20 μm and applies to A and B. Though the image stack was rotated in three dimensions to obtain the included view, the scale bar was obtained from a single plane of the imaging stack. (B) Analogous to A, but using R60D05-GAL4 to drive expression in the E-PG neurons. Although presynaptic labeling was most intense in the protocerebral bridge and gall, synaptotagmin was also detected in the ellipsoid body, suggesting E-PG presynaptic sites in that neuropil as well. (C) Functional connectivity experiment with CsChrimson-mCherry expressed in P-EN, and GCaMP6m in E-PG neurons. (Ci) (Left) Average projection of fluorescence from a dissected brain. Both E-PG and P-EN arborizations can be seen in the ellipsoid body. E-PGs furthermore project to the gall (box, left) and P-ENs to the noduli (paired structure at bottom). (Right) Average frame from imaging the E-PGs in the gall while activating the P-ENs. (Cii) Average response (in black) from six flies to a train of 2 ms, 590 nm light pulses delivered at 30 Hz and 50 μW/mm2. Stimulation time indicated by gray area. Gray traces correspond to the average of 4 trials for individual flies. (D) Functional connectivity experiment with CsChrimson-mCherry expressed in E-PG and GCaMP6f in P-EN neurons. (Di) (Left) Average projection of fluorescence from a dissected brain. (Right) Average frame from imaging the P-ENs in the protocerebral bridge while activating the E-PGs. (Dii) Analogous to Cii, except that stimulation light intensity was increased to 500 μW/mm2. (inset) Comparison of low stimulation intensity responses (50 μW/mm2, dotted line) and the high stimulation responses shown in the plot below (solid line). These responses were acquired in different regions (low stimulation: noduli, high stimulation: protocerebral bridge), but results were consistent across neuropiles. The scale bars are scaled versions of those shown in Cii. All scale bars in Ci, Di: 10 µm.
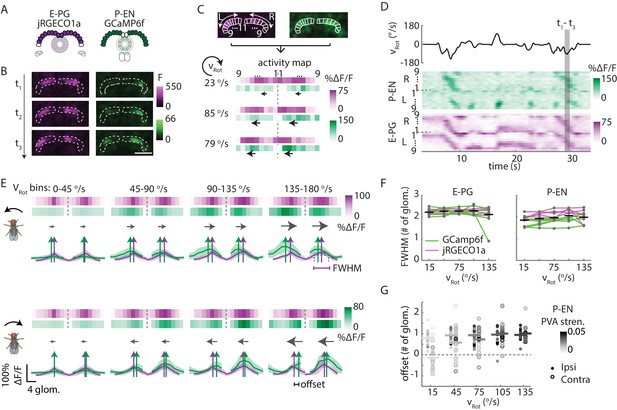
In the protocerebral bridge, P-EN calcium activity overlaps E-PG calcium activity with an offset.
(A) Overview of a typical protocerebral bridge imaging experiment. jRGECO1a (shown in magenta), a red calcium indicator, is expressed in the E-PG neurons. GCaMP6f (shown in green), a green calcium indicator, is expressed in the P-EN neurons. Both populations are imaged simultaneously. (B) Maximum intensity projections of fluorescence from simultaneous imaging of E-PG and P-EN neural populations. Time points are 0.4 s apart. Scale bar: 50 μm. (C) ROI definition and ΔF/F projections for E-PG neurons (magenta) and P-EN neurons (green). The three time points shown in B are displayed at the bottom. The dotted line divides the right and the left half of the protocerebral bridge. Arrows indicate the direction of bump movement. (D) Protocerebral bridge activity over time for one trial for the E-PG and P-EN neurons. The fly’s rotational velocity during the trial is shown in the black trace at top. The right and left side activity is concatenated. (E) Fluorescence transients binned by angular velocity and aligned to the peak of E-PG (magenta) activity in the right protocerebral bridge for five trials for one fly. The bar projections show the mean values while the cross-sectional plots show the mean and standard deviation. Colored vertical arrows show the PVA values of the traces. (F) Bump half widths (full width at half maximum, or FWHM) for P-EN neurons and E-PG neurons across flies and across velocity bins. The center velocity of each bin is shown, and the bin width is 30°/s. Points for individual flies are connected with colored lines indicating the calcium indicator tag. Normalized P-EN (E–PG) widths, obtained by dividing each width by the bump width for the given fly at 0–30°/s, were flat across velocities, with slopes of −0.71 ± 2.2×10−3 (0.98 ± 0.68×10−3) s/° for the green indicator and 0.36 ± 0.68×10−3 (0.55 ± 1.1×10−3) s/° for the red indicator. (G) Difference in the population vector average (PVA) for the mean E-PG and P-EN calcium activity in the side of the protocerebral bridge ipsilateral and contralateral to a turn, plotted across flies and across angular velocity bins (N = 5 flies with the P-EN neurons tagged with GCaMP6f and E-PG neurons tagged with jRGECO1a, and N = 6 flies with the opposite calcium indicator combination). Each point represents the mean across trials for one fly and bars show the mean across flies. Individual dots are shaded to match P-EN PVA strength. At 120–150°/s, the offset is 0.96 ± 0.40 glomeruli for the ipsilateral side and 1.0 ± 0.56 glomeruli for the contralateral side. If the offset is instead calculated by finding the average PVA offsets of all of the individual traces (instead of the one PVA offset of the average trace), those values become 0.97 ± 0.43 and 0.46 ± 1.0 for the ipsilateral and contralateral sides, respectively. If the peaks are used instead of the PVA, the offsets at 120–150°/s are found to be 0.59 ± 1.2 and 0.36 ± 1.3 for the mean traces and 0.95 ± 0.42 and 0.41 ± 0.91 for the mean of the peak differences of the individual traces.
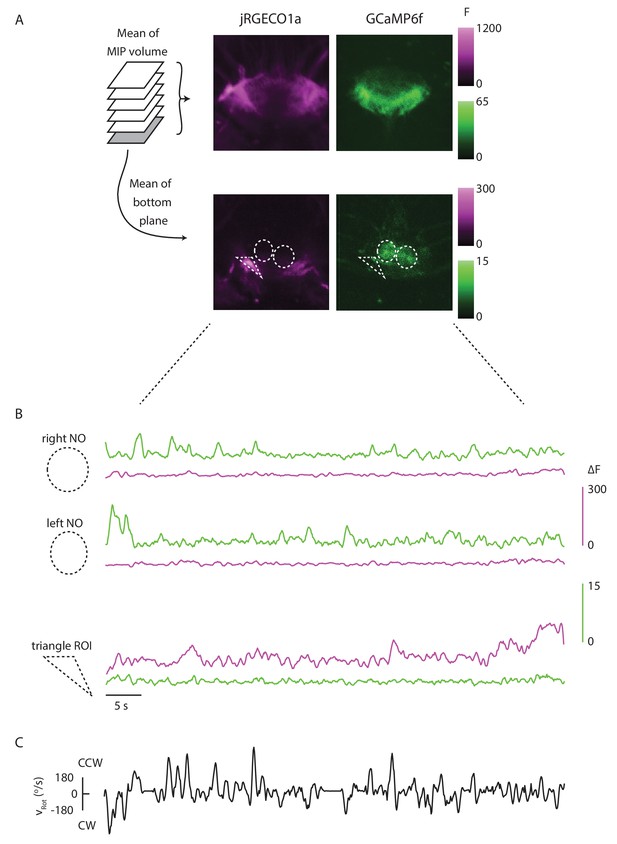
The red and green channels are well separated.
(A) Mean of the maximum intensity projection of an ellipsoid body volume, and mean of the bottom plane for one trial. The P-EN and E-PG populations are noticeably distinct, showing that bleed-through is limited. (B) (Top) If ROIs are drawn around each of the noduli, the mean signal in the green channel within each is strongly modulated by the fly’s turns, without noticeable signals in the red channel. (Bottom) When, instead, a triangular ROI is drawn around an area with a high baseline red fluorescence, but no apparent green signal, the red signal varies noticeably across the trial while the green signal does not. This further shows that the GCaMP6f signal is not bleeding through to the red PMT. (C) The rotational velocity of the fly over the course of the trial shown in A and B.
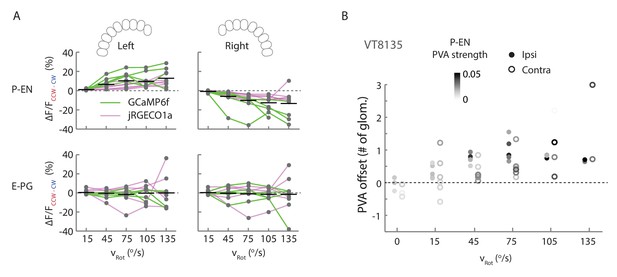
Additional quantification of protocerebral bridge activity.
(A) Amplitude differences between P-EN and E-PG fluorescence transients evoked by counterclockwise and clockwise turns for different angular velocities. Points are connected by colored lines representing the indicator used in a particular fly (magenta: jRGECO1a; green: GCaMP6f). For ipsilateral turns across flies, the normalized P-EN (E–PG) peaks increased at a rate of 2.2 ± 0.96×10−3 (0.69 ± 0.31×10−3) s/o for the green indicator and a rate of 1.2 ± 0.29×10−3 (0.48 ± 0.28×10−3) s/o for the red indicator. (B) PVA offset between the P-EN and E-PG activity as binned by turn velocity, for both ipsilateral and contralateral turns for a second fly line, VT008135, that also labels the P-EN neurons. The dots are shaded by the mean P-EG PVA strength for the given bin.
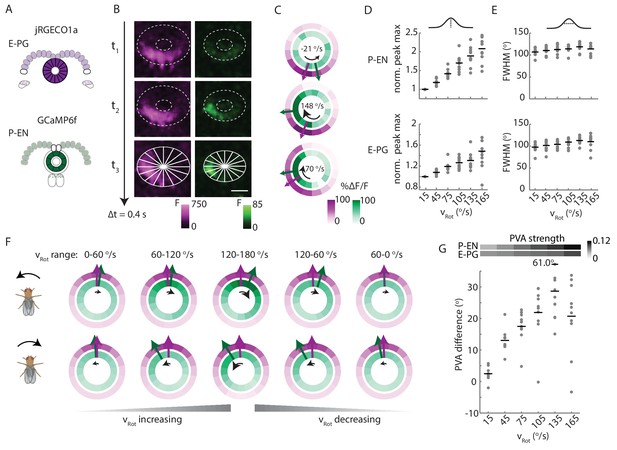
P-EN activity leads E-PG activity in the ellipsoid body.
(A) Overview of a typical two-color calcium imaging experiment targeting the ellipsoid body. jRGECO1a is expressed in E-PG neurons (shown in magenta), and GCaMP6f is expressed in the P-EN neurons (shown in green). (B) Maximum intensity projections of fluorescence from simultaneous imaging of the E-PG and P-EN neural populations. Time points are 0.4 s apart. The bottom images show the regions of interest (solid white lines) and a scale bar of 20 μm. (C) Fluorescence transients and PVA for the three successive time points shown in B, labeled with the corresponding rotational velocities. The direction of bump movement is shown in the inner black arrows. The colored arrows represent the direction but not the magnitude of the PVA. (D) P-EN and E-PG bump amplitude as a function of rotational velocity. The center of each rotational velocity bin is shown. Bin width is 30°/s. Each point represents the mean across trials for one fly and bars show the mean across flies. The values for each fly are normalized to the intensity in the 0–30°/s bin. Statistics for flies with the two populations labeled with the opposite colored indicators are shown in Figure 9—figure supplement 2. Normalized P-EN (E-PG) peaks increased at a rate of 7.4 ± 2.3×10−3 (3.1 ± 1.6×10−3) s/° for the green indicator and a rate of 2.7 ± 1.23×10−3 (3.0 ± 2.2×10−3) s/° for the red indicator. (E) P-EN and E-PG bump half widths (FWHM). Each point represents the mean across trials for one fly and bars show the mean across flies. Statistics for flies with the two populations labeled with the opposite colored indicators are shown in Figure 9—figure supplement 2. Normalized P-EN (E–PG) widths, obtained by dividing each width by the bump width for the given fly at 0–30°/s, were flat across velocity bins, with slopes of −0.20 ± 0.57×10−3 (−0.36 ± 1.3×10−3) s/o for the green indicator and 0.92 ± 0.81×10−3 (0.48 ± 1.1×10−3) s/o for the red indicator. (F) Mean fluorescence transients binned by rotational velocity and aligned to the peak of E-PG activity at 12 o’clock across five trials for the fly shown in A–C. (G) Difference in PVA for mean P-EN and E-PG fluorescence transients across flies and rotational velocity bins. Each point represents the mean across trials for one fly and bars show the mean across flies. (N = 10 flies) The P-EN and E-PG PVA strengths are shown at the top. The offset increase across bins is significant (one-way ANOVA across angular velocities, p=1.3×10−7). The PVA offset at 150–180°/s is 20.7 ± 11.7°. If the PVA offset is instead calculated for each individual pair of traces, the offset is 14.6 ± 20.9° The peak offset of the mean traces is 24.8 ± 22.4° and the mean offset of the peak differences of the individual traces is 25.8 ± 9.8°.
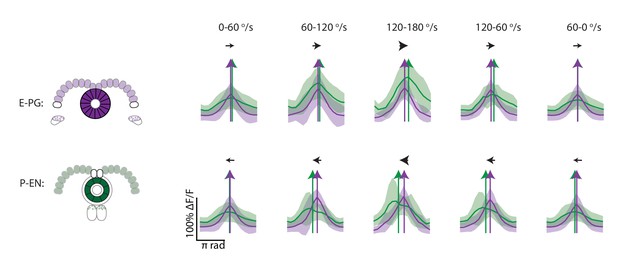
Cross sections of binned and aligned E-PG and P-EN activity.
Cross-sectional plots of binned and aligned ellipsoid body activity. Standard deviations are shown in lighter colors. P-EN activity is in green and E-PG activity is in magenta. Colored arrows show the PVA values in the cross sections.
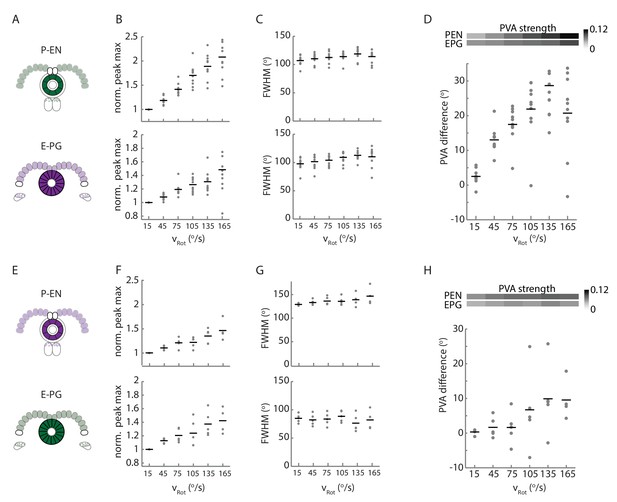
Statistics of P-EN and E-PG activity in the ellipsoid body for flies with the opposite calcium indicator combination.
Comparison of ellipsoid body statistics between N = 10 flies with jRGECO1a expressed in the E-PG neurons and GCaMP6f expressed in the P-EN neurons and N = 5 flies with the flipped indicator combination. Plots A-D are shown and described in Figure 9, and repeated here for easy comparison. (A) Overview of color indicator expression. jRGECO1a is expressed in E-PG neurons (shown in magenta), and GCaMP6f is expressed in the P-EN neurons (shown in green). (B) The intensity increase, normalized for the 0–30°/s condition for the P-EN and E-PG neurons as a function of rotational velocity. (C) The full width at half maximum (FWHM) width of the P-EN and E-PG bumps as a function of rotational velocity bin. (D) The PVA offset between the P-EN and E-PG activity as binned by turn velocity. (E) As in A. jRGECO1a is now expressed in the P-EN neurons while GCaMP6f is expressed in the E-PG neurons. (F) As in B. with the colored indicators now reversed. (G) As in C. with the colored indicators now reversed. (H) As in C. with the colored indicators now reversed. The change in PVA difference, though still positive, is not significant across bins (one-way ANOVA of PVA difference across angular velocities, p=0.26).
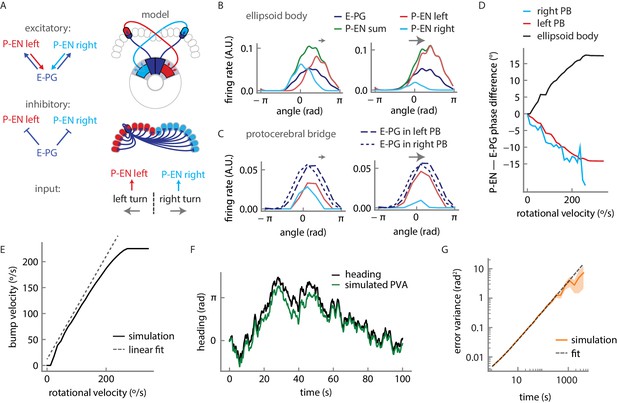
Firing rate model for a circuit mechanism displaying persistent localized activity and angular velocity integration.
(A) Schematic of effective excitatory (top) and inhibitory (middle) connectivity assumed in the firing rate model and external inputs to the P-EN populations (bottom). Note the anatomical shift in the ellipsoid body between E-PG and P-EN neurons relative to their protocerebral bridge connections. We assume one P-EN and three E-PG neurons per protocerebral bridge glomerulus. (B) Activity of E-PG and P-EN neurons in the ellipsoid body for counterclockwise turns at low (left, 35°/s) and high—that is, close to saturation— (right, 190°/s) angular velocities. (C) Activity of E-PG and P-EN neurons in the protocerebral bridge at low (left) and high (right) angular velocities for a snapshot in time. The velocities are the same as in B. (D) PVA difference between P-EN and E-PG bumps in the ellipsoid body (black) or protocerebral bridge (red, blue) for different angular velocities for counterclockwise turns. (E) E-PG bump velocity as a function of the fly’s rotational velocity. The bump velocity displays saturation at high velocities. A linear fit of slope one around the origin is also displayed (upward shifted for display purposes). Rotational velocities along this line will be reliably integrated. (F) Simulated PVA of the E-PG population as a function of time for a time varying rotational velocity input (see Figure 10—figure supplement 2 for a description of the input). (G) Evolution of the estimator of the error variance between the velocity input and the simulated PVA. Beyond 10 s, the statistics of the discrepancy follow a diffusion equation with a diffusion coefficient of 1.82 × 10−3 rad2/s (see Materials and methods for a description of the fitting procedure). The shaded area indicates the standard deviation of the estimator.
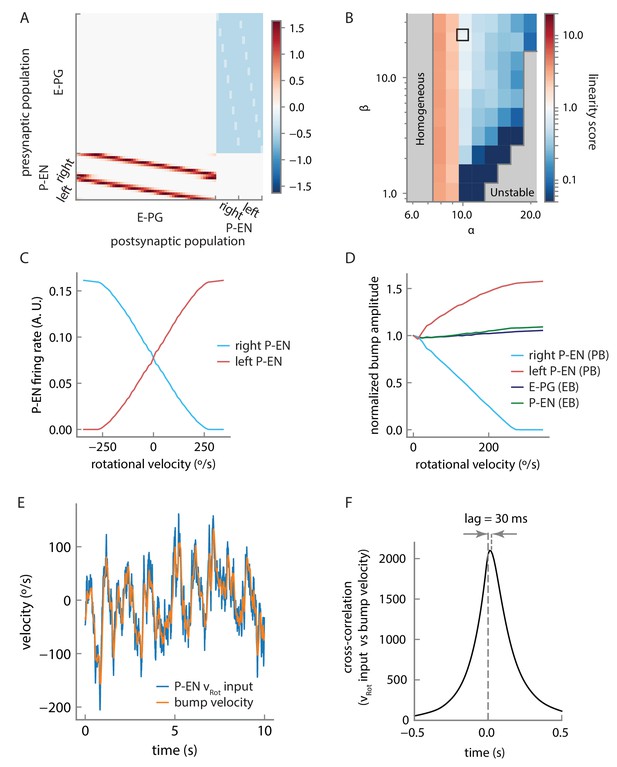
Model connectivity and performance.
(A) Connection matrix for the firing rate model. Note that local E-PG-to-P-EN excitation appears as a reduction in inhibition in the connectivity matrix because of strong uniform inhibition, but is converted to excitation by a constant and positive bias current in the P-EN neurons (see Materials and methods). (B) The linearity of rotational velocity integration in the model depends on the balance of local excitation and inhibition, as represented by model parameters α and β. The parameters we selected lie within the black box, that is, in a near-linear regime of velocity integration for the range of rotational velocities represented by the P-EN neuron tuning curves. Note that perfectly linear velocity integration is represented here by a linearity score of 1 (see Materials and methods). (C) Tuning curves of single, simulated P-EN neurons as a function of the velocity input. (D) Amplitude of the bumps in the ellipsoid body and the protocerebral bridge as a function of rotational velocity. (E) Velocity of the bump compared to artificially generated velocity input to P-EN neurons over a short timescale of 10 s for an artificially generated input velocity (see Figure 10—figure supplement 2 and Materials and methods). High frequencies are smoothed out, but the input is otherwise tracked well. (F) Cross-correlation of the rotational velocity input to model P-EN neurons against the velocity of the activity bump shows that the bump velocity lags the velocity input by ~30 ms. Note that this is the lag of the bump relative to the P-EN velocity input, not to the fly’s rotational velocity.
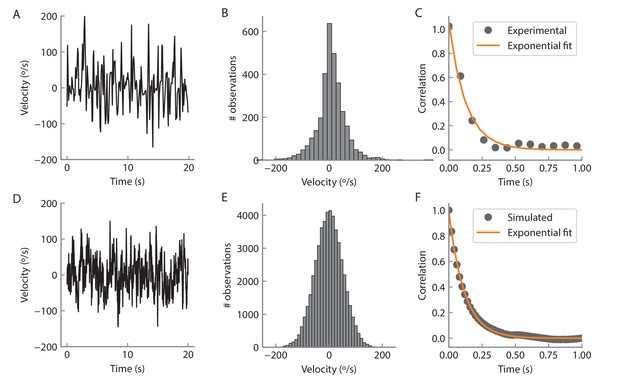
Angular velocity statistics.
(A) Example of an experimentally measured angular velocity track for 20 s. The velocity was downsampled to a rate of 12 Hz to match the volume imaging rate. (B) Histogram of the recorded angular velocities. The velocity was recorded over 350 s and the time points for which the angular velocity was zero were not considered for the analysis. The standard deviation of the distribution is 54°/s. (C) Time autocorrelation of the velocity tracks. The autocorrelation is well fit by an exponential with a time constant of 128 ms (orange curve). D, E, F Same as A, B, C but for a simulated track (see Materials and methods) at a rate of 50 Hz for 1000 s.
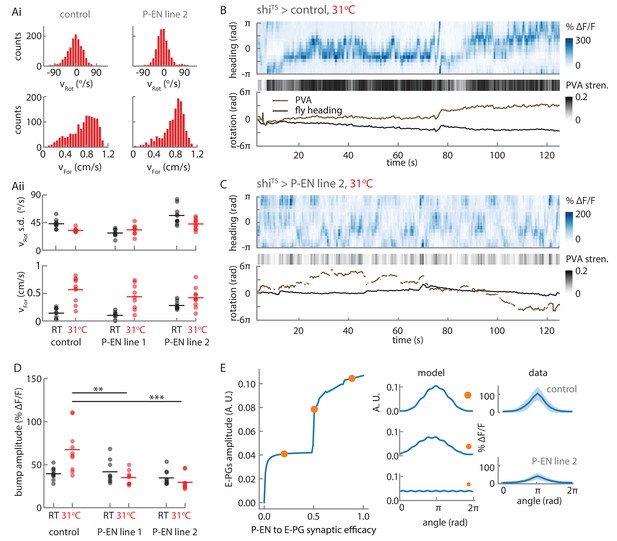
Synaptic block of P-EN neurons disrupts E-PG activity.
(A) Walking statistics for flies across temperatures and across genotypes. (Ai) Example rotational velocity (top) and forward velocity (bottom) distributions for single trials for control (left, shiTS expressed in Empty Gal4) and P-EN blocked (right, shiTS expressed in the P-EN containing line R37F06, herein referred to as P-EN line 2). (Aii) Walking statistics at room temperature (RT, black) and at 31°C (red) for the control line and for two lines that express shiTS in the P-EN neurons. P-EN line one is GAL4-VT008135. The standard deviation of the rotational velocity is plotted at top, while the median of the forward velocity is shown at bottom. Lines show the means of all values. (B) Recordings at 31°C for one fly with shiTS expressed in empty Gal4. (Top) E-PG activity recorded in the ellipsoid body. (Center) The E-PG PVA strength. (Bottom) E-PG PVA and fly heading. The PVA is only shown when the PVA strength exceeds 0.025. (C) Same as in A, but now for a fly with shiTS expressed in the P-EN neurons (P-EN line 2). The PVA strength is lower and the bump movement is sporadic. The PVA is only shown when the PVA strength exceeds 0.025. (D) Mean bump amplitude at RT and at 31°C for all flies and fly lines (N = 10 for the control, N = 9 for P-EN line 1, N = 10 for P-EN line 2, bootstrapping gives p=0.0056 (**) and p=6.0×10−4 (***)). (E) Comparison of the firing rate model predictions and the shiTS data. (Left) E-PG bump amplitude as a function of the P-EN to E-PG synaptic efficacy for the firing rate model. (Center) The predicted ellipsoid body activity for three points along the curve that are marked with orange circles. (Right) The mean and standard deviation of the ellipsoid body activity across trials for the control fly shown in B and the shiTS > P-EN line 2 fly shown in C. The synaptic efficacy for the shiTS fly, likely less than 1, is unknown.
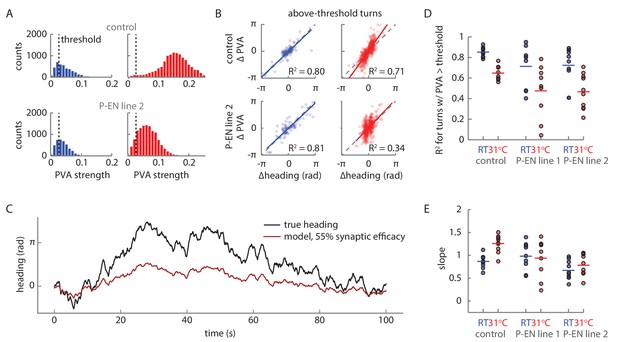
PVA behavior during turns under synaptic block.
(A) PVA strength distribution at room temperature (RT, shown in blue) and at 31°C (shown in red) across trials for the flies shown in Figure 11B and C. The flies walk forward quickly at 31°C, which causes the PVA strength distribution to shift right for the control fly. A PVA strength threshold of 0.025 is shown with the vertical black line. Only periods where the data exceeds this threshold are considered in B. (B) The change in PVA vs. the change in heading for each turn where the PVA strength exceeds 0.025 throughout the turn. A turn is defined as any sustained period where the rotational speed exceeds 15°/s. The solid colored lines show a linear fit to the data. The dotted gray lines show the condition where the change in heading equals the change in PVA. (C) Simulated PVA as a function of time given a time varying rotational velocity input (see Figure 10—figure supplement 2 for a description of the input) and a synaptic efficacy of 55%. (D) R2 values for the linear fits to the change in heading vs. the change in PVA data for thresholded turns, as described in B. (E) The slopes of the linear fits for the change in heading vs. change in PVA data.
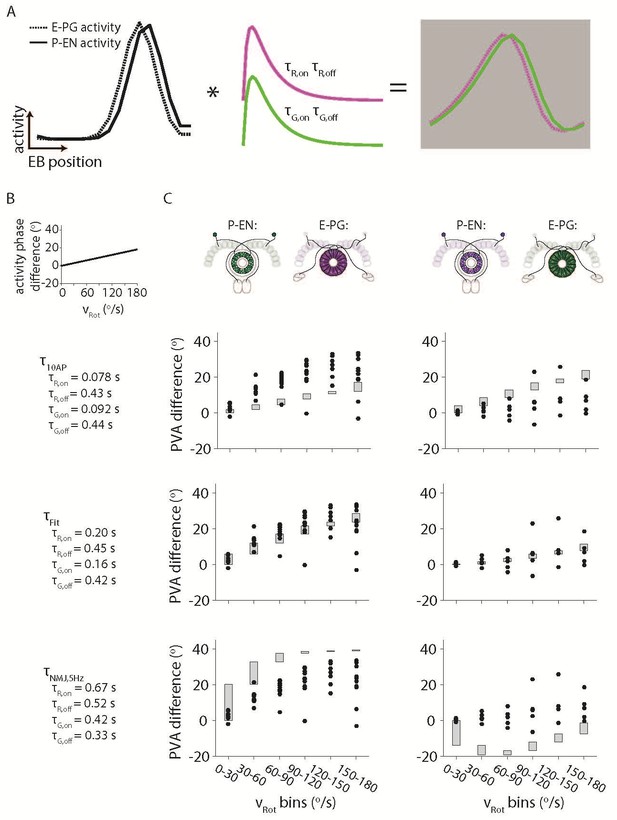
Simulating the effects of the Ca2+ time constants on the observed bump activity.
(A) Overview of the procedure for convolving a simulated activity profile with on and off GECI time constants. The bump profiles are assumed to be Gaussians with full width at half maxima of 90o. Those profiles are then convolved with the difference between a decaying exponential with time constant τon and a decaying exponential with time constant τoff to create a final simulated activity profile. (B) The phase difference between the simulated P-EN Gaussian bump and the simulated E-PG Gaussian bump was assumed to vary linearly with the rotational velocity. (C) The simulated phase difference of the activity bumps after being convolved with a variety of time constants. (Top) The time constants are assumed to be those measured after exciting dissociated mouse neurons with 10 action potentials (Chen et al., 2013) (Dana et al., 2016) (Middle). Time constants chosen to best fit the data. (Bottom) The time constants are assumed to be those measured after exciting neurons in the larval Drosophila neuromuscular junction at 5 Hz (Dana et al., 2016). For the left columns, the P-EN neurons are assumed to express the green GECI, GCaMP6f, while the E-PG neurons are assumed to express the red GEC, jRGECO1a. The opposite colors are assumed to have been used at right.
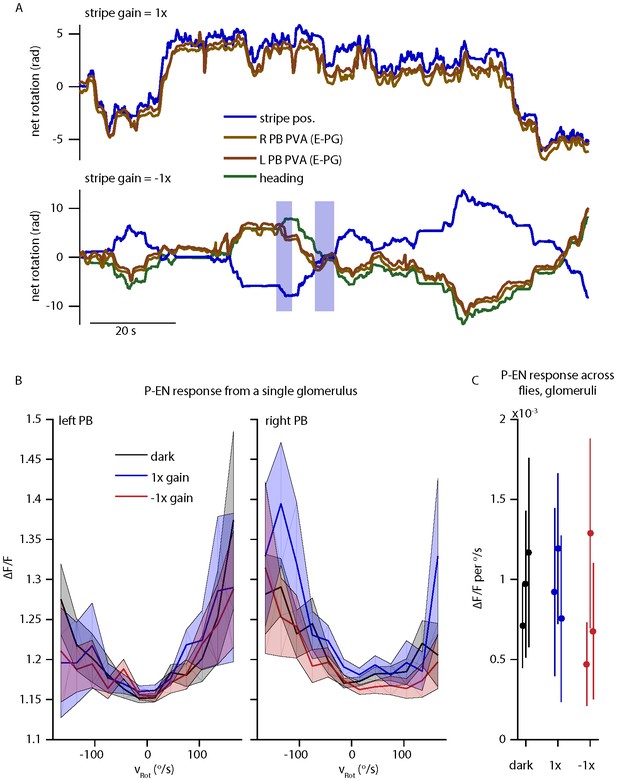
P-EN and E-PG activity in the protocerebral bridge while the fly controls a 15o wide stripe with a gain of -1.
(A) Left and right E-PG bump position, as measured by the bump PVA, for a fly viewing a closed loop strip with a gain of 1 (top) and a gain of -1 (bottom) as compared to the position of the visual stripe and to the fly’s heading. (B) The mean and standard deviation of the left and right P-EN activity in the protocerebral bridge as a function of rotational velocity when the fly is in the dark or viewing a stipe with a gain of 1 or -1 for one fly. (C) Mean (dot) and standard deviation (line) of the mean ipsilatateral P-EN activity (from 0-150 o/s) across flies.
Videos
Cartoon animation of E-PG and P-EN compass function during a turn.
Activity of E-PG neurons is shown in red, and that of P-EN neurons in blue. Animation shows the interaction of the two populations during a turn, and highlights how heading-dependent E-PG input combines with turn-direction-dependent angular velocity input to trigger P-EN activity in the appropriate protocerebral bridge glomeruli. This activity, in turn, activates E-PG neurons in ellipsoid body sectors neighboring those that the bump originally inhabited, thereby updating the heading representation.
P-EN bump leads the E-PG bump in the ellipsoid body.
Example of two-color calcium imaging in the ellipsoid body of a fly walking on a ball. Part 1. (Top) Red channel signal from the E-PG neurons. The outline of the E-PG arborizations in the ellipsoid body is marked with dotted white lines. The heading of the fly, as read out from the ball position, is shown by the white circle. The population vector average of the activity is labeled by the magenta line. (Bottom) Video of the fly on the ball. Part 2. (Top) Green channel signal from the P-EN neurons. The outline of the P-EN arborizations in the ellipsoid body is marked with dotted white lines. The amplitude of the rotational velocity is indicated by the white bar at right. The population vector average is labeled with the green line. (Bottom) Video of the fly on the ball. Part 3. (Top) Combined red and green activity and PVA of both the E-PG and P-EN neurons. (Bottom) Video of the fly on the ball.
Additional files
-
Supplementary file 1
Recording parameters of in vivo electrophysiology.
Listed are, for all in vivo electrophysiology experiments, the fly’s genotype, experimental condition, time spent walking, mean forward, absolute sideslip, and absolute rotational velocity as well as the range of rotational velocities displayed by the fly while walking. Spike rate and membrane potential (Vm) at rest were determined for non-walking periods, maximum spike rates are computed for 50 ms windows. All previous parameters are reported as mean and standard deviation across one-minute windows of the entire experiment. The spike threshold is the membrane potential at the peak of the second derivative of the membrane potential before a spike and was determined for a subset of spikes measured at resting membrane potential. The apparent input resistance was computed from a single exponential fit to the decay phase of a depolarizing current pulse. The bias current is a constant current injected to compensate for the leak through the seal resistance, in some experiments this current was readjusted during the recording.
- https://doi.org/10.7554/eLife.23496.026
-
Supplementary file 2
Imaging genotypes and walking statistics.
Listed are, for all in vivo calcium imaging experiments, the fly’s genotype, temperature (in the case of the shiTS flies), percentage of time spent walking, mean forward, absolute sideslip, and absolute rotational velocity as well as the range of rotational velocities displayed by the fly while walking.
- https://doi.org/10.7554/eLife.23496.027