Striatal fast-spiking interneurons selectively modulate circuit output and are required for habitual behavior
Figures
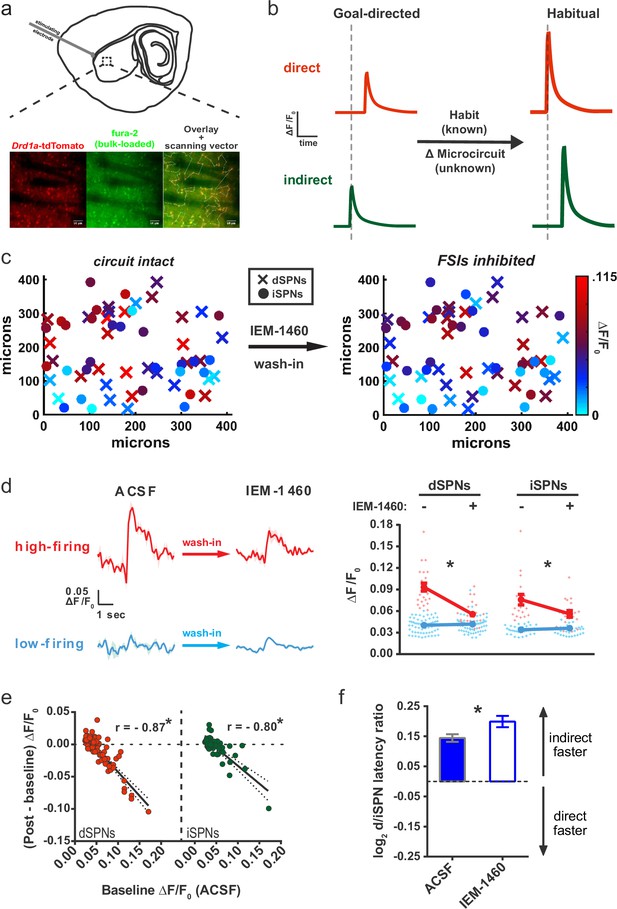
Striatal output reconfiguration following pharmacological inhibition of FSIs directly opposes substrates for habitual behavior.
(A) Schematic of calcium imaging approach. Top: SPN activity was evoked by electrical stimulation of cortical afferent fibers in an acute parasaggital brain slice. Bottom: Evoked SPN firing was imaged in the direct and indirect pathways simultaneously using a transgenic direct pathway reporter mouse line (left), calcium indicator dye fura-2 (middle) and two-photon laser scanning microscopy (right, see scanning vector in overlay). (B) Experimental approach. Striatal microcircuitry was manipulated in tissue from untrained animals in order to reproduce the known circuit substrate for habitual behavior (described in O'Hare et al., 2016) and thereby identify a candidate microcircuit mechanism. (C) Representative heat maps of dSPN (x) and iSPN (●) calcium transient amplitudes before (left) and after (right) pharmacological inhibition of FSIs using IEM-1460 show a selective reduction in cells with the strongest (bright red) initial responses. (D) Left: Representative SPN calcium transient waveforms before and after wash-in of IEM-1460. SPNs were grouped into ‘high-firing’ (red) or ‘low-firing’ (blue) clusters based solely on their baseline response amplitudes using a Gaussian mixture model. SPNs with strong baseline responses (red, ‘high firing’) show weaker responses after wash-in whereas those with initially weak responses (blue, ‘low firing’) are unaffected. Right: Evoked calcium transient amplitudes for all imaged SPNs before (-) and after (+) wash-in of IEM-1460. For both cell types, high-firing SPNs showed decreased responses after IEM-1460 wash-in (dSPNs: t(22) = 6.43, p=0.0000018, n = 23 cells; iSPNs: t(17) = 3.43, p=0.0032, n = 18 cells) whereas low-firing SPNs did not (dSPNs: p=0.24, n = 64 cells; iSPNs: p=0.21, n = 34 cells). (E) Linear regression and correlational analyses show that the inhibitory effect of IEM-1460 on SPN responses (post – baseline difference) is a linear function of baseline response amplitudes for both dSPNs (red; r(86) = −0.87, p=2.20×10−28, n = 87 cells) and iSPNs (green; r(51) = −0.80, p=1.59×10−12, n = 52 cells). (F) Relative pathway timing, as measured by latency to peak detection, before and after inhibition of FSIs using IEM-1460. Indirect pathway activation precedes direct pathway activation by a greater margin after wash-in of IEM-1460 (t(102) = 2.42, p=0.017, n = 52 independent dSPN/iSPN pairs). *p<0.05. Dotted error bands indicate 95% confidence interval. Error bars indicate SEM. Effects of IEM-1460 on FSI and SPN spike probability are shown in Figure 1—figure supplement 1. Electrophysiological assessment of IEM-1460’s effect on evoked multi-AP SPN responses is included in Figure 1—figure supplement 2. GMM parameters and calcium transient amplitude source data can be found in Figure 1—source data 1.
-
Figure 1—source data 1
GMM parameters and source data for SPN calcium transient amplitudes (MATLAB).
GMMs contains parameters for the Gaussian mixture model fits on pre-IEM-1460 calcium transient amplitude data by cell type. Amplitude values are included for high- and low-firing dSPNs and iSPNs in dSPNs_high, dSPNs_low, iSPNs_high, and iSPNs_low. Matrices are N x 2 with column 1 containing pre-drug amplitudes and column 2 containing paired measurements after drug wash-in. Data can be combined within cell type and analyzed using source code file PrePostGMM.m to reproduce the clustering shown in Figure 1D (see comments in code).
- https://doi.org/10.7554/eLife.26231.006
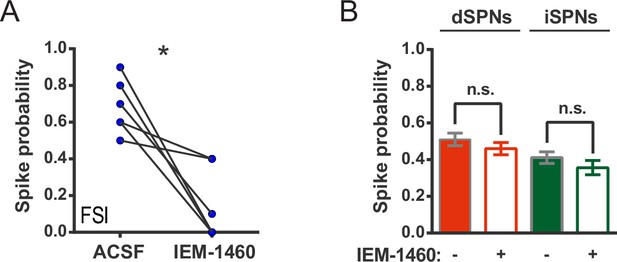
IEM-1460 inhibits evoked FSI firing but does not affect SPN spike probability.
(A) Probability of evoked FSI action potential firing, as measured in cell-attached recordings, before and after wash-in of IEM-1460. Drug wash-in significantly inhibited FSI firing (t(5) = 4.08, p=0.0096, n = 6 cells). (B) Spike probability for dSPNs (red) and iSPNs (green) before (filled) and after (open) wash-in of IEM-1460 in 2PLSM calcium imaging experiments. Drug wash-in did not affect spike probability for dSPNs (p=0.055, n = 87) or iSPNs (p=0.11, n = 52). *p<0.05. Error bars represent SEM.
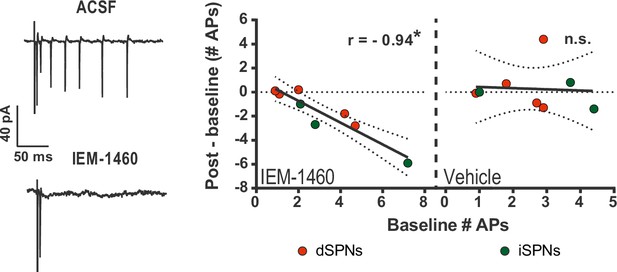
IEM-1460 selectively inhibits evoked multi-action potential SPN responses ex vivo.
Cell-attached electrophysiological recordings showing selective effect of IEM-1460 for multi-action potential SPN responses to afferent stimulation. Left: example trace showing multi-action potential SPN response to single-pulse stimulation of cortical afferents (top) and response to same stimulus after drug wash-in (bottom). Right: Effect of IEM-1460 (left) and vehicle (right) as a function of mean # APs fired prior to drug wash-in. IEM-1460 consistently reduced SPN responses to singlets (r(7) = 0.94, p=0.00060, n = 8 cells) whereas vehicle had no such effect (mean effect = 0.28 ± 0.66; p=0.89 for correlational analysis, n = 8 cells). *p<0.05. Dotted error bands indicate 95% confidence interval.
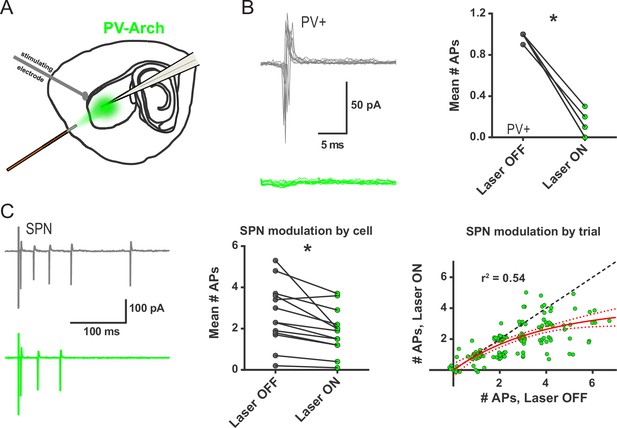
Ex vivo optogenetic inhibition of FSIs selectively reduces evoked multi-action potential SPN responses.
(A) Experimental setup to record cortically-evoked action potentials in cell-attached mode with interleaved optogenetic inhibition of striatal FSIs. (B) Example traces (left) and mean number of APs (right) for evoked FSI firing with laser off (grey) and on (green). 532 nm light strongly inhibits evoked FSI firing (t(5) = 15.54, p=0.000020, n = 6 cells). (C) Evoked SPN action potential firing with interleaved optical inhibition of striatal FSIs. Left: Example traces showing consecutive sweeps of evoked multi-AP SPN firing with laser off (grey) and on (green). Middle: Mean number of evoked SPN APs with laser off (grey) and on (green). Inhibition of striatal FSIs caused SPNs to fire fewer action potentials (t(12) = 3.33, p=0.0060, n = 13 cells). Right: Data in middle plot shown as individual laser ON-OFF paired trials instead of by cell. Black dashed line denotes hypothetical regression line if laser had no effect. Data were jittered in x and y with Gaussian N(0, 0.15) to visualize overlapping points. Single exponential fit consistent with specific laser effect on multi-AP SPN responses (τ=13.78, r2(127)=0.54, n = 130 paired trials from 13 cells). *p<0.05. Dotted error bands indicate 95% confidence interval. Cell type specificity of Arch expression is shown in Figure 2—figure supplement 1.
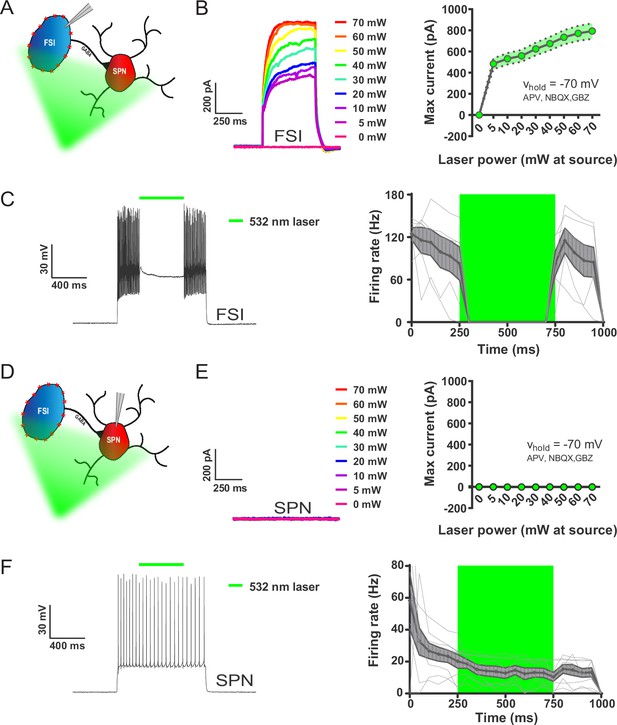
532 nm light selectively inhibits FSIs in PV-Arch mice ex vivo.
(A) Recording configuration used to verify optical inhibition of FSIs in (B-C). (B) Light-driven currents in Arch-expressing FSIs measured in voltage clamp with synaptic blockers. Left: representative traces showing FSI response to increasing intensities of 532 nm light. Right: quantification of light-driven currents in FSIs (n = 4). Dotted error bands indicate SEM. (C) Arch-mediated current suppresses high-frequency firing driven by somatic current injection in FSIs. Left: example trace of FSI response to somatic current injection with an interposed 500 ms pulse of 532 nm light (green bar). Right: Mean FSI responses show that 532 nm light reliably abolishes high-frequency firing (F(1.20, 5.99)=19.66, p=0.0037, n = 6 cells). Fine grey lines indicate individual FSI recordings. Data are represented as mean ±SEM. (D) Left: recording configuration to assess off-target effects of 532 nm light on SPN firing in (E-F). (E) SPN responses to 532 nm light measured in voltage clamp as in (B). Left: representative trace showing SPN response to increasing intensities of 532 nm light. Right: quantification of light-driven currents in SPNs (n = 5). (F) SPN responses to somatic current injection with interposed 532 nm light as in (C). Although analysis of variance showed an effect of laser on SPN firing (F(1.04, 7.27)=9.80, p=0.015, n = 8), this effect was due to an early frequency adaptation which SPNs are known to display in response to suprathreshold excitation (Freiman et al., 2006). SPN firing rates during and after laser stimulation were indistinguishable (p=0.31, n = 8).
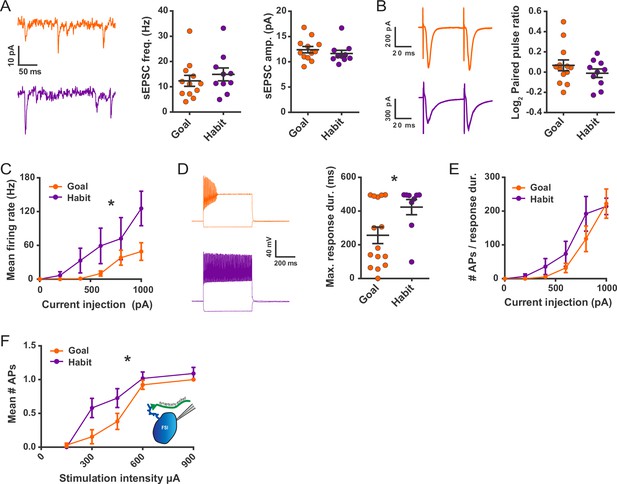
Habit formation enhances sustained high-frequency firing and cortically-evoked action potential firing in DLS FSIs ex vivo.
(A) sEPSCs in FSIs of goal-directed (orange) and habitual (purple) mice. Left: Example sEPSC traces. No effect of training was found in sEPSC frequency (middle, p=0.45, n = 12 and 10 cells) or amplitude (right, p=0.42, n = 12 and 10 cells). (B) Paired-pulse measurements in FSIs of goal-directed and habitual mice. Left: Example traces showing FSI responses to paired single-pulse stimuli spaced 50 ms apart. Right: Habitual behavior was not associated with a change in paired pulse ratio relative to goal-directed behavior (p=0.29, n = 13 and 10 cells). (C) Input-output curve showing mean FSI firing rate in response to a series of increasing current steps. Habitual FSIs fired at an overall higher rate relative to goal-directed FSIs (F(1, 22) = 5.84, p=0.024, n = 15 and 9 cells). (D) FSI response durations, i.e. the time over which FSIs sustain firing. Left: Representative traces show that goal-directed FSIs often are unable to sustain firing for the duration of a 500 ms current step whereas habitual FSIs are typically able to do so. Right: Goal-directed FSIs are less-able to sustain firing than habitual FSIs (U = 34.5, p=0.049, n = 15 and 9 cells). Goal-directed response durations were bimodally distributed (p=0.020, Hartigans’ dip test). (E) Firing rates as in (C) normalized to response duration. When accounting for response duration, no difference in firing rates is observed (p=0.25, n = 15 and 9 cells). (F) Input-output curve showing mean number of synaptically-evoked action potentials fired by goal-directed versus habitual FSIs in response to a series of increasingly strong single-pulse stimuli delivered to cortical afferent fibers. Responses recorded in cell-attached mode. Habitual FSIs fired more readily than goal-directed FSIs in response to afferent activation (F(1,22) = 4.77, p=0.040, n = 13 and 11 cells). *p<0.05. Data are represented as mean ± SEM. Additional behavioral and electrophysiological measures are included in Figure 3—figure supplement 1.
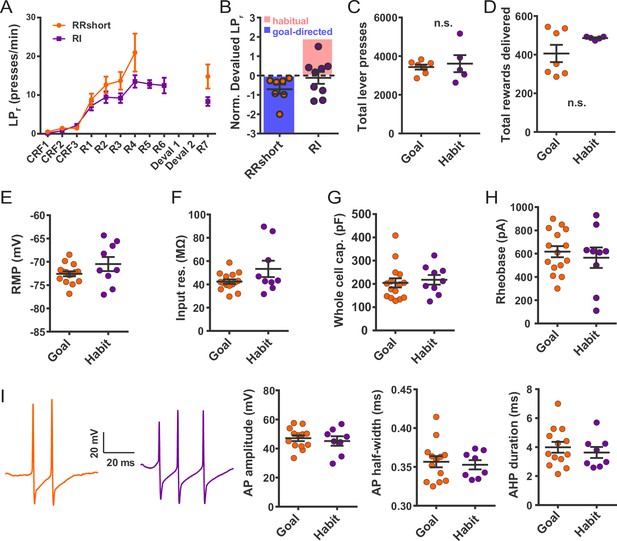
Electrophysiological properties of FSIs from habitual and goal-directed mice.
(A) Learning curves showing lever press rate over training sessions. Mice acquired lever pressing behavior with continuous reinforcement (CRF) of lever presses and were then trained on either random interval (RI) or abbreviated random ratio (RRshort) reinforcement schedules to induce habitual and goal-directed behavior, respectively. A final training session was administered after devaluation testing, and 0–24 hr prior to recording, to mitigate any effects of devaluation testing. (B) Inclusion criteria for analysis of electrophysiological data. RRshort-trained mice that expressed goal-directed behavior (NDLPr <0) and RI-trained mice that expressed habitual behavior (NDLPr ≥0) were included. Mice that expressed modes of behavioral control inconsistent with training, that is, NDLPr <0 for RI-trained mice, were excluded from analysis. (C–D) Goal-directed (orange) and habitual (purple) mice used for group-wise comparisons of electrophysiological properties did not differ in total number of lever presses (p=0.72, n = 7 and 5 mice) or number of rewards delivered (p=0.72, n = 7 and 5 mice, Mann-Whitney U test) over the course of training. (E–H) Passive membrane properties of FSIs in slices from goal-directed and habitual mice. No differences were found for any membrane property (p=0.13, 0.081, 0.67, 0.58, n = 15 and 9 cells). (I) Left to right: representative action potential traces and quantification of action potential amplitude, half-width, and afterhyperpolarization current duration for FSIs from goal-directed and habitual mice. No difference was detected for any waveform property (p=0.60, 0.71, 0.53 n = 13 and 8 cells). Data are represented as mean ±SEM.
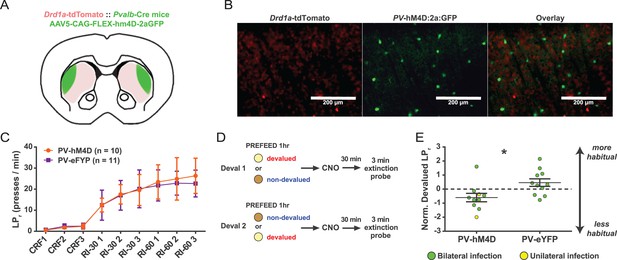
Acute chemogenetic inhibition of FSIs in dorsolateral striatum prevents expression of a learned lever pressing habit.
(A) Diagram of coronal brain section showing tdTomato expression throughout striatum in dSPNs and expression of hM4D:2a:GFP construct in DLS. (B) Epifluorescent images of DLS showing tdTomato in dSPNs (left), GFP in PV+ cells (middle), and overlay (right). (C) Learning curves for hM4D and reporter construct-injected cohorts show that groups did not learn the task differently (p=0.70, n = 10 and 11 mice). (D) Experimental flow of devaluation testing to evaluate habit expression. Upon completion of multi-day training sessions, mice were pre-fed sucrose or grain pellets on alternating days, intraperitoneally administered CNO, and subjected to a 3 min extinction probe test 30 min later. Devalued (sucrose) and non-devalued (grain) lever press rates (LPr) are compared ratiometrically using the normalized devalued LPr (NDLPr) to assess habitual behavior:. (E) Quantification of habit expression in individual subjects using NDLPr. PV-hM4D mice showed less habit expression relative to PV-eYFP controls (t(19) = 2.66, p=0.016, n = 10 and 11 mice). *p<0.05. Data are represented as mean ± SEM. Effect of CNO on absolute LPr in the non-devalued condition is shown in Figure 4—figure supplement 1.
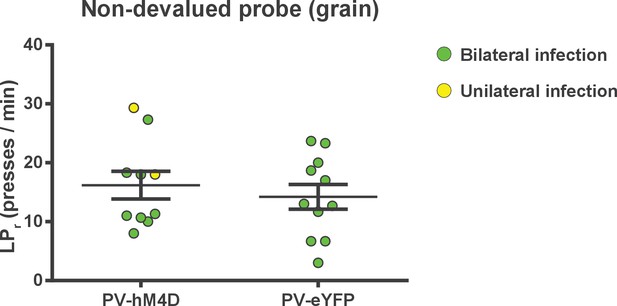
Chemogenetic inhibition of FSIs in dorsolateral striatum does not affect operant lever pressing in general.
Lever press rates during the non-devalued probe test. Mice from both groups were pre-fed a sensory-specific satiety control pellet (grain-only) and administered CNO (5 mg/kg, intraperitoneally) prior to undergoing a 3 min extinction probe test to assess the effect of inhibiting FSIs on operant behavior independent of sensitivity to outcome value, i.e. habit. Mice expressing hM4D and eYFP in FSIs of the DLS did not differ in response rates (p=0.53, n = 10 and 11 mice), indicating that inhibition of DLS FSIs did not affect general lever pressing behavior. Two mice displayed unilateral infection (yellow) as opposed to bilateral (green). Because inclusion or exclusion of these data did not affect statistical results for any behavioral measure, data were included and indicated as above. Data are represented as mean ±SEM.
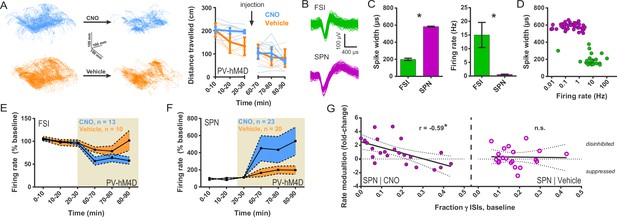
Chemogenetic inhibition of FSIs in DLS exerts a strongly disinhibitory net effect and selective excitatory effect on striatal output.
(A) Locomotion before and after CNO or vehicle administration. Left: Example 3D traces showing head position during 30 min recordings before and after i.p. injection of CNO (blue) or vehicle (orange). Right: group-wise quantification of distance travelled shows that CNO- and vehicle-treated subjects did not respond differently to i.p. injections (p=0.16 for interaction of time and treatment, n = 6 and 7 mice). Subjects non-specifically decreased locomotor activity following the i.p. injection procedure (F(11,1) = 49.01, p=2.27×10−5, n = 6 and 7 mice). (B) Representative single-unit waveforms classified as FSIs (top, green) and SPNs (bottom, purple). (C) Waveform properties used for cell type classification. Left: FSI waveforms display a shorter spike width relative to those of SPNs (t(64) = 30.67, p=5.53×10−40, n = 23 FSIs and 43 SPNs). Right: FSIs display higher firing rates than SPNs (t(64) = 4.32, p=0.000056, n = 23 FSIs and 43 SPNs). (D) Classification of single units as FSIs (green) or SPNs (purple) by spike width and firing rate. (E) Time course showing FSI firing rates before (white background) and after (tan background) i.p. injection of CNO (blue) or vehicle (orange). CNO injection decreased FSI firing rate relative to vehicle (interaction between drug and time: F(5,105) = 2.51, p=0.034, n = 13 and 10 FSIs). (F) SPN responses to CNO or vehicle as in (E). CNO injection increased SPN firing rate relative to vehicle (interaction between drug and time: F(5,205) = 2.63, p=0.025, n = 23 and 20 SPNs). (G) Linear regression of fold-change (log2 post/pre) in firing rate after CNO (left) or vehicle (right) injection against the baseline fraction of ISIs in the gamma frequency band. SPNs with higher fractions of gamma-frequency ISIs at baseline are more likely to decrease firing rate when FSIs are inhibited with CNO (r(22) = −0.59, p=0.0032, n = 23 cells) whereas vehicle caused no change in firing rate that could be predicted by baseline fraction of gamma ISIs (p=0.92, n = 20 cells). *p<0.05. Data are represented as mean ± SEM. Example units before and after CNO shown in Figure 5—figure supplement 1.
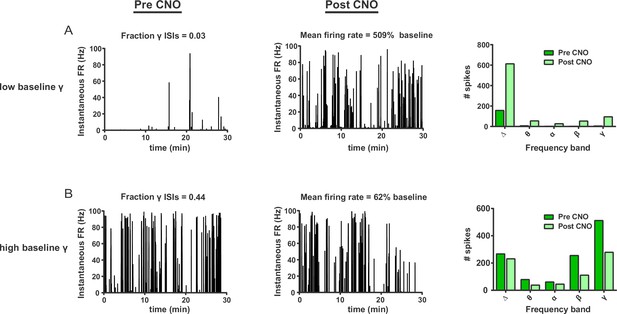
FSIs bidirectionally modulate firing rates as a function of baseline gamma spiking activity in individual SPNs.
(A) Instantaneous firing rate of a representative SPN with a low fraction of gamma ISIs before (left) and after (middle) i.p. injection of CNO (5 mg/kg). Baseline fraction of gamma-frequency ISIs for this SPN was 0.03 (3% of all ISIs) and inhibition of FSIs via CNO i.p. caused a 509% increase in overall firing rate. Right: raw quantification of spike counts within each frequency band before (dark green) and after (light green) CNO i.p. (B) Instantaneous firing rate, as in (A), of a representative gamma-rich SPN. Baseline fraction of gamma-frequency ISIs = 0.44. Suppression of FSI activity decreased firing rate to 62% baseline. Right: raw quantification of spike counts within each frequency band as in (A).
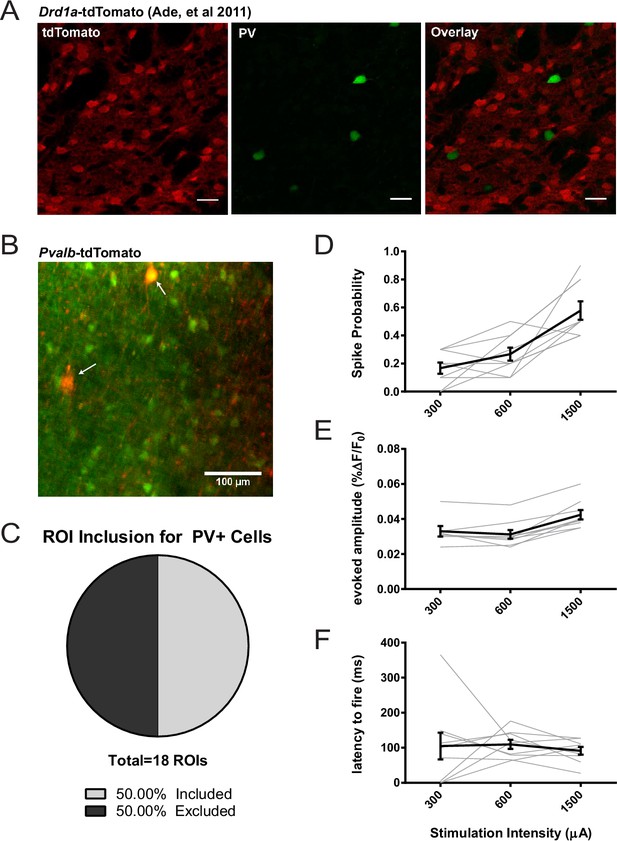
Assessing potential contribution of FSIs to SPN 2PLSM datasets.
(A) Figure panel adapted from Ade et al. (2011) showing that the BAC transgenic Drd1a-tdTomato mouse that was used in 2PLSM experiments described in Figure 1 does not express tdTomato in striatal PV+ neurons. tdTomato signal (left) was not detected in neurons staining positive for the parvalbumin antigen (middle) as shown in overlay (right). Scale bars represent 25 μm. (B) Field of view for 2PLSM calcium imaging of PV+ neurons in Pvalb-Cre/Ai9 mice which Cre-dependently express tdTomato. Fura-2-loaded PV+ neurons were identified by transgenic expression of tdTomato (indicated by white arrows) and overlapping fura-2 signal (green) (C) Pie chart showing that only 50% of transgenically-labelled PV+ neurons are sufficiently loaded with fura-2 and pass other ROI criteria to be included in 2PLSM calcium imaging analysis (n = 18 cells). (D-F) Firing properties of PV+ neurons, as a function of stimulation intensity at cortical afferent fibers, including spike probability (D), amplitude of evoked calcium transients (E), and latency to fire (F) (n = 9 cells).
Tables
Details of sample sizes.
Table showing source of sample sizes for each subfigure in the study. Ex: Fig. 2C shows N = 13 cells from 11 slices and 6 mice.
Figure | Cells | Slices | Mice |
---|---|---|---|
1D | 139 | 5 | 2 |
1E | 139 | 5 | 2 |
1F | 52 independent pairs | 5 | 2 |
2B | 6 | 5 | 3 |
2C | 13 | 11 | 6 |
3A | 22 | 21 | 12 |
3B | 23 | 21 | 12 |
3C | 24 | 20 | 12 |
3D | 24 | 20 | 12 |
3E | 24 | 20 | 12 |
3F | 24 | 23 | 12 |
4C | N/A | N/A | 21 |
4E | N/A | N/A | 21 |
5A | N/A | N/A | 11 |
5C | 66 | N/A | 11 |
5E | 23 | N/A | 11 |
5F | 43 | N/A | 11 |
5G (CNO) | 23 | N/A | 5 |
5G (Vehicle) | 20 | N/A | 6 |
1 - figure supplement 1A | 6 | 6 | 1 |
1 - figure supplement 1B | 139 | 5 | 2 |
1 - figure supplement 2 (IEM) | 8 | 8 | 6 |
1 - figure supplement 2 (Veh) | 8 | 8 | 5 |
2 - figure supplement 1B | 4 | 4 | 2 |
2 - figure supplement 1C | 6 | 6 | 4 |
2 - figure supplement 1E | 5 | 5 | 2 |
2 - figure supplement 1F | 8 | 7 | 1 |
3 - figure supplement 1A | N/A | N/A | 16 |
3 - figure supplement 1B | N/A | N/A | 16 |
3 - figure supplement 1C | N/A | N/A | 12 |
3 - figure supplement 1D | N/A | N/A | 12 |
3 - figure supplement 1E | 24 | 20 | 12 |
3 - figure supplement 1F | 24 | 20 | 12 |
3 - figure supplement 1G | 24 | 20 | 12 |
3 - figure supplement 1H | 24 | 20 | 12 |
3 - figure supplement 1I | 21 | 19 | 12 |
4 - figure supplement 1 | N/A | N/A | 21 |
5 - figure supplement 2A (Pre CNO) | 23 | N/A | 5 |
5 - figure supplement 2A (Pre Vehicle) | 20 | N/A | 6 |
5 - figure supplement 2B (Pre CNO) | 23 + 23 rate-matched simulations | N/A | 5 |
5 - figure supplement 2B (Pre Vehicle) | 20 + 20 rate-matched simulations | N/A | 6 |
Additional files
-
Transparent reporting form
- https://doi.org/10.7554/eLife.26231.017