Actin-based protrusions of migrating neutrophils are intrinsically lamellar and facilitate direction changes
Figures
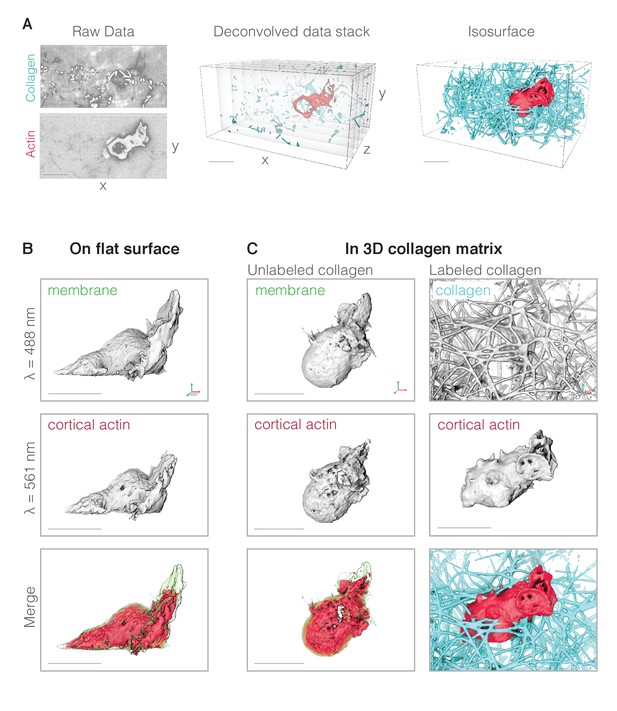
Data processing and visualization.
(A) Data processing pipeline. Left: representative example of raw LLSm data showing a single image plane that passes through a neutrophil-like HL-60 cell (bottom panel) migrating through a collagen network (top panel). Resolution is ~230 in X and Y,~370 in Z. Collagen is directly labeled with fluorescein and the cell’s actin cytoskeleton is highlighted using a utrophin-based probe (see below). Middle: the raw data are deconvolved into a pair of single-color vertical image stacks (here shown merged). Right: the vertical image stacks are then rendered as three-dimensional isosurfaces in UCSF Chimera. (B) Representative fluorescence iso-surface images of an HL-60 cell expressing markers for the plasma membrane (top) and actin cytoskeleton (middle) and crawling across a fibronectin-coated glass coverslip. Top: fluorescence of plasma membrane-anchored palmitoylated m-Emerald. Middle: fluorescence of cortical actin filaments in the cell body labeled by Utrophin-mCherry. Bottom: false-color, three-dimensional overlay of the membrane-mEmerald and Utrophin-mCherry images. (C) Representative fluorescence iso-surface images of HL-60 cells migrating through unlabeled (left column) and fluorescently labeled (right column) three-dimensional collagen networks. Scale bars = 10 µm. Axes indicate relative orientation across rotated views. For cells crawling on a 2D surface, the orientation of the coverslip is indicated by gray shading. Unless otherwise specified, cells were illuminated with 488 and 560 nm light at 37C in 1 × HBSS supplemented with 3% FBS, 1 × pen/strep, and 40 nM of the tripeptide formyl-MLP (to stimulation migration).
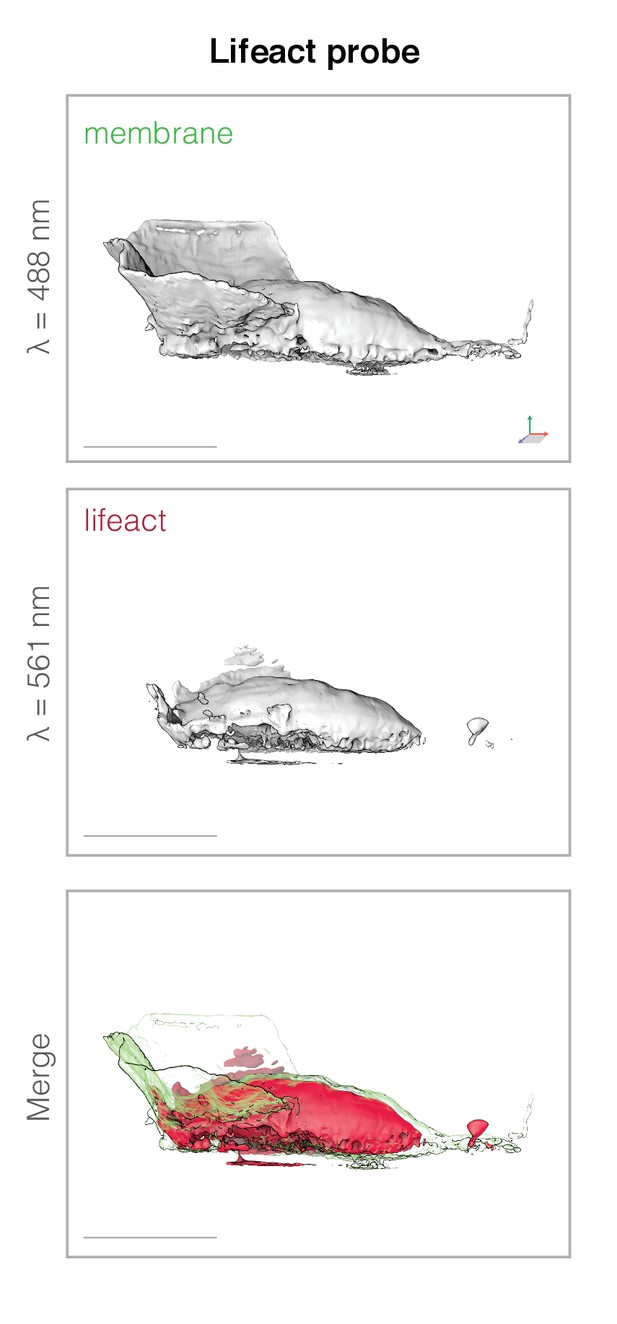
A Lifeact-based fluorescent probe does not label filamentous actin in HL-60 pseudopods.
Top: UCSF Chimera rendering of a fluorescence isosurface of a plasma membrane probe (palmitoylated m-Emerald) expressed in a motile HL-60 cell. Middle:a similar surface rendering of mCherry-Lifeact fluorescence in the same cell. This Lifeact fusion construct is identical to the mCherry-Utrophin fusion used throughout this work, except for the substitution of the F-actin binding Lifeact peptide (Riedl et al., 2008)for the Utrophin CH-ch domain. Bottom: three-dimensional merge of the membrane-mEmerald and mCherry-Lifeact fluorescence. The overlay shows that, similar to the Utrophin probe, Lifeact does not accumulate in lamellar pseudopods. Axes as indicated in Figure 1. Scale bar = 10 µm.
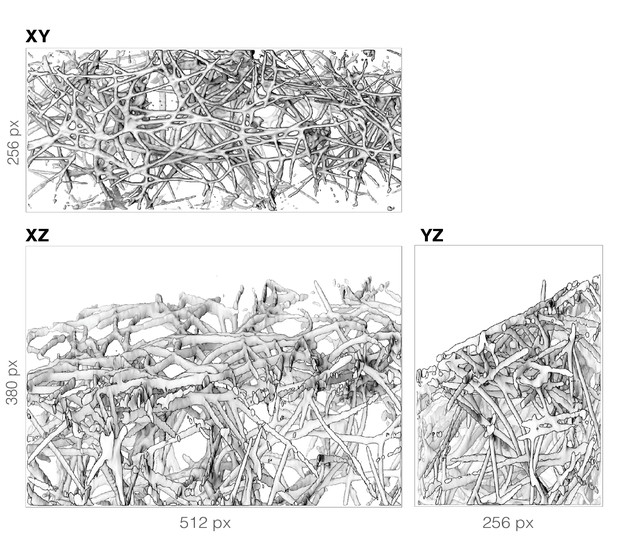
Comparison of isosurface views normal to the XY, YZ, and XZ imaging planes, illustrating the near-isotropic resolution of lattice light sheet microscopy.
Top panel: three-dimensional surface rendering of fluorescein-conjugated collagen fluorescence, oriented so that the viewing angle is normal to the microscope image plane (XY), and parallel to the optical axis of the microscope (Z-axis). Bottom left panel: the same labeled collagen matrix as above but with the viewing angle rotated 90 degrees so that the optical axis has become the vertical axis of the rendering. Bottom right panel: the same collagen matrix as in the other two panels but with the viewing angle rotated 90 degrees within the image plane of the microscope. The three-dimensional stack was rendered in Cinema4D, with a top (X,Y), front (X,Z), and side (Y,Z) view. Numbers on the axes represent image dimensions in pixels. Scale bar = 10 µm.
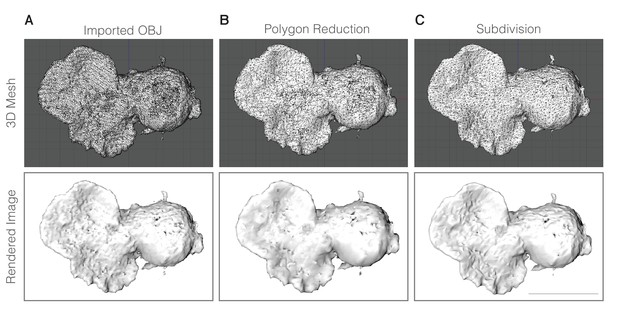
Mesh processing for figures, showing both the polygon counts (or ‘three-dimensional Mesh’) and the rendered images.
(A) Initial Wavefront file exported from UCSF Chimera. (B) Results of applying the polygon reduction tool in Cinema4D. (C) Final version using Polygon Subdivison, a method of calculating a smoothed surface from a reduced polygon mesh. Scale bar = 10 µm.
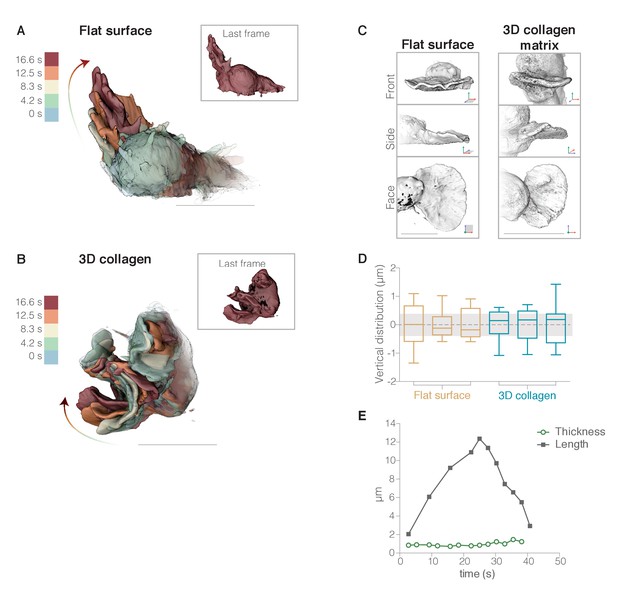
Neutrophils form lamellar pseudopods regardless of whether they are crawling on two-dimensional surfaces or moving through complex three-dimensional environments.
(A–B) To illustrate the morphology of rapidly growing membrane protrusions we overlaid multiple fluorescence iso-surface images taken at different time points of individual HL-60 cells expressing a membrane probe (palmitoylated m-Emerald). Individual surface renderings are tinted according to time. Insets show each cell at the final time point. (A) HL-60 cell crawling across a fibronectin-coated glass coverslip. (B) HL-60 cell crawling through a polymerized collagen mesh. (C) Three-dimensional surface renderings of two pseudopods from two different cells, one crawling on fibronectin-coated glass (left) and one moving through a collagen fiber matrix (right). Each LLSm dataset is rendered from three different viewing angles: en face (top), from the side (middle), and from above (bottom). For more examples, see Figure 2—figure supplement 1. (D) Box and whisker plot (with median, quartiles, and range) of vertical error (estimate of flatness) for each pseudopod, calculated by measuring the average Euclidean distance between the original points on the leading edge and a reference plane with a width equal to the average measured width of lamellar pseudopods (gray band). The first three are measurements from cells crawling across a flat surface (brown), and the next three from cells crawling through a polymerized collagen network (blue). (E) Example of a lamellar pseudopod retaining a similar thickness (green circles) during extension and retraction as indicated by the distance from the tip of the pseudopod to the cell body (black squares). Axes as indicated in Figure 1. All scale bars = 10 µm.
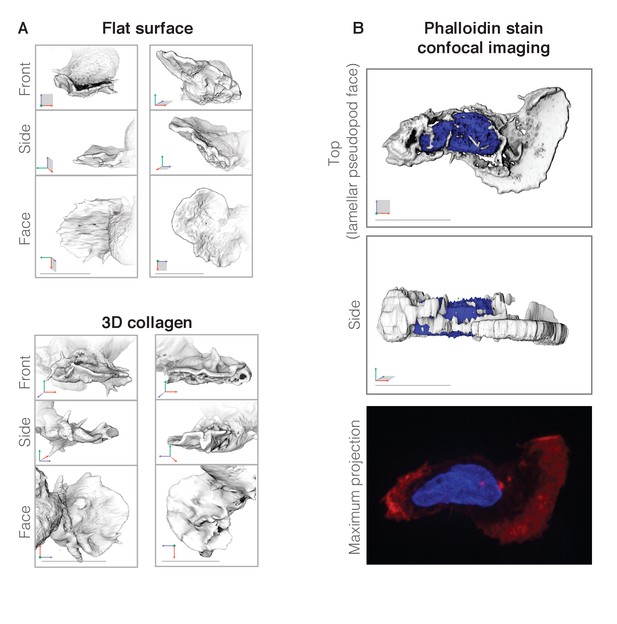
Neutrophils form lamellar pseudopods.
(A) Additional three-dimensional visualizations of single time points from four independent cells (two on glass and two in matrix) forming lamellar pseudopods shown en face, from the side, and from the top. (B) Spinning disk confocal imaging of a fixed cell with a lamellar pseudopod. The resulting three-dimensional stack was rendered in UCSF Chimera, with a top (X,Y) view and side (Z,Y) view shown. The bottom panel is a maximum intensity projection of phalloidin staining (red) and DNA stained with DAPI (blue), revealing that planar pseudopods are filled with polymerized actin. Axes as indicated in Figure 1. Scale bars = 10 um.
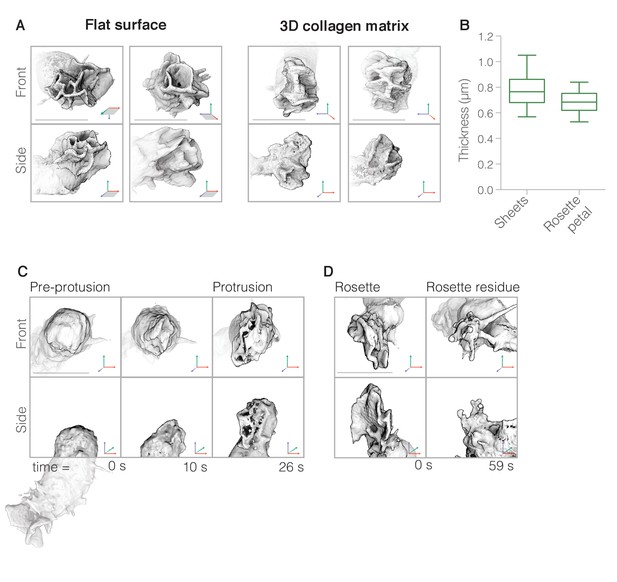
Neutrophils build complex pseudopods (‘rosettes’) formed of multiple lamellar sheets.
(A) Three-dimensional visualizations of rosettes built by cells crawling on two-dimensional surfaces (left) as well as through unlabeled collagen meshes (right). Single timepoints of two independent cells from each condition are shown from the side (top panels) and en face (bottom panels). (B) Box and whisker plot showing that simple lamellar pseudopods have similar thickness as individual petals of complex rosette pseudopods. (n = 18 pseudopods, from 6 cells from two biological replicates). (C) Enlarged views of three time points of a portion of a single cell (whole cell shown below) showing initial stages of rosette pseudopod formation viewed en face (top) and from the side (bottom). Note that, in this instance, the new pseudopod emerges from the rear of the cell, opposite to a previously formed pseudopod. (D) Two time points showing the dissolution of a separate pseudopod shown en face (top) and from the side (bottom). For (A) and (C): Axes as indicated in Figure 1. Scale bars = 10 µm.
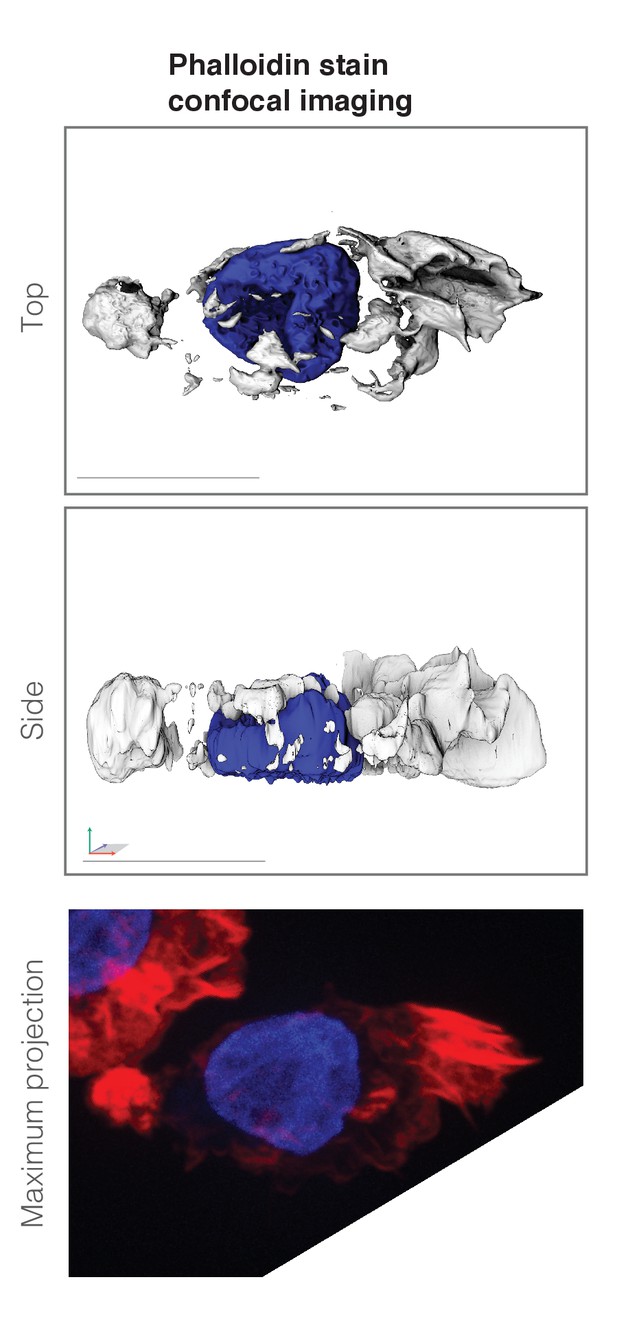
Spinning disk confocal image of fixed cell with a complex rosette pseudopod.
The resulting three-dimensional stack was rendered in UCSF Chimera, with a top (X,Y) view and side (Z,Y) view shown. (Bottom) Maximum intensity projection of phalloidin staining (red) reveals rosette pseudopods are filled with polymerized actin, with DNA stained with DAPI (blue). Scale bar = 10 µm. The bottom right corner of the data stack was removed because a second cell interfered with three-dimensional visualization of the data. Axes as indicated in Figure 1.
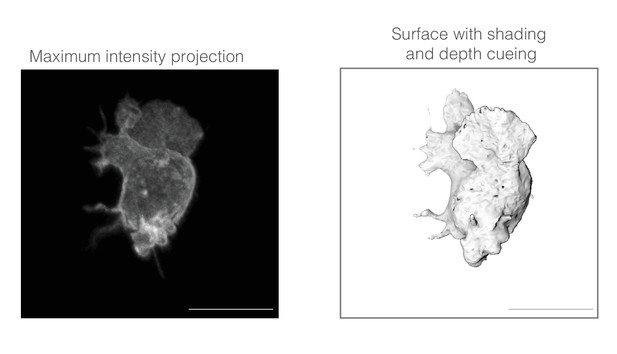
Maximum intensity projection of deconvolved lattice light sheet microscopy data (left) compared to surface rendering (right).
This particular time point was chosen to highlight the need for appropriate visualization tools to determine spatial relationships between structures protruding from the cell surface.
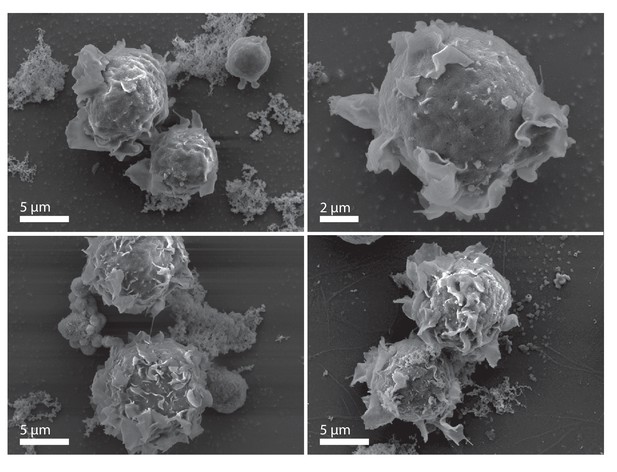
Scanning electron micrographs of Jurkat T cells on coverslips.
Planar protrusions on these lymphocytes are reminiscent of those we observe in HL-60 cells. Scale bar = 2 µm for top right panel and 5 µm for other panels.
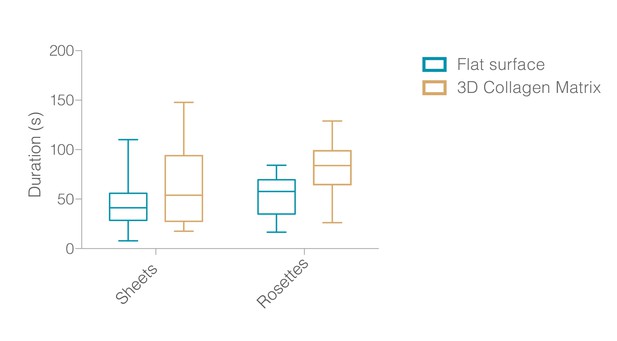
Box and whisker plot of lifetimes of simple (left) and rosette (right) pseudopods built by cells crawling across coated glass surfaces (blue) and through collagen meshes (brown).
Nine cells were analyzed from three independent experiments.
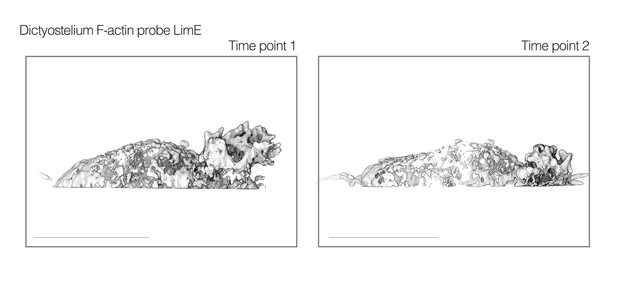
UCSF Chimera visualization of two time points of published Dictyostelium cell dataset imaged using Bessel beam microscopy (Gao et al., 2014) showing rosette-like protrusions.
Cell is expressing the Dictyostelium F-actin probe LimE (Gao et al., 2014). Scale bar = 10 µm.
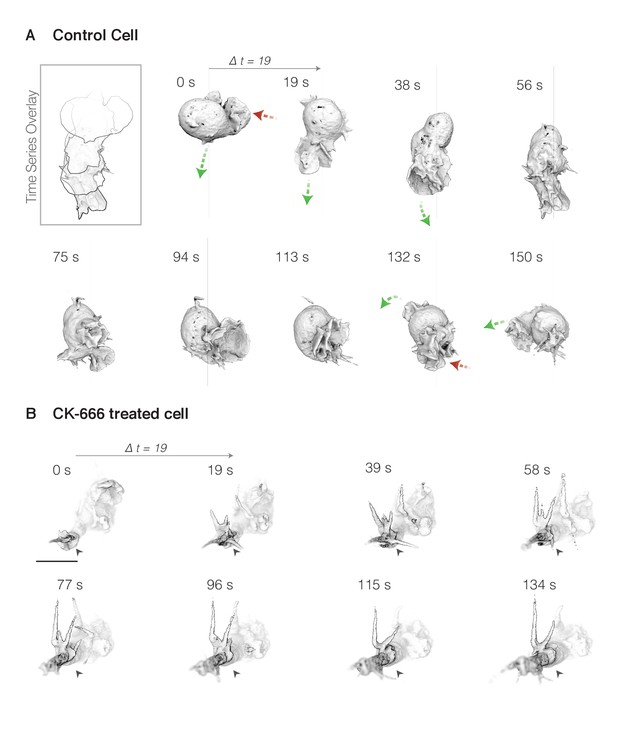
Both simple lamellar and rosette pseudopods are formed de novo from the cell body and require Arp2/3 activity for assembly.
(A) Surface rendering of membrane-labeled control HL-60 cell at ~19 s intervals. Green arrows highlight growing pseudopods, while shrinking pseudopods are indicated by red arrows. Inset (top left): overlay of cell outline at each time point to highlight pseudopod extension and cell movement. (B) A similar cell treated with the Arp2/3 inhibitor CK-666 for ten minutes prior to imaging. A single plane of the three-dimensional image is darkened and indicated with a black arrow to highlight the extension of aberrant tubular protrusions. Scale bar = 10 µm.
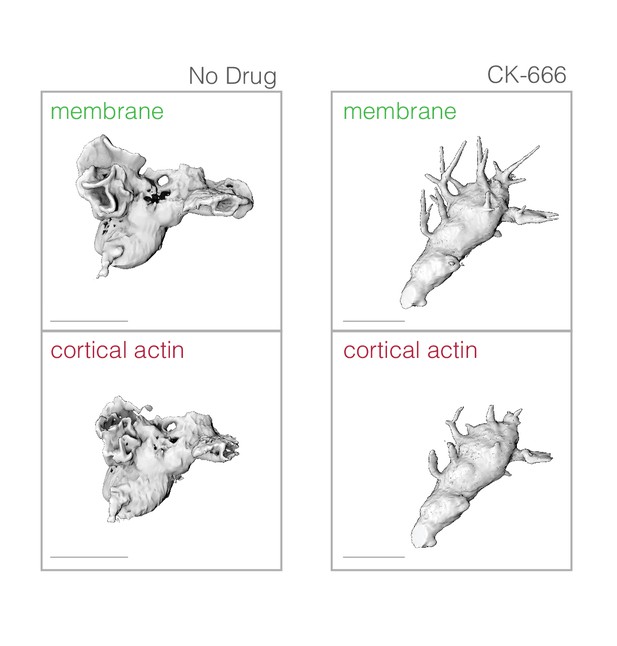
Comparison of protrusions formed by control (Left column) and CK-666 treated (Right column) cells expressing both membrane (Top row) and cortical actin Utrophin-based actin probes (Bottom row).
https://doi.org/10.7554/eLife.26990.027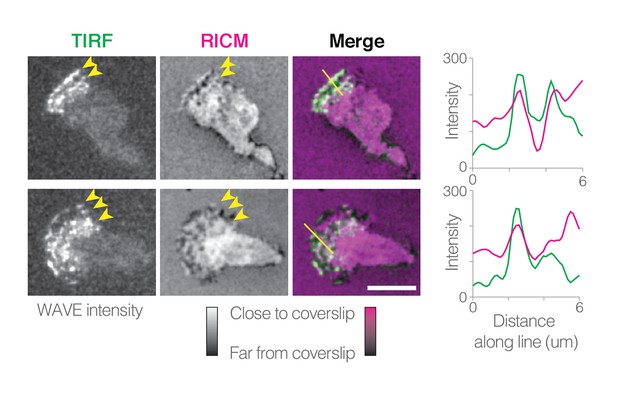
WAVE complex localizes to ventral protrusions.
Time-lapse images of WAVE complex fluorescence in TIRF (left) and ventral cell surface distance from the glass coverslip in reflective interference contrast microscopy (RICM, middle), and overlay (right). For RICM, the colormap has been inverted from the raw data: dark areas represent larger distances between the coverslip and the ventral cell surface; bright white (or magenta, in the overlay) indicates close contact of the cell onto the glass. Line scans of the TIRF and RICM intensity along the lines shown on image overlays (far right). Scale bars = 5 µm. See also Video 13 for dynamic localization and linescans.
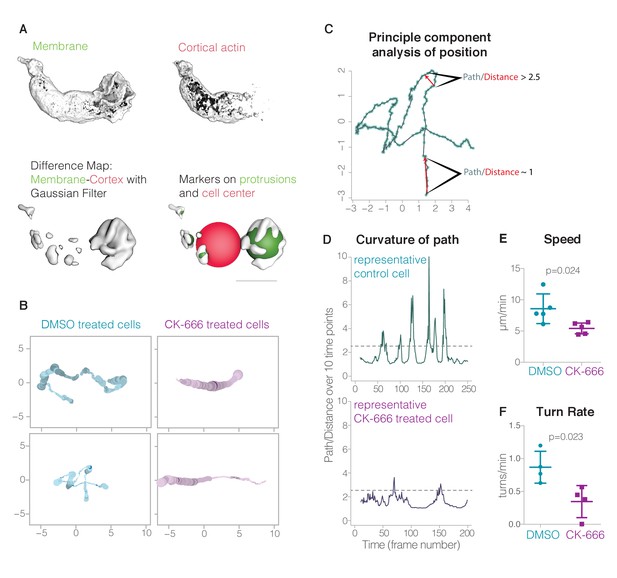
Lamellar pseudopods are associated with cell turning, but are not required for locomotion.
(A) Automatic pseudopod detection relies on the relative exclusion of the Utrophin-based actin probe from the dynamic actin networks within pseudopods; pseudopods are identified by first subtracting the cortical actin signal (top right) from the membrane signal (top left), resulting in a difference map (bottom left). Pseudopods (bottom right) are then defined as enclosed volumes that fulfill all of the following three criteria: larger than 1 µm3, within 15 µm of cell center, and outside the cell body (or larger than 15 um3). The cell body is defined by a sphere with a volume equal to the volume of the actin map; if the center of the enclosed volume was inside this sphere, it was not considered a pseudopod unless its volume exceeded 15 um3. In Videos 14,15, the cell body is indicated by a yellow ball. (B) Plots resulting from a principal component analysis of cell position. At various points along a cell’s track, the color saturation denotes the number of pseudopods and the line thickness indicates the pseudopod distribution, or the ratio of the auxiliary pseudopod volume to the total pseudopod volume. Two examples each of control cells and CK-666 treated cells are shown. (C) The results of a principal component analysis of the cell position in three-dimensional space for each time point (blue dots). Low curvature, where the path length is close to the distance traveled by the cell in ten time points, and high curvature, where the path length is 2.5 times longer than the distance traveled, are both indicated by red arrows. (D) Curvature of path of a representative control cell and a CK-666 treated cell. (E) Centroid speed of control cells and CK-666 treated cells (n = 5 cells from at least two independent experiments, p value = 0.024). Error bars represent standard deviation. (F) Turn rates of control cells (n = 4 cells over a total of 20.7 min, from at least two independent experiments) and CK-666 treated cells (n = 5 cells over a total of 13.7 min, from at least two independent experiments). Error bars represent standard deviation.
-
Figure 6—source code 1
‘protrusions_chimera_script.py’ Python script used to calculate protrusion volumes with UCSF chimera.
This outputs two text files: one containing data for pseudopod volumes at each time point and one of the angles between the main pseudopod and the cell path.
- https://doi.org/10.7554/eLife.26990.035
-
Figure 6—source code 2
‘cell protrusions analysis functions.R’ Analysis script for use in R to calculate and plot the path characteristics, and the relationships with pseudopod activity.
- https://doi.org/10.7554/eLife.26990.036
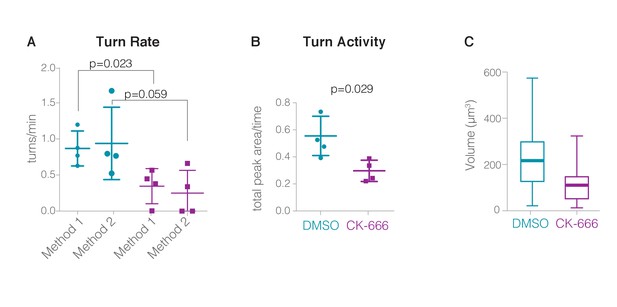
Calculating turning points (A) Turn rates (calculated by two distinct methods) of control cells (n = 4 cells over a total of 20.7 min, from at least two independent experiments) and CK-666 treated cells (n = 5 cells over a total of 13.7 min, from at least two independent experiments).
Error bars represent standard deviation. (B) Comparison of Turn Activity, calculated as the area under the curvature-of-path plots for the cells shown in (A). Error bars represent standard deviation. (C) Box-and-whisker plot of total volume of largest pseudopod. Control (average ±SD, N): 184.0 ± 97.9 µm3, 980 time points; CK666 (average, SD, N): 110.5 ± 64.6 µm3, 600 time points.
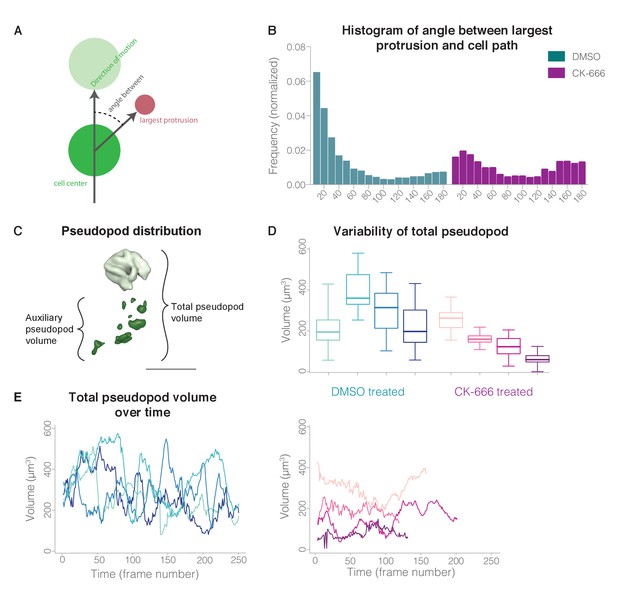
Effects of Arp2/3 inhibitor on pseudopod behavior.
(A) Schematic showing how the angle between main pseudopod and cell path is calculated. (B) Comparison of angle between the largest pseudopod and the cell motion trajectory for control and CK-666 treated cells shown in Figure 6—figure supplement 1. Each histogram is normalized to a sine curve to compensate for expected normal distribution of the angle between two random vectors in three-dimensional space. (C) Pseudopod distribution is determined as the ratio of the auxiliary pseudopod volume to the total volume. (D) Box-and-whisker plot of pseudopod total volume variability for control and CK-666 treated cells. (E) Variability of total pseudopod volume over time, with the same 8 cells as in (D) shown.
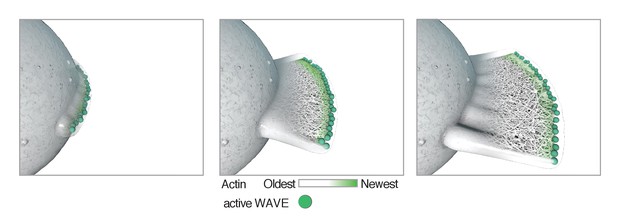
Model showing how localization of Arp2/3 activator (activated WAVE complex, green) could result in the formation of a lamellar protrusion independent of surface interactions.
Once formed, a flat protrusion would continue growth as a planar projection as described (Schmeiser and Winkler, 2015).
Videos
Example of lamellar pseudopods formed by cells crawling on a flat surface (fibronectin-coated glass coverslip). Video plays at 10.5 × real time.
https://doi.org/10.7554/eLife.26990.006Another example of lamellar pseudopods formed by cells crawling on a flat surface (fibronectin-coated glass coverslip).
Video plays at 11 × real time. See also Video 1.
Example where sheets never come into contact with surface.
Because the sample is held vertically in the imaging chamber, edges of cells falling off of the coverslip can pass through the field of view, seen here as objects flowing from right to left. Video plays at 9.8 × real time.
Another example where sheets never come into contact with surface.
Video plays at 11 × real time. See also Video 3.
Example of lamellar pseudopods formed by cells crawling through three-dimensional collagen networks. Video plays at 9.5 × real time.
https://doi.org/10.7554/eLife.26990.010Another example of lamellar pseudopods formed by cells crawling through three-dimensional collagen networks.
Please note that the stage is repositioned several times because the cell migrates out of the field of view. Video plays at 10 × real time. See also Video 5.
Example of ‘rosette’ pseudopods built by cells crawling on flat surface (fibronectin-coated glass coverslips). Video 7 plays at 11 × real time.
https://doi.org/10.7554/eLife.26990.012Another example of ‘rosette’ pseudopods built by cells crawling on flat surface (fibronectin-coated glass coverslips).
Video plays at 11 × real time. See also Video 7.
Example of ‘rosette’ pseudopods built by cells crawling through polymerized collagen networks.
Video plays at 11 × real time.
Another example of ‘rosette’ pseudopods built by cells crawling through polymerized collagen networks.
Video plays at 10 × real time. See also Video 9.
Example of CK-666 treated cells migrating through a polymerized collagen network.
Video plays at 11 × real time.
Another example of CK-666 treated cells migrating through a polymerized collagen network.
Video plays at 11 × real time. See also Video 11.
Videos of WAVE fluorescence in TIRF (left) and ventral cell surface distance from the glass coverslip in RICM (middle), and overlay (right).
For RICM, dark areas represent larger distances between the coverslip and the ventral cell surface; bright white (or magenta, in the overlay) indicates close contact of the cell onto the glass. Two different example cells are shown. Graphs show TIRF and RICM intensity along lines drawn on image overlays (far right). Video plays at 5 × real time.
Video highlighting automation of protrusion detection in control cells. Video plays at 10 × real time.
https://doi.org/10.7554/eLife.26990.030Video 15 Video highlighting automation of protrusion detection in CK666 cells. Video plays at 11 × real time.
https://doi.org/10.7554/eLife.26990.031Additional files
-
Transparent reporting form
- https://doi.org/10.7554/eLife.26990.038