Molecular basis of fatty acid taste in Drosophila
Abstract
Behavioral studies have established that Drosophila appetitive taste responses towards fatty acids are mediated by sweet sensing Gustatory Receptor Neurons (GRNs). Here we show that sweet GRN activation requires the function of the Ionotropic Receptor genes IR25a, IR76b and IR56d. The former two IR genes are expressed in several neurons per sensillum, while IR56d expression is restricted to sweet GRNs. Importantly, loss of appetitive behavioral responses to fatty acids in IR25a and IR76b mutant flies can be completely rescued by expression of respective transgenes in sweet GRNs. Interestingly, appetitive behavioral responses of wild type flies to hexanoic acid reach a plateau at ~1%, but decrease with higher concentration, a property mediated through IR25a/IR76b independent activation of bitter GRNs. With our previous report on sour taste, our studies suggest that IR-based receptors mediate different taste qualities through cell-type specific IR subunits.
https://doi.org/10.7554/eLife.30115.001Introduction
Detection of food compounds plays a critical role in feeding behavior. Likewise, the ability to avoid harmful chemicals is essential to navigate the evaluation of suboptimal food sources that might contain toxins or are contaminated with microorganism producing harmful chemicals. Hence, animals have evolved chemoreceptors to detect and discriminate between nutritious food chemicals, and chemoreceptors that sense non-nutritious and potentially harmful chemicals. Receptors for sugars, amino acids and fatty acids have been identified in mammals (Cartoni et al., 2010; Galindo et al., 2012; Max et al., 2001; Montmayeur et al., 2001; Nelson et al., 2001; Zhao et al., 2003), and other vertebrates (Ishimaru et al., 2005), albeit in some, receptor types for certain nutritious chemicals have been lost (Baldwin et al., 2014; Jiang et al., 2012; Jin et al., 2011; Lagerström et al., 2006; Zhao et al., 2012). In mice, these various types of receptors are expressed in distinct populations of taste cells located mainly in taste buds on the tongue. For example, three different groups of cells respond to calorie-rich carbohydrates, amino acids and fatty acids, respectively, while a separate group of cells is dedicated to the detection of salt (NaCl), a modest amount of which is essential for many cellular functions. Lastly, two functionally distinct types of taste cells detect diverse organic, but repugnant chemicals (phenols, alkaloids etc.) and acids, perceived as bitter and sour, respectively (Liman et al., 2014). Thus, the intrinsic quality of different taste chemicals is initially encoded in the taste periphery, which is transmitted through dedicated sensory pathways, a concept generally referred as the labeled line hypothesis of taste coding (Dethier, 1978; Di Lorenzo, 2000; Spector and Travers, 2005).
With the exception of sugars, perception of nutritious chemicals is relatively poorly understood in insects. In Drosophila, food and other soluble chemicals are detected by taste sensilla (comparable to mammalian taste buds) located on the labial palps and the legs. Most of these sensilla harbor four Gustatory Receptor Neurons (GRNs), each of which is thought to mediate a taste quality: sweet, water, low salt, and bitter/high salt (Liman et al., 2014; Vosshall and Stocker, 2007). The only appetitive taste modality that has been studied in detail is that of sweet taste, elicited by a relative small group of dietary sugars, such as glucose, sucrose, trehalose, maltose, melizitose and raffinose, found mostly in fruits. Genetic studies, combined with behavioral analysis, electrophysiology and Ca2+ imaging have revealed that a group of eight sugar Gustatory receptor genes (Gr5a, Gr61a and Gr64a-f) is mostly responsible for the detection of sugars (Dahanukar et al., 2007; Fujii et al., 2015; Jiao et al., 2008; Miyamoto et al., 2013; Slone et al., 2007; Yavuz et al., 2014). With the exception of Gr5a, all sugar Gr genes are expressed at most in a single GRN per sensillum (Fujii et al., 2015; Slone et al., 2007), which is generally referred to as the sweet GRN. Indeed, electrophysiological studies on a small number of labellar taste sensilla (Dahanukar et al., 2007) and both Ca2+ imaging and electrophysiological recordings on tarsal sensilla of the most distal segment of the forelegs (Ling et al., 2014; Miyamoto et al., 2013; Yavuz et al., 2014) have confirmed that sweet GRNs respond specifically to sugars, but not to bitter compounds or salt. Interestingly, sweet GRNs vary in the number of expressed sugar Gr genes (Fujii et al., 2015), providing different GRNs with the potential for distinct sugar sensing specificities.
In contrast to sweet taste, little is known about the cellular and molecular basis of amino acid and fatty acid taste in insects. While both these nutrients are essential for growth and development during larval life, their relevance in adults is mainly restricted to females, which require fat and protein for the production of eggs. Evidence for appetitive taste of fatty acids in Drosophila has been demonstrated using the classical Proboscis Extension Reflex (PER) assay (Masek and Keene, 2013), but whether flies can sense amino acids through their taste sensory system is less clear and appears at least in part to depend on the internal nutrient status (Toshima and Tanimura, 2012). Regardless, no defined set of taste neurons that respond to amino acids have been described to date.
Here, we employed a genetic approach to investigate the cellular and molecular basis for fatty acid taste. Using Ca2+ imaging, we report that tarsal sweet GRNs are activated by fatty acids, and we show that IR25a and IR76b are expressed in numerous GRNs, including sweet GRNs, where they are required for their activation by fatty acids, but not by sugars. RNAi knock-down of a third IR transcript, IR56d, in sweet GRNs also abolished fatty acid responses both at the behavioral as well as the cellular level. In contrast to IR25a and IR76b, which are also required for sour taste and expressed in respective acid sensing GRNs (Chen and Amrein, 2017), expression of IR56d is confined to sweet GRNs, suggesting a more restricted role for this receptor in fatty acid taste.
In contrast to responses to sugars, which increase in a concentration dependent manner to reach a plateau, we observed that wild type flies show maximal PER responses at modest concentrations to one fatty acids, (hexanoic acid), while higher concentrations led to a reduction in PER responses. Interestingly, we found that hexanoic acid also activates bitter GRNs, albeit in an IR25a/IR76b independent manner, suggesting that bitter and sweet GRN activation modulate feeding response to this ligand. Consistent with this hypothesis, inhibition of neural transmission of sweet GRNs abolishes PER responses completely, while the same manipulation of bitter GRNs results in further increase in PER responses to hexanoic acid. These observations suggest a model in which activation of bitter GRNs counteract the activation of sweet GRNs to suppress feeding responses to hexanoic acid, possibly to moderate intake of this nutrient compound.
Results
The notion that sweet GRNs are exclusively tuned to sugar has recently been challenged, as it was shown that flies exhibit sweet GRN -dependent appetitive behavioral responses to fatty acids (Masek and Keene, 2013). Thus, we first wanted to confirm these findings using flies with intact and functionally impaired, tetanus toxin (TNT) expressing sweet GRNs, respectively (Figure 1). Both sets of control flies (Gr64f-GAL4/+ and + /UAS-TNT) showed robust PER responses to fatty acids and the sugar sucrose when tarsi were stimulated, while flies with inactivated sweet GRNs (Gr64f-GAL4/UAS-TNT) lost PER responses to all these food chemicals (Figure 1A). We note that somewhat lower PER responses were elicited when the labellum was stimulated with fatty acids (Figure 1—figure supplement 1). To explore whether fatty acid taste requires the sugar Gr genes, we used a sugar Gr deficient fly strain (Gr5aLexA; ΔGr61a ΔGr64a-f) (Fujii et al., 2015; Yavuz et al., 2014) and tested PER responses to fatty acids and sucrose (Figure 1B). While PER responses to fatty acids were unaffected or higher in these octuple mutant flies, they were severely reduced to sucrose (Figure 1B), indicating that other receptors must be expressed in sweet GRNs to detect fatty acids. Use of different taste receptors for mediating the two taste modalities is consistent with the finding that mutations in norpA, which encodes a phospholipase C, affected PER responses to fatty acids, but not to sugars (Masek and Keene, 2013). Moreover, and consistent with this behavioral phenotype, sweet taste neurons of norpA mutant flies had strongly reduced responses to fatty acids, but not sucrose, using a Ca2+ imaging assay (Figure 1—figure supplement 2).
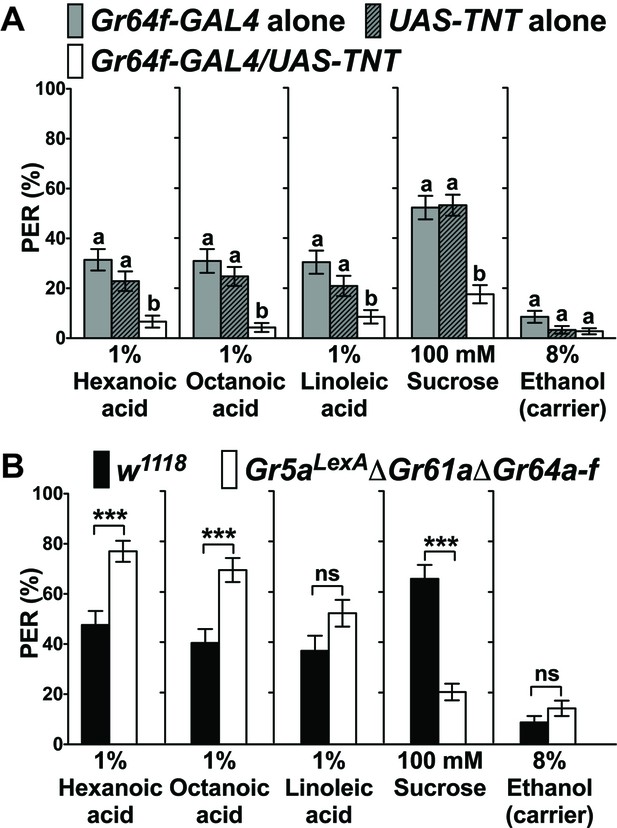
Sweet GRNs, but not sugar Gr genes, are necessary for fatty acid taste.
(A) Sweet GRNs are necessary for fatty acid sensing. Inactivation of sweet GRNs (Gr64f-GAL4/UAS-TNT) leads to loss of PER responses to both sucrose and fatty acids. Control flies (Gr64f-GAL4/+ and UAS-TNT/+) show robust PER responses to fatty acids and sucrose. Each bar represents the mean ± SEM of PER responses (n = 60–70 flies). Bars with different letters are significantly different (Kruskal-Wallis test by ranks with Dunn’s multiple comparison tests, p<0.05). Each y-axis delineates groups for Kruskal-Wallis test. (B) Sugar Gr genes are dispensable for fatty acid sensing. Octuple mutant flies lacking all sugar Gr genes (Gr5aLexA;ΔGr61aΔGr64a-f) exhibit PER responses to fatty acids similar to flies with functional sugar Gr genes (w1118), but they have severely reduced PER response to sucrose. The residual PER responses to sucrose of octuple mutant flies is mediated by Gr43a (Miyamoto et al., 2012). Each bar represents the mean ± SEM of PER responses (n = 42–83 flies). Asterisks indicate a significant difference between the mutant and control flies (Two-tailed, Mann-Whitney U test, ***p<0.001, ns: not significant). Each y-axis delineates groups for Mann-Whitney U test. The genotype of octuple mutant flies is R1 Gr5aLexA; +; ΔGr61a ΔGr64a-f (Yavuz et al., 2014). Source data for summary graphs are provided in Figure 1—source data 1.
-
Figure 1—source data 1
PER responses to fatty acids of flies with impaired neurons and genes.
(A) PER responses of sweet GRNs to fatty acids when inactivated by expression of UAS-TNT. (B) PER responses of octuple mutant flies lacking all sugar Gr genes (Gr5aLexAΔGr61aΔGr64a-f) to fatty acids.
- https://doi.org/10.7554/eLife.30115.007
Broadly expressed IR25a and IR76b are required for PER responses to fatty acids
In mammals, fatty acid taste is mediated by at least two G-protein coupled receptors, GPR40 and GPR120 (Cartoni et al., 2010; Matsumura et al., 2007). However, BLAST searchers showed that no homologs for these genes are found in the Drosophila genome. To identity putative candidates for Drosophila fatty acid taste receptors, we turned our attention to members of the Ionotropic Receptor (IR) gene family. IRs are derived from ionotropic Glutamate Receptors (iGluRs) genes and expanded in number during arthropod evolution, comprising a family of 61 genes in Drosophila (Benton et al., 2009; Croset et al., 2010). The role of IR proteins has been extensively characterized in the olfactory system, where they form multimeric receptors for the detection of an array of different odorant molecules (Abuin et al., 2011; Ai et al., 2013; Benton et al., 2009; Hussain et al., 2016; Silbering et al., 2011), but IR based receptors have also been implicated in temperature sensing (Knecht et al., 2016; Ni et al., 2016), humidity sensing (Enjin et al., 2016; Knecht et al., 2017; Knecht et al., 2016) and taste perception (Croset et al., 2016; Ganguly et al., 2017; Hussain et al., 2016; Koh et al., 2014; Zhang et al., 2013). Of particular interest were IR25a and IR76b, because these two genes are enriched in taste neurons (Cameron et al., 2010). We therefore examined the expression of IR-GAL4/QF drivers for both genes and found that they were indeed expressed in multiple GRNs per sensillum (Figure 2). In tarsal sensilla, their expression largely overlapped (Figure 2F) and included the sweet and bitter GRNs that express sugar and bitter Gr genes, respectively (Figure 2B–E), thus identifying them as possible subunits of fatty acid taste receptors. Both genes were also expressed in multiple neurons per sensillum in the labial palps, albeit co-expression was incomplete in this taste organ (Figure 2—figure supplement 1). To examine a possible role for IR25a and IR76b in fatty acid taste, we conducted PER assays with flies from each mutant strain to hexanoic, octanoic and linoleic acids and found that PER responses were abolished in both IR25a-/- and IR76b-/- flies, a phenotype completely (hexanoic acid and octanoic acid) or partially (linoleic acid) rescued when expression was restored with UAS-IR25a or UAS-IR76b transgenes (Figure 3A and B). Additionally, IR25a-/- and IR76b-/- flies showed normal PER responses to sucrose, indicating that the role of these two genes is specific to fatty acid taste and not required for sweet taste to sugars. Importantly, when IR25a function was provided in sweet GRNs of IR25a-/- flies, their PER responses were restored to levels indistinguishable from controls (Figure 3C). Likewise, expression of IR76b in sweet GRNs alone also rescued PER responses to fatty acids in IR76b-/- flies (Figure 3D). Together, these observations indicate that the functions of IR25a and IR76b are required in sweet GRNs for robust PER responses to fatty acids, but not to sugars.
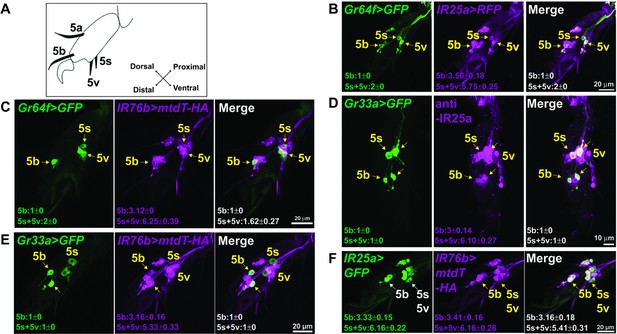
IR25a and IR76b are expressed in numerous taste neurons, including sweet and bitter GRNs.
(A) Drawing of the fifth tarsal segment of the prothoracic leg that is used for immunofluorescence experiments (B–F). Tarsal taste sensilla are indicated. (B and C) IR25a and IR76b are expressed in sweet GRNs. Immunostaining with anti-GFP (green) and anti-mCD8 (B; magenta) or anti-HA (C; magenta) antibodies on whole-mount preparations of the fifth tarsal segment of the prothoracic leg from flies of the genotypes: IR25a-GAL4/UAS-mCD8:RFP; Gr64fLexA lexAop-rCD2:GFP (B) or IR76b-QF UAS-mCD8:GFP/Gr64f-GAL4;QUAS-mtd-Tomato-3xHA/+ (C). Arrows refer to a GRN of a sensillum expressing both Gr64f and the indicated IR genes. (D and E) IR25a and IR76b are expressed in bitter GRNs. Immunostaining with anti-GFP (green) and anti-IR25a (D; magenta) or anti-HA (E; magenta) antibodies on whole-mount preparations of the fifth tarsal segment of the prothoracic leg from flies of the genotypes: Gr33aGAL4/UAS-mCD8:GFP (D) or IR76b-QF UAS-mCD8:GFP/Gr33aGAL4;QUAS-mtd-Tomato-3xHA/+ (E). Arrows refer to a GRN of a sensillum expressing both Gr33a and the indicated IR genes. (F) IR25a and IR76b are largely co-expressed in tarsal GRNs. Immunostaining with anti-GFP (green) and anti-HA (magenta) antibodies on whole-mount preparations of the fifth tarsal segment of the prothoracic leg from flies of the genotypes: IR76b-QF UAS-mCD8:GFP/IR25a-GAL4;QUAS-mtd-Tomato-3xHA/+. Arrows refer to GRNs expressing both IR25a and IR76b genes. Numbers indicate the average count of IR or Gr expressing GRNs/sensillum. Due to close proximity, neurons could not always be associated with either the 5s and 5v sensillum, and the cell count was therefore pooled.
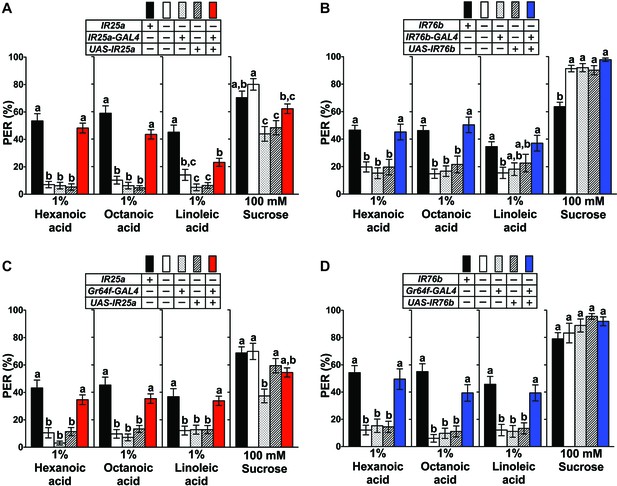
IR25a and IR76b are necessary for behavioral responses to fatty acids.
(A and B) Mutations in IR25a or IR76b abolish PER responses to fatty acids. IR25a (A) and IR76b (B) mutant flies show significantly reduced PER responses to fatty acids, which are rescued by expression of UAS-IR25a and UAS-IR76b respectively, under control of their respective GAL4 drivers. Expression of UAS-IR25a transgenes only partially rescues PER responses to linoleic acid (A). Each bar represents the mean ± SEM of PER responses (n = 34–116 flies). Bars with different letters are significantly different (Kruskal-Wallis test by ranks with Dunn’s multiple comparison tests, p<0.05). Each y-axis delineates groups for Kruskal-Wallis test. Fly genotypes: wild-type: w1118 (black), mutants: IR25a1/IR25a1 and IR76b1/IR76b1 (white), IR25a1 IR25a-GAL4/IR25a1 and IR76b-GAL4/+; IR76b1/IR76b1 (dotted), IR25a1 UAS-IR25a/IR25a1 and UAS-IR76b/+; IR76b1 (lines), and rescue: IR25a1 IR25a-GAL4/IR25a1 UAS-IR25a (red), IR76b-GAL4/UAS-IR76b; IR76b1/IR76b1 (blue). (C and D) Functions of IR25a and IR76b in sweet GRNs are required and sufficient for fatty acid taste. IR25a (C) or IR76b (D) mutant flies show significantly reduced PER responses to fatty acids but not to sucrose. Restoring expression by UAS-IR25a (C) or UAS-IR76b (D) in sweet GRNs of IR25a or IR76b mutant flies is sufficient to rescue the loss of PER responses to fatty acids. Each bar represents the mean ± SEM of PER responses (n = 22–124 flies). Bars with different letters are significantly different (Kruskal-Wallis test by ranks with Dunn’s multiple comparison tests, p<0.05). Each y-axis delineates groups for Kruskal-Wallis test. Fly genotypes: control flies: wild-type: w1118 (black), mutants: IR25a1/IR25a1 and IR76b2/IR76b2 (white), IR25a1 Gr64f-GAL4/IR25a1 and Gr64f-GAL4/+; IR76b2/IR76b2 (dotted), IR25a1 UAS-IR25a/IR25a1 and UAS-IR76b/+; IR76b2/IR76b2 (lines), and rescue: IR25a1 Gr64f-GAL4/IR25a1 UAS-IR25a (red), Gr64f-GAL4/UAS-IR76b; IR76b2/IR76b2 (blue). Source data for summary graphs are provided in Figure 3—source data 1.
-
Figure 3—source data 1
PER responses of IR25a and IR76b mutant flies to fatty acids.
(A) PER responses of IR25a mutant flies to fatty acids. (B) PER responses of IR76b mutant flies to fatty acids. (C and D) PER responses to fatty acids of IR25a (C) or IR76b (D) mutant flies with UAS-IR25a or UAS-IR76b transgenes, respectively, under the control of Gr64f-GAL4.
- https://doi.org/10.7554/eLife.30115.011
Sweet neurons require IR25a and IR76b for fatty acid sensing
To obtain direct evidence that IR25a and IR76b mediate fatty acid sensing in taste neurons, we performed Ca2+ imaging experiments on tarsal sensilla preparations (Miyamoto et al., 2013, 2012) of wild type control flies (w1118), IR25a-/- and IR76b-/- flies that expressed the calcium sensor GCaMP6m in sweet GRNs (Dahanukar et al., 2007; Fujii et al., 2015) (Figure 4). We measured Ca2+ responses in sweet GRNs of the taste sensilla in the fifth tarsal segment of the prothoracic leg (5b, 5s and 5v; Figure 4A; the 5a sensillum is mainly dedicated to pheromone sensing, and no sugar or fatty acid responses were observed in these GRNs). In wild type flies, the sweet GRNs of 5b and 5s sensilla showed robust Ca2+ increases to hexanoic and octanoic acids and modest, but significant increases to linoleic acid (Figure 4B and C). Only weak Ca2+ responses were observed in the 5v-associated sweet GRNs. Importantly, Ca2+ responses were absent in 5b- and 5s-associated sweet GRNs of IR25a-/- or IR76b-/- flies (Figure 4D and E). IR25a or IR76b requirement for cellular fatty acid responses was confirmed by transgene rescue experiments, which restored Ca2+ increases to the level observed in w1118 controls flies (Figure 4D and E).
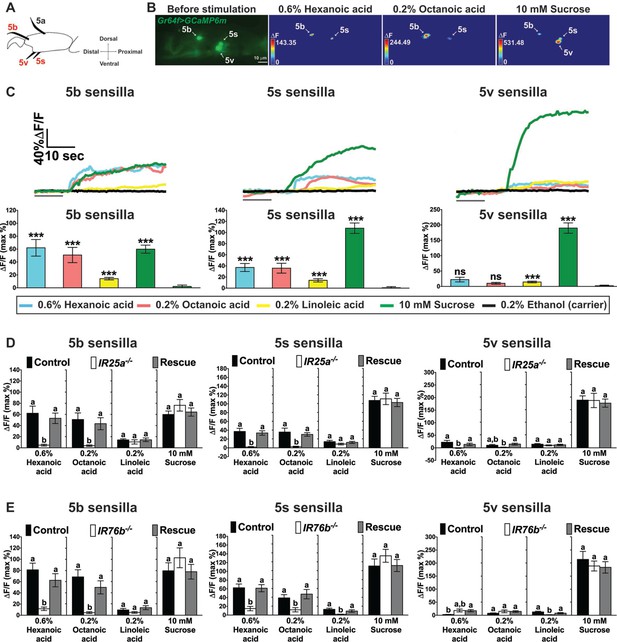
Cellular responses to fatty acids in sweet GRNs require IR25a and IR76b functions.
(A) Diagram of the fifth tarsal segment of the prothoracic leg. Ca2+ imaging was carried out for the sweet GRN of the 5b, 5s and 5v sensilla (the Gr64f-GAL4 expressing GRN in the 5a sensillum does not respond to sugar and was not included in our analysis). (B) Representative still images of the fifth tarsal segment of the prothoracic leg show maximum Ca2+ responses of the sweet GRNs upon stimulation by indicated ligands. ΔF indicates the changes in fluorescence light intensity of the cell body before/after ligand stimulation. (C) Representative fluorescence traces (top) and corresponding Ca2+ responses (bottom) of the sweet GRN associated with the 5b, 5s and 5v sensilla after stimulation with indicated ligands. The gray line underneath the fluorescence traces indicates time of ligand application. Hexanoic and octanoic acids elicit strong Ca2+ responses in the sweet GRN associated with 5b and 5s sensilla, but not the 5v sensillum. The 5v-associated sweet GRN shows weak, but significant Ca2+ responses to linoleic acid when compared to carrier. 10 mM sucrose was used as a positive control. Each bar represents the mean ± SEM of Ca2+ imaging with 13–57 female prothoracic legs. Two-tailed, Mann-Whitney U test versus 0.2% ethanol (carrier), ***p<0.001, ns: not significant. Fly genotype is Gr64f-GAL4/+; UAS-GCaMP6m/+. Note that traces and graphs for a second UAS-GCaMP6m reporter (located on the second chromosome and used to analyze IR76b mutant flies) are shown in Figure 4—figure supplement 1A and B. (D and E) IR25a and IR76b are necessary in sweet GRNs for fatty acid responses. Ca2+ responses of 5b-, 5s- and 5v-associated sweet GRNs of IR25a (D) or IR76b (E) mutant flies to indicated ligands. Ca2+ responses to hexanoic and octanoic acids are abolished in 5b- and 5s-associated sweet GRNs of both IR25a and IR76b mutant flies, and they are fully rescued when UAS-IR25a or UAS-IR76b expression is provided in sweet GRNs. Ca2+ responses to linoleic acid are significantly reduced only in the sweet GRN associated with 5s and 5v sensilla from IR76b mutant flies (E). Each bar represents the mean ± SEM of Ca2+ imaging with 16–50 female prothoracic legs. Bars with different letters are significantly different (Kruskal-Wallis test by ranks with Dunn’s multiple comparison tests, p<0.05). Each y-axis delineates groups for Kruskal-Wallis test. Fly genotypes D: Gr64f-GAL4/+; UAS-GCaMP6m/+ (Control, black bar), IR25a2 Gr64f-GAL4/IR25a2; UAS-GCaMP6m/+ (IR25a-/-, white) and IR25a2 Gr64f-GAL4/IR25a2 UAS-IR25a; UAS-GCaMP6m/+ (Rescue, grey); Fly Genotypes E: Gr64f-GAL4 UAS-GCaMP6m/+ (Control, black), Gr64f-GAL4 UAS-GCaMP6m/+; IR76b2/IR76b2 (IR76b-/-, white) and Gr64f-GAL4 UAS-GCaMP6m/UAS-IR76b; IR76b2/IR76b2 (Rescue, grey). For representative traces of these genotypes, see Figure 4—figure supplement 1C and D. Source data for summary graphs are provided in Figure 4—source data 1.
-
Figure 4—source data 1
Ca2+ imaging results of sweet GRNs of w1118, IR25a and IR76b mutant flies to fatty acids.
(C) Ca2+ responses of sweet GRNs associated with 5b, 5s or 5v sensilla to fatty acids. (D) Ca2+ responses of sweet GRNs associated with 5b, 5s or 5v sensilla of IR25a mutant flies to fatty acids. (E) Ca2+ responses of sweet GRNs associated with 5b, 5s or 5v sensilla of IR76b mutant flies to fatty acids.
- https://doi.org/10.7554/eLife.30115.015
The IR56d gene is sweet GRN specific and required for fatty acid taste
IR25a and IR76b are expressed in multiple GRNs per sensillum (Figure 2), including a recently characterized sour GRN where they are required to sense carboxylic acids and HCl (Chen and Amrein, 2017). This finding, together with the observation that IRs are likely to form tetrameric complexes containing three and possibly four different IR subunits (Abuin et al., 2011) led us to search for additional IR genes involved in fatty acid taste. We therefore examined seven candidate IR genes based on their expression in taste sensilla (Cameron et al., 2010; Koh et al., 2014) for effects on PER when functionally perturbed. RNAi knock-down of one of these genes, IR56d, led to a significant reduction in PER responses to all fatty acids (Figure 5A), while knock-downs of or of mutations in the other six IR genes had no effect (Figure 5—figure supplement 1). We next expressed GCaMP6m in sweet GRNs, along with the RNAiIR56d construct and carried out Ca2+ imaging experiments of sweet GRNs. As expected, we observed an almost complete loss of GRN activation to hexanoic and octanoic acids (Figure 5B and C), similar as observed for IR25a or IR76b mutants (Figure 4D and E). Finally, we examined expression of an IR56d-GAL4 line in the tarsal segments of the prothoracic leg (Koh et al., 2014). Indeed, antibody staining of tarsi of flies also expressing a marker for sweet GRNs showed complete overlap between IR56d and Gr64f, while no expression was observed in bitter GRNs, labeled by Gr66a-LexA (Figure 5E and F). Taken together, these experiments imply that the fatty acid taste receptor in sweet GRNs is composed of at least three subunits, IR25a, IR76b and the more narrowly expressed IR56d subunit. However, they do not exclude the possibility of yet a fourth IR being part of a fatty acid taste receptor complex.
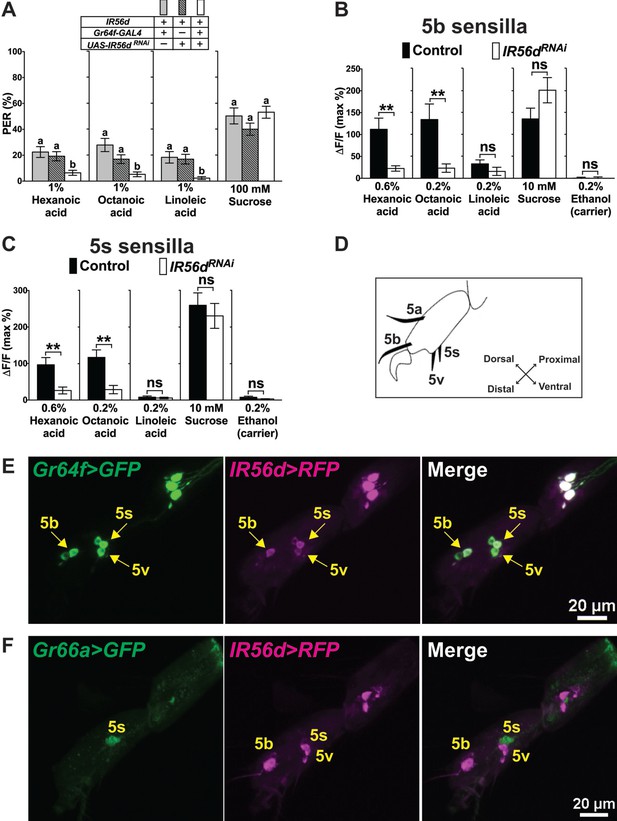
IR56d is necessary for fatty acid taste.
(A) PER responses are reduced to all three fatty acids, but not to sucrose, in flies expressing an UAS-RNAiIR56d construct in sweet GRNs. Targeted knockdown of IR56d is conducted by expression of UAS-IR56dRNAi under control of Gr64f-GAL4. Each bar represents the mean ± SEM of PER responses (n = 49–59 flies). Bars with different letters are significantly different (Kruskal-Wallis test by ranks with Dunn’s multiple comparison tests, p<0.05). Each y-axis delineates groups for Kruskal-Wallis test. Fly genotypes: control flies: Gr64f-GAL4/+ (grey), UAS-IR56dRNAi/+ (lines) and IR56d knock-down fly: Gr64f-GAL4/+; UAS-IR56dRNAi/+ (white). (B and C) Ca2+ responses in 5b- (B) and 5s- (C) associated sweet GRNs of flies expressing UAS-GCaMP6m and UAS-RNAiIR56d in sweet GRNs show loss of fatty acid induced neural activation, compared to neurons of control flies. Targeted knockdown of IR56d in sweet GRNs has no effect on Ca2+ responses to linoleic acid. 10 mM sucrose was used as a positive control. Each bar represents the mean ± SEM of Ca2+ imaging with 3–21 female prothoracic legs. Asterisks indicate a significant difference between the IR56dRNAi and control flies (Two-tailed, Mann-Whitney U test, **p<0.01, ns: not significant). Each y-axis delineates groups for Mann-Whitney U test. Fly genotypes: Gr64f-GAL4 UAS-GCaMP6m/+ (control, black) and Gr64f-GAL4 UAS-GCaMP6m/+; UAS-IR56dRNAi/+ (IR56dRNAi, white). (D) Drawing of the fifth tarsal segment of the prothoracic leg that is used for immunofluorescence experiments (E and F). Tarsal taste sensilla are indicated. (E and F) Expression of IR56d-GAL4 is restricted to a single GRN in all sensilla examined and co-localizes with Gr64f-LexA (E). No expression of IR56d-GAL4 is observed in Gr66a-LexA expressing bitter GRNs associated with 5s sensilla (F). Immunostaining with anti-GFP (green) and anti-mCD8 (magenta) antibodies on whole-mount preparations of tarsal segments of the prothoracic leg from flies of the genotypes: UAS-mCD8:RFP lexAop-rCD2:GFP;IR56d-GAL4/+;Gr64fLexA/TM6c (E) and UAS-mCD8:RFP lexAop-rCD2:GFP;Gr66a-LexA/IR56d-GAL4;+/TM6c (F). Arrows refer to a GRN of a sensillum expressing both Gr64f and IR56d genes. Source data for summary graphs are provided in Figure 5—source data 1.
-
Figure 5—source data 1
PER and Ca2+ responses of IR56d knock-down flies to fatty acids.
(A) PER responses of flies with knockdown of IR56d in sweet GRNs to fatty acids. (B and C) Ca2+ responses of the sweet GRNs associated with 5b (B) or 5s (C) sensilla to fatty acids after knockdown of IR56d in sweet GRNs.
- https://doi.org/10.7554/eLife.30115.019
Bitter GRNs modulate acceptance behavior of hexanoic acid
Because IR25a and IR76b are also found in bitter GRNs, we carried out Ca2+ imaging experiments using Gr33aGAL4, a bitter GRN specific marker (Moon et al., 2009). Bitter GRNs of both the s- and b-type sensilla strongly responded to denatonium, a bitter tasting compound. Intriguingly, strong Ca2+ increases were also observed in the 5b-associated bitter GRN to hexanoic acid, while octanoic or linoleic acid elicited no significant responses (Figure 6A and B; note that octanoic and linoleic acids could only be tested at 0.2%, due to low solubility; see Materials and methods). These observations indicate that at least hexanoic acid activates both appetitive and repulsive GRNs. However, and in contrast to sweet GRNs, bitter GRN responses to hexanoic acid did not require IR25a or IR76b (Figure 6C and D), indicating that these neurons employ a different molecular receptor.
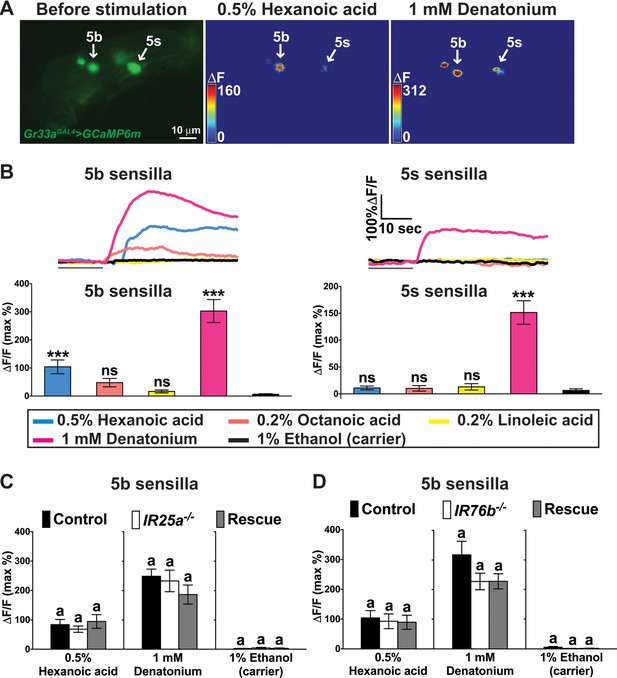
A subset of bitter GRNs responds to hexanoic acids in an IR25a/IR76b independent manner.
(A) Representative still images of the fifth tarsal segment of the prothoracic leg show maximum Ca2+ responses in the bitter GRNs associated with 5b and 5 s sensilla upon stimulation by indicated ligands. ΔF indicates the changes in fluorescence light intensity of the cell body before/after ligand application. (B) Representative fluorescence traces (top) and corresponding Ca2+ responses (bottom) of the 5b- and 5s-associated bitter GRNs upon stimulation by indicated ligands. The gray line underneath the fluorescence traces indicates time of ligand application. Hexanoic acid elicits highly significant Ca2+ responses in the 5b- associated bitter GRNs (compared to carrier). 1 mM denatonium was used as a positive ligand control. 1% ethanol was used as a carrier to facilitate solubilization of high concentrations of hexanoic acid (2.5%). Each bar represents the mean ± SEM of Ca2+ imaging with 10–30 female prothoracic legs. Two-tailed, Mann-Whitney U test versus carrier (1% ethanol), ***p<0.001, ns: not significant. Fly genotype is Gr33aGAL4 UAS-GCaMP6m/+. (C and D) Cellular responses to fatty acid in bitter GRNs do not require IR25a and IR76b. Ca2+ responses of the 5b-assoicated bitter GRNs of IR25a (C) or IR76b (D) mutant flies to indicated ligands. Ca2+ responses of GRNs of mutants to hexanoic acid were not significantly reduced when compared to control flies. Each bar represents the mean ± SEM of Ca2+ imaging with 4–42 female prothoracic legs. Bars with different letters are significantly different (Kruskal-Wallis test by ranks with Dunn’s multiple comparison tests, p<0.05). Each y-axis delineates groups for Kruskal-Wallis test. Fly genotypes C: Gr33aGAL4/+; UAS-GCaMP6m/+ (Control, black), IR25a2 Gr33aGAL4/IR25a2; UAS-GCaMP6m/+ (IR25a-/-, white), and IR25a2 Gr33aGAL4/IR25a2 UAS-IR25a; UAS-GCaMP6m/+ (Rescue, grey); Fly Genotypes D: Gr33aGAL4 UAS-GCaMP6m/+ (Control, black), Gr33aGAL4 UAS-GCaMP6m/+; IR76b2/IR76b2 (Ir76b-/-, white) and Gr33aGAL4 UAS-GCaMP6m/UAS-IR76b; IR76b2/IR76b2 (Rescue, grey). See Figure 6—figure supplement 1—source data 1 for hexanoic acid responses of 5s-associated bitter GRNs from IR25a or IR76b mutant flies. Source data for summary graphs are provided in Figure 6—source data 1.
-
Figure 6—source data 1
Ca2+ responses of bitter GRNs of IR25a or IR76b mutant flies to fatty acids.
(B) Ca2+ responses of bitter GRNs associated with 5b or 5s sensilla to fatty acids. (C) Ca2+ responses of bitter GRNs associated with 5b sensilla of IR25a mutant flies to hexanoic acid. (D) Ca2+ responses of bitter GRNs associated with 5b sensilla of IR76b mutant flies to hexanoic acid.
- https://doi.org/10.7554/eLife.30115.023
To investigate hexanoic acid response characteristics in more detail, we compared dose response profiles of sweet and bitter GRNs of 5b sensilla (Figure 7A). Interestingly, sweet GRNs reached maximal activation already at the modest concentration of 1% (Figure 7A, left). In contrast, maximal activation of bitter GRNs was reached at the highest applicable concentration (2.5%; Figure 7A, right). These observations suggest that bitter GRNs might counteract the activity of sweet GRNs above a certain concentration threshold (>1%) and possibly modulate taste behavior. Indeed, w1118 flies exhibited a maximal PER response at ~1%, whereas higher concentrations of hexanoic acid led to a decrease in PER responses (Figure 7B). In contrast, when we inhibited the activity of bitter GRNs by either expressing TNT or the inward-rectifier potassium channel Kir2.1, PER responses stayed at the same high level or continued to increase with increasing hexanoic acid concentration (Figure 7C and Figure 7—figure supplement 2). Thus, activation of bitter GRNs appears to suppress PER responses at high (2.5%) hexanoic acid concentration. To test whether the decrease of sweet GRN responses was mediated through lateral inhibition, we compared Ca2+ responses of sweet GRNs to 2.5% hexanoic acid in the presence and absence of functional bitter GRNs. However, hexanoic acid elicited the same Ca2+ responses in sweet GRNs, regardless of whether functional bitter GRNs were present (Figure 7D).
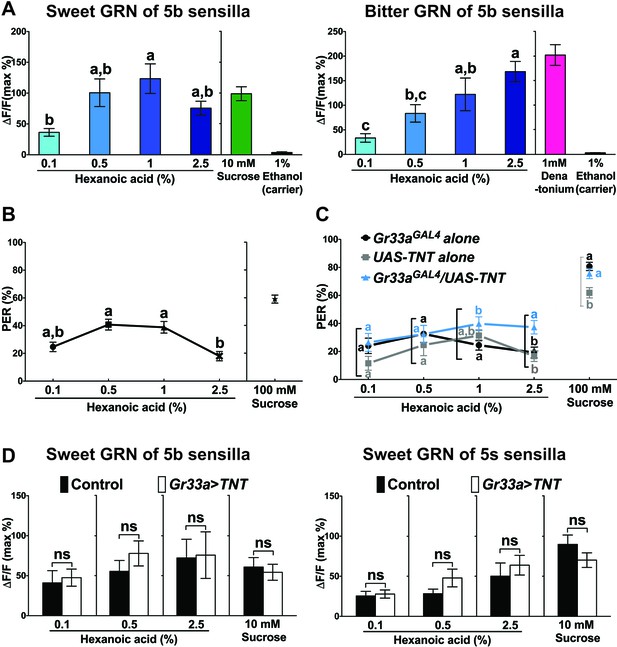
Bitter GRNs inhibit acceptance of high concentration of hexanoic acid.
(A) Hexanoic acid dose response profiles of the sweet (left) and the bitter (right) GRNs associated with the 5b sensilla. sweet GRN reaches a maximal response already at 1%, while bitter GRNs responses further increases as ligand concentration increases. Each bar represents the mean ± SEM of Ca2+ imaging with 7–30 female prothoracic legs. Bars with different letters are significantly different (Kruskal-Wallis test by ranks with Dunn’s multiple comparison tests, p<0.05). Each y-axis delineates groups for Kruskal-Wallis test. Fly genotypes: Gr64f-GAL4 UAS-GCaMP6m/+ and Gr33aGAL4/+;UAS-GCaMP6m/+. For hexanoic acid response profiles of the sweet GRNs, or the bitter GRN associated with 5 s/5v sensilla, see Figure 7—figure supplement 1. (B) PER responses of wild-type flies (w1118) to different concentrations of hexanoic acid. At high concentration (2.5%) hexanoic acids induces a much lower PER response compared to more modest concentrations (0.5%–1%). Each symbol represents the mean ± SEM of PER responses (n = 61–142 flies). Symbols with different letters are significantly different (Kruskal-Wallis test by ranks with Dunn’s multiple comparison tests, p<0.01). Each y-axis delineates groups for Kruskal-Wallis test. (C) Inactivation of bitter GRN leads to concentration dependent PER responses to hexanoic acid, while control flies show a response profile similar to w1118 flies (see B). Each symbol represents the mean ± SEM of PER responses (n = 23–121 flies). Symbols with different letters are significantly different (Kruskal-Wallis test by ranks with Dunn’s multiple comparison tests, p<0.05). Each square bracket delineates groups for Kruskal-Wallis test. Fly genotypes: Gr33aGAL4/+ (Gr33aGAL4 alone), UAS-TNT/+ (UAS-TNT alone) and Gr33aGAL4/UAS-TNT. (D) Inactivation of bitter GRNs has no effect on cellular responses of sweet GRNs to hexanoic acid. Ca2+ responses of sweet GRNs to 2.5% hexanoic acid are similar regardless of whether a functional bitter GRN is present. Bitter GRNs was inactivated by expressing UAS-TNT under the control of Gr33aGAL4. Each bar represents the mean ± SEM of 10–15 female GRNs from prothoracic legs. Two-tailed, Mann-Whitney U test between the flies lacking functional bitter GRNs and control flies, p<0.05, ns: not significant. Each y-axis delineates groups for Mann-Whitney U test. Fly genotypes: Gr64fLexA/lexAop-GCaMP6m (control) and Gr33aGAL4/UAS-TNT; Gr64fLexA/lexAop-GCaMP6m (Gr33a > TNT). Source data for summary graphs are provided in Figure 7—source data 1.
-
Figure 7—source data 1
Dosage dependent Ca2+ and PER responses of neurons and flies to hexanoic acid.
(A) Ca2+ responses of the sweet or the bitter GRNs associated with 5b sensilla to different dosages of hexanoic acid. (B) PER responses of wild-type flies (w1118) to different dosages of hexanoic acid. (C) PER responses of flies to different dosages of hexanoic acid when bitter GRNs are inactivated by expression of UAS-TNT. (D) Ca2+ responses of the sweet GRNs associated with 5b or 5s sensilla to different dosages of hexanoic acid when bitter GRNs are inactivated by expression of UAS-TNT.
- https://doi.org/10.7554/eLife.30115.029
Discussion
IR genes have emerged as a second large gene family encoding chemoreceptors in insects. In the Drosophila olfactory system, IRs function as multimeric receptors in coeloconic olfactory sensory neurons (OSN) and are thought to sense volatile carboxylic acids, amines and aldehydes (Abuin et al., 2011; Benton et al., 2009; Hussain et al., 2016; Silbering et al., 2011). Expression analyses have shown that each coeloconic OSN expresses up to four IR genes, including high levels of either IR8a or IR25a (Abuin et al., 2011). IR25a and IR8a are distinct from other IRs in that they are more conserved to each other and iGluRs, and they share a long amino terminal domain absent in all other IRs (Croset et al., 2010). These observations, along with functional analyses of basiconic olfactory neurons that express combinations of IR genes, led to a model in which IR based olfactory receptors are tetrameric complexes thought to consist of up to three different subunits that contain at least one core unit (IR8a or IR25a) and two additional IRs that determine ligand binding specificity (Abuin et al., 2011; Silbering et al., 2011). The findings presented in this paper expand this concept to taste receptors that sense fatty acids through the sweet GRNs found in tarsal taste sensilla.
IR25a and IR76b mediate fatty acid taste in sweet GRNs
Our analysis extends the multimodal role of IR25a and IR76b to the taste systems. Consistent with gene expression arrays (Cameron et al., 2010), we showed in this paper that up to three GRNs, including many sweet and bitter GRNs, co-express IR25a and IR76b. Our functional studies have established a novel role for these two IR proteins in fatty acid taste, which revealed that these two subunits are not only critically important to elicit PER responses in flies when challenged with fatty acids, but are also necessary for fatty acid induced Ca2+ increases in tarsal sweet GRNs. Based on these findings and with consideration of their established role in other sensory systems, we propose that IR25a and IR76b play central roles in sweet GRNs in a multimeric receptor complex for initiating appetitive taste behavior to these chemicals. Intriguingly, both genes are also co-expressed in two other GRNs of most tarsal taste sensilla, strongly arguing for additional taste functions. While the subset of tarsal bitter GRNs activated by hexanoic acid does not require either gene, we have discovered that the third GRN (the sour GRN) is narrowly tuned to acids in an IR25a/IR76b dependent manner (Chen and Amrein, 2017). These observations suggest that modality specific IRs are likely expressed in a cell-type specific fashion whereby they complement IR25a/IR76b to function as either a fatty acid or a sour taste receptor. Indeed, our screen identified IR56d, a gene that is expressed in sweet GRNs of tarsal taste sensilla, as a likely candidate encoding an IR subunit specific for a fatty acid taste receptor (Figure 5). It remains to be seen whether IR25a, IR76b and IR56d comprise all subunits that constitute this receptor or whether yet additional IRs are necessary to mediate responses to these chemicals.
The fact that different food chemicals can activate a single class of neurons raises the question how flies discriminate between sugars and fatty acids. First, we note the difference in sensitivity of appetitive GRNs to sugars and fatty acids, respectively: The most responsive GRN for sugars is the one associated with the 5v sensilla, followed by that with the 5s and finally the 5b sensilla, while the responsiveness for fatty acids is the reverse (5b > 5s > 5v; Figure 4). Second, fatty acids induces weaker PER responses from stimulation of the labial palps as opposed to tarsi, while sugars induce equally strong PER responses from stimulation of either taste organ (Figure 1—figure supplement 1) (Fujii et al., 2015). Third, at least some fatty acids activate bitter GRNs (Figure 6), and hence, generate more complex activation patterns in the brain than sugars, which are not known to activate neurons other than sweet GRNs. These properties may provide a rationale for differential coding of these two classes of chemicals in the brain. Finally, sugars but not fatty acids are soluble in water, and hence, the specific solvents in which these chemicals are presented provides different textural quality, which was recently shown to play a role in taste perception (Zhang et al., 2016).
NorpA, which encodes a phospholipase C (PLC), plays a critical role in sweet GRNs for appetitive feeding responses to fatty acids, but it is dispensable for behavioral responses to sugars (Masek and Keene, 2013). We found that its absence also selectively abolishes Ca2+ responses to fatty acids, but not sugars, in sweet cells (Figure 1—figure supplement 2). NorpA is known for its role as downstream effector of G-protein coupled receptors in the fly’s visual system (Bloomquist et al., 1988; Wilson and Ostroy, 1987), but interestingly it is also required for olfactory responses in neurons of the maxillary palps (Riesgo-Escovar et al., 1995), which express ORs that are thought to function as ligand-gated ion channels (Sato et al., 2008; Wicher et al., 2008). We note that fatty acid taste in mammals is in part mediated by two G – protein coupled receptors, GPR40 and GPR120 (Cartoni et al., 2010; Matsumura et al., 2007), and that one of these (GPR120) was found to signal through a phospholipase C (Reed and Xia, 2015). Thus, future studies will be necessary to gain insights for how PLC mediates chemosensory responses through ORs and the phylogenetically unrelated IRs.
IRs form multimodal receptors that mediate diverse sensory cues
Mutlimeric IR based receptors were recently shown to be required in non-chemosensory processes. Specifically, Dorsal Organ Cool Cells (DOCCs) located in the larval brain, express and require the function of three IRs (IR21a IR25a and IR93a), thereby allowing larvae to avoid temperatures below ~20°C (Knecht et al., 2016; Ni et al., 2016). Similarly, two sets of cells in the antennal sacculus of adult flies, requiring the functions of IR25a and IR93a and either IR40a or IR68a, were shown to mediate a fly’s preferred humidity environment, which is generally in the dry range, but is also dependent on the fly’s hydration state (Enjin et al., 2016; Knecht et al., 2017; Knecht et al., 2016). Intriguingly, these non-chemosensory IR complexes share a common theme with the fatty acid and carboxylic acid taste receptors (Chen and Amrein, 2017) in that they all require a core unit (IR25a) and two additional IRs that mediate specificity for a particular stimulus type (i.e. temperature, humidity, fat, acid).
An IR76b based sodium channel and an IR76b based amino acid receptor appear to lack an obligate core unit (IR25a or IR8a) found in olfactory receptors or fatty acid and carboxylic acid taste receptors. The IR76b sodium channel mediates salt responses in a heterologous systems independently of any other IRs (Zhang et al., 2013), while a proposed multimeric IR76b containing receptor mediates amino acids taste in wild type and IR25a mutant flies (IR8a is not expressed in taste neurons) (Ganguly et al., 2017). It will be interesting to elucidate the compositions of complete IR based amino acid and sour taste receptors, and – with regard of amino acid receptors – to identify the neurons that mediate this taste modality.
Materials and methods
Reagent type | Designation | Source or reference | Identifiers |
---|---|---|---|
Antibodies | |||
Chicken polyclonal anti-GFP | Anti-GFP | Thermo Fisher Scientific | PA1-86341; RRID: AB_931091 |
Rat monoclonal anti-mCD8 | Anti-mCD8 | Thermo Fisher Scientific | MCD0800; RRID: AB_10392843 |
Rabbit polyclonal anti-IR25a | Anti-IR25a | Benton et al. (2009) | N/A |
Mouse monoclonal anti-HA | Anti-HA | Covance Research Product Inc. | MMS-101P; RRID: AB_2314672 |
Alexa 488 conjugated goat anti-chicken | Green | Thermo Fisher Scientific | A11039; RRID: AB_2534096 |
Cy3-conjugated goat anti-rat | Magenta | Jackson Immunoresearch Laboratories Inc. | 112-165-072; RRID: AB_2338248 |
Cy3-conjugated goat anti-rabbit | Magenta | Jackson Immunoresearch Laboratories Inc. | 111-166-003; RRID: AB_2338000 |
Cy3-conjugated goat anti-mouse | Magenta | Jackson Immunoresearch Laboratories Inc. | 115-166-072; RRID: AB_2338706 |
Chemical compounds | |||
Hexanoic acid | Hexanoic acid | Sigma-Aldrich | H12137 |
Octanoic acid | Octanoic acid | Sigma-Aldrich | O3907 |
Linoleic acid | Linoleic acid | Sigma-Aldrich | L1376 |
Denatonium benzoate | Denatonium | Sigma-Aldrich | D5765 |
Sucrose | Sucrose | Amresco | M1117 |
Experimental Models: Organisms/Strains | |||
D. melanogaster: w1118 | Wild-type control | Bloomington Drosophila Stock Center | BDSC: 3605; FlyBase: FBst0003605 |
D. melanogaster: w*; P{Gr64f-GAL4.9.7}5/CyO; MKRS/TM2 | Gr64f-GAL4 | Dahanukar et al. (2007) | Flybase: FBst0057669 |
D. melanogaster: w*; TI{LexA::VP16}Gr64fLexA | Gr64fLexA | Fujii et al. (2015) | Flybase: FBti0168176 |
D. melanogaster: w*; TI{GAL4}Gr33aGAL4 | Gr33aGAL4 | Moon et al. (2009) | Flybase: FBst0031425 |
D. melanogaster: w*; P{UAS-TeTxLC.tnt}G2 | UAS-TNT | Sweeney et al. (1995) | Flybase: FBst0028838 |
D. melanogaster: w*; P{lexAop-rCD2-GFP} | lexAop-rCD2:GFP | Lai and Lee (2006) | Flybase: FBst0066687 |
D. melanogaster: w*;P{10XUAS-IVS-mCD8::RFP}attP40 | UAS-mCD8:RFP | Bloomington Drosophila Stock Center | BDSC: 32219; Flybase: FBti0131967 |
D. melanogaster: w1118; PBac{20XUAS-IVS-GCaMP6m}VK00005 | UAS-GCaMP6m | Bloomington Drosophila Stock Center | BDSC: 42750; Flybase: FBst0042750 |
D. melanogaster: y1 w*; P{UAS-mCD8::GFP.L}LL5,P{UAS-mCD8::GFP.L}2 | UAS-mCD8:GFP | Bloomington Drosophila Stock Center | BDSC: 5137; Flybase: FBst0005137 |
D. melanogaster: y1 w1118; P{QUAS-mtdTomato-3xHA}26 | QUAS-mtd-Tomato-3xHA | Bloomington Drosophila Stock Center | BDSC: 30005; Flybase: FBst0030005 |
D. melanogaster: y1 sc* v1; P{TRiP.HMC03664}attP40 | UAS-IR94h-RNAi | Bloomington Drosophila Stock Center | BDSC: 53675; Flybase: FBst0053675 |
D. melanogaster: w1118; Mi{ET1}IR52cMB04402 | Mi{ET1}IR52cMB04402 | Bloomington Drosophila Stock Center | BDSC: 24580; Flybase: FBst0024580 |
D. melanogaster: w1118; Mi{ET1}IR56bMB09950 | Mi{ET1}IR56bMB09950 | Bloomington Drosophila Stock Center | BDSC: 27818; Flybase: FBst0027818 |
D. melanogaster: y1 w*;Mi{MIC}IR62aMI00895 lml1MI00895/TM3, Sb1, Ser1 | Mi{Mic}IR62aMI00895 | Bloomington Drosophila Stock Center | BDSC: 32713; Flybase: FBst0032713 |
D. melanogaster: w1118;P{UAS-norpA.WT}2 | UAS-norpA | Bloomington Drosophila Stock Center | BDSC: 35529; Flybase: FBst0035529 |
D. melanogaster: w*; TI{TI}IR25a1/CyO | IR25a1/CyO | Benton et al. (2009) | Flybase: FBst0041737 |
D. melanogaster: w*; P{IR25a-GAL4.A}236.1; TM2/TM6B, Tb1 | IR25a-GAL4 | Abuin et al. (2011) | Flybase: FBst0041728 |
D. melanogaster: w*; M{UAS-IR25a.attB} | UAS-IR25a | Abuin et al. (2011) | Flybase: FBal0249355 |
D. melanogaster: {KK104276}VIE-260B | UAS-IR11a-RNAi | Vienna Drosophila Resource Center | VDRC ID: 100422; Flybase: FBgn0030385 |
D. melanogaster: w1118; P{GD773}v2472 | UAS-IR21a-RNAi | Vienna Drosophila Resource Center | VDRC ID: 2472; Flybase: FBgn0031209 |
D. melanogaster: w1118; P{GD2094}v4704 | UAS-IR56b-RNAi | Vienna Drosophila Resource Center | VDRC ID: 4704; Flybase: FBgn0034456 |
D. melanogaster: w1118; P{GD2096}v6112 | UAS-IR56d-RNAi | Vienna Drosophila Resource Center | VDRC ID: 6112; Flybase: FBgn0034458 |
D. melanogaster: w*; IR76b1 | IR76b1/IR76b1 | Zhang et al. (2013) | Flybase: FBst0051309 |
D. melanogaster: w*; IR76b2 | IR76b2/IR76b2 | Zhang et al. (2013) | Flybase: FBst0051310 |
D. melanogaster: w*; P{IR76b-GAL4.1.5}2 | IR76b-GAL4, | Zhang et al. (2013) | Flybase: FBst0051311 |
D. melanogaster: w*; P{UAS-IR76b.Z}2/CyO; TM2/TM6B, Tb1 | UAS-IR76b | Zhang et al. (2013) | Flybase: FBst0052610 |
D. melanogaster: w*; P{IR76b-QF.1.5} | IR76b-QF | Zhang et al. (2013) | Flybase: FBtp0085487 |
D. melanogaster: w*; P{UAS-Hsap\KCNJ2.EGFP}1 | UAS-Kir2.1-GFP | Baines et al. (2001); Paradis et al. (2001) | Flybase: FBst0006596 |
D. melanogaster: w*; norpA36(P24) | norpAP24 | Masek and Keene (2013) | Flybase: FBst0009048 |
D. melanogaster: w*; P{IR56d-GAL4.K}7–2/CyO; P{UAS-mCD8::GFP.L}LL6 | IR56d-GAL4 | Koh et al. (2014) | Flybase: FBst0060708 |
D. melanogaster: w*; Gr66a-LexA/CyO; TM2/TM6B | Gr66a-LexA | Thistle et al. (2012) | Flybase: FBal0277069 |
Software and Algorithms | |||
NIS-Elements | N/A | Nikon | N/A |
Prism software | Prism software | GraphPad Software 5.0 Inc | N/A |
Other | |||
Nikon Eclipse Ti inverted microscope | Nikon Eclipse Ti inverted microscope | Nikon | N/A |
Nikon A1 confocal microscope | Nikon A1R confocal microscope system | Nikon | N/A |
35 mM Glass bottom dish | Glass bottom culture dish | MatTek Corporation | P35G-0–10 C |
Drosophila stocks
Request a detailed protocolFlies were maintained on standard corn meal food in plastic vials under a 12 hr light/dark cycle at 25° C. The w1118 strain was used as a wild-type control. Fly lines used: Gr64f-Gal4 (Dahanukar et al., 2007); Gr64fLexA (Fujii et al., 2015); Gr33aGAL4 (Moon et al., 2009); UAS-TNT (Sweeney et al., 1995); lexAop-rCD2:GFP (Lai and Lee, 2006); UAS-mCD8:RFP, UAS-GCaMP6m, UAS-mCD8:GFP, QUAS-mtd-Tomato-3xHA, UAS-IR94h-RNAi, Mi{ET1}IR52cMB04402, Mi{ET1}IR56bMB09950, Mi{Mic}IR62aMI00895 and UAS-norpA (Bloomington Drosophila Stock Center, numbers 32219, 42750, 5137, 30005, 53675, 24580, 27818, 32713 and 35529); IR25a1 (Benton et al., 2009); IR25a-GAL4, UAS-IR25a (Abuin et al., 2011); UAS-IR11a-RNAi, UAS-IR21a-RNAi, UAS-IR56b-RNAi and UAS-IR56d-RNAi (Vienna Drosophila Resource Center, transformant ID 100422, 2472, 4704 and 6112); IR76b1, IR76b2, IR76b-GAL4, UAS-IR76b, IR76b-QF (Zhang et al., 2013); UAS-Kir2.1-GFP (Baines et al., 2001; Paradis et al., 2001); norpAP24 (Masek and Keene, 2013); IR56d-GAL4 (Koh et al., 2014) and Gr66a-LexA (Thistle et al., 2012).
Chemicals
Hexanoic (Cat No. H12137), octanoic (Cat No. O3907) and linoleic acids (Cat No. L1376), and denatonium benzoate (Cat No. D5765) were purchased from Sigma-Aldrich(St. Louis, MO) with a purity of >98%. Sucrose (Cat No. M1117) was purchased from Amresco (Solon, OH). Stock solutions of fatty acids were prepared in 80% ethanol, except for hexanoic acid (in 20% ethanol) and kept at −20° C. A stock solution for denatonium benzoate was prepared in Millipore Q water and kept at 4° C for up to one week. Stock solutions were diluted to the final concentration using Millipore Q water prior to each experiment. Sucrose and denatonium benzoate solutions were also mixed with ethanol to obtain the same final concentration of ethanol.
Proboscis extension reflex (PER) assay
Request a detailed protocolPER assays were carried out as described in Slone et al. (Slone et al., 2007) with some modifications. Briefly, files were collected on the day of eclosion and kept in standard corn meal food for 3–7 days at 25°C. Before performing PER assays, flies were starved for 24 to 26 hr at 25°C in vials with a water-saturated Whatman filter paper. Flies were immobilized by cooling on ice, mounted by their backs/wings on a microscope slide using double-sided Scotch tape and allowed to recover for 30 min at room temperature. Prior to the PER assay, flies were allowed to drink water until satiation to ensure PER responses were nutrient derived. Flies that showed no response to water were excluded. Taste solutions were delivered with a 10 μl pipette to legs for up to ~four s. Each fly was tested three times with each taste solutions, and flies were allowed to drink water between each new application. A PER response was recorded as positive (1) if the proboscis was fully extended, otherwise it was recorded as negative (0). PER response scores (%) from a single fly was 0% (0/3 responses in the three applications), 33% (1/3), 66% (2/3) or 100% (3/3).
Immunofluorescence
Request a detailed protocolFlies were aged for 4–7 days in standard corn meal food at 25°C before dissection. Labella were detached from the proboscis, while the tarsi were cut off above the fourth segment. Tissues were placed in eppendorf tubes containing fixation buffer (phosphate buffered saline with 4% paraformaldehyde and 0.2% triton X-100) for 30 min at room temperature. Tissues were washed twice in washing buffer (phosphate buffered saline containing 0.2% triton X-100) for 30 to 60 min at room temperature and incubated with the primary antibodies (chicken anti–GFP, 1:2000 dilution; rat anti–mCD8, 1:200 dilution, Thermo Fisher Scientific; rabbit anti-IR25a, 1:1000 dilution, (Benton et al., 2009); mouse anti-HA, 1:1000 dilution, Covance Research Product Inc.) at 4°C overnight in washing buffer containing 5% heat-inactivated goat serum. Tissues were washed twice in washing buffer for 30 to 60 min at room temperature and incubated with the secondary antibodies (goat anti–chicken ALEXA 488, 1:500 dilution, Thermo Fisher Scientific; goat anti–rat Cy3, 1:300 dilution; goat anti-rabbit Cy3, 1:300 dilution; goat anti-mouse Cy3, 1:100 dilution, Jackson Immunoresearch Laboratories Inc.) in washing buffer containing 5% heat-inactivated goat serum for 3 hr at room temperature. Tissues were washed twice in washing buffer for 30 to 60 min at room temperature. All incubations, except incubations with antibody, were performed under gentle mixing. Tissues were mounted with VectaShield (Vector Lab) on a microscope slide and images were obtained using a Nikon A1R confocal microscope system.
Calcium imaging
Request a detailed protocolCalcium imaging for tarsal taste neurons was performed based on the protocol described in Miyamoto et al. (Miyamoto et al., 2013, 2012) with minor modification. Briefly, female files were collected on the day of eclosion and kept in standard corn meal food for 3–8 days at 25°C. The prothoracic leg was cut with a razor blade between the femur and the tibia, and silicone lubricant (Dow Corning) was applied to seal the cut area of the leg. Legs were mounted laterally on a glass bottom culture dish (MatTek Corporation) using double-sided scotch tape and covered with 1% agarose, leaving the fourth and fifth tarsal segments exposed for ligand application. Millipore Q water (100 µl) was applied to cover the preparation, which was placed on the stage of a Nikon eclipse Ti inverted microscope. Images were obtained every 500 ms, starting 15 s before application and ending 105 s after ligand application. Three to five different ligands (100 μl) were tested in each preparation. Sucrose or denatonium ligands were included in each preparation to ensure functionality of sweet or bitter GRNs, respectively. The sequence of ligand application was sucrose (or denatonium) solution, carrier (0.2 or 1% ethanol in water) and fatty acid solution. When fatty acids were tested, 0.2% ethanol was used as a carrier for all Ca2+ imaging experiments (1% ethanol when testing 2.5% hexanoic acid), because ethanol concentration above 1% destabilizes the preparation. Maximal concentration of octanoic and linoleic acids was 0.2% in 1% ethanol solution due to insolubility at higher concentrations. Preparations were washed with 200 μl of Millipore Q water five times after a ligand was tested, and equilibrated for 3 min before the next ligand was applied. Fluorescence light intensity of tarsal taste neurons was measured in the cell bodies. Background auto fluorescence, obtained from adjacent region, was subtracted. Baseline fluorescence was determined from the average of five frame measurements, taken immediately before ligand application. An equation for ∆F/F (%) was fluorescence light intensity of the cell body – baseline/baseline x 100. ∆F/F (max %) was calculated as the maximum value within 30 s after ligand application.
Statistical analysis
Request a detailed protocolStatistical analyses were conducted using Prism software (GraphPad Software 5.0 Inc.). PER assay and Ca2+ imaging data were analyzed with nonparametric statistics because tested groups did not meet the assumption for normal distribution based on D`Agostino-Perason and Shapiro-Wilk normality tests. For comparison between multiple groups (data of all figures, except Figures 1B, 4C, 5B, C, 6B, 7D and Figure 1—figure supplement 1, Figure 4—figure supplement 1B and Figure 5—figure supplement 1B), Kruskal-Wallis test by ranks (nonparametric one-way ANOVA) was performed to test for difference of rank distribution. As post hoc test, Dunn’s multiple comparison tests were employed to compare two specific groups. Mann-Whitney U test (nonparametric t test) with two-tailed P value was used to compare means of two groups (Figures 1B, 4C, 5B, C, 6B, 7D and Figure 1—figure supplement 1, Figure 4—figure supplement 1B and Figure 5—figure supplement 1B). Sample size for PER assays and Ca2+ imaging experiments were based on Slone et al. (Slone et al., 2007) and Miyamoto et al. (Miyamoto et al., 2012)
References
-
Altered electrical properties in Drosophila neurons developing without synaptic transmissionJournal of Neuroscience 21:1523–1531.
-
Taste preference for fatty acids is mediated by GPR40 and GPR120Journal of Neuroscience 30:8376–8382.https://doi.org/10.1523/JNEUROSCI.0496-10.2010
-
The neural code for taste in the brain stem: response profilesPhysiology & Behavior 69:87–96.https://doi.org/10.1016/S0031-9384(00)00191-8
-
Humidity sensing in DrosophilaCurrent Biology 26:1352–1358.https://doi.org/10.1016/j.cub.2016.03.049
-
G protein-coupled receptors in human fat taste perceptionChemical Senses 37:123–139.https://doi.org/10.1093/chemse/bjr069
-
Two families of candidate taste receptors in fishesMechanisms of Development 122:1310–1321.https://doi.org/10.1016/j.mod.2005.07.005
-
The G protein-coupled receptor subset of the chicken genomePLoS Computational Biology 2:e54.https://doi.org/10.1371/journal.pcbi.0020054
-
Genetic mosaic with dual binary transcriptional systems in DrosophilaNature Neuroscience 9:703–709.https://doi.org/10.1038/nn1681
-
The molecular and cellular basis of taste coding in the legs of DrosophilaJournal of Neuroscience 34:7148–7164.https://doi.org/10.1523/JNEUROSCI.0649-14.2014
-
GPR expression in the rat taste bud relating to fatty acid sensingBiomedical Research 28:49–55.https://doi.org/10.2220/biomedres.28.49
-
A candidate taste receptor gene near a sweet taste locusNature Neuroscience 4:492–498.https://doi.org/10.1038/87440
-
Recent advances in fatty acid perception and geneticsAdvances in Nutrition: An International Review Journal 6:353–360.https://doi.org/10.3945/an.114.007005
-
Sugar receptors in DrosophilaCurrent Biology 17:1809–1816.https://doi.org/10.1016/j.cub.2007.09.027
-
The representation of taste quality in the mammalian nervous systemBehavioral and Cognitive Neuroscience Reviews 4:143–191.https://doi.org/10.1177/1534582305280031
-
Taste preference for amino acids is dependent on internal nutritional state in Drosophila melanogasterJournal of Experimental Biology 215:2827–2832.https://doi.org/10.1242/jeb.069146
-
Molecular architecture of smell and taste in DrosophilaAnnual Review of Neuroscience 30:505–533.https://doi.org/10.1146/annurev.neuro.30.051606.094306
-
Studies of the Drosophila norpA phototransduction mutant. I. Electrophysiological changes and the offsetting effect of lightJournal of Comparative Physiology. A, Sensory, Neural, and Behavioral Physiology 161:785–791.https://doi.org/10.1007/BF00610220
-
Genomic and genetic evidence for the loss of umami taste in batsGenome Biology and Evolution 4:73–79.https://doi.org/10.1093/gbe/evr126
Article and author information
Author details
Funding
National Institutes of Health (RO1GMDC05606)
- Hubert O Amrein
National Institutes of Health (RO1DC13967)
- Hubert O Amrein
The funders had no role in study design, data collection and interpretation, or the decision to submit the work for publication.
Acknowledgements
We thank R Benton and C Montell for fly strains. This work was supported by grants 1RO1GMDC05606 and 1RO1DC13967 from National Institutes of Health.
Copyright
© 2017, Ahn et al.
This article is distributed under the terms of the Creative Commons Attribution License, which permits unrestricted use and redistribution provided that the original author and source are credited.
Metrics
-
- 4,471
- views
-
- 641
- downloads
-
- 117
- citations
Views, downloads and citations are aggregated across all versions of this paper published by eLife.
Citations by DOI
-
- 117
- citations for umbrella DOI https://doi.org/10.7554/eLife.30115