Activity-induced Ca2+ signaling in perisynaptic Schwann cells of the early postnatal mouse is mediated by P2Y1 receptors and regulates muscle fatigue
Figures
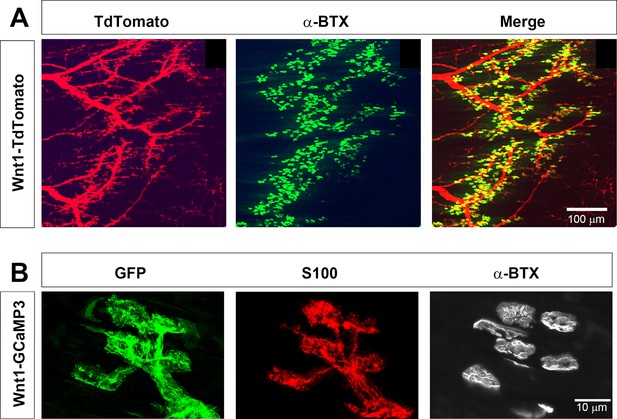
Wnt1-Cre drives expression of reporters and activity sensors to Schwann cells of the neonatal diaphragm.
(A) Whole mounts of P7 Wnt1-TdTomato diaphragm were labeled with 488-conjugated α-bungarotoxin (α-BTX). Myelinating Schwann cells along phrenic nerve branches as well as terminal/perisynaptic Schwann cells at α−BTX-labeled neuromuscular junctions (NMJs; green) exhibit tdTomato epifluorescence (red). (B) Higher magnification of whole mounts of P7 Wnt1-GCaMP3 diaphragm labeled with GFP, S100, and 633-conjugated α-BTX. All NMJ-associated, S100-immunostained TPSCs express GFP and thus GCaMP3.
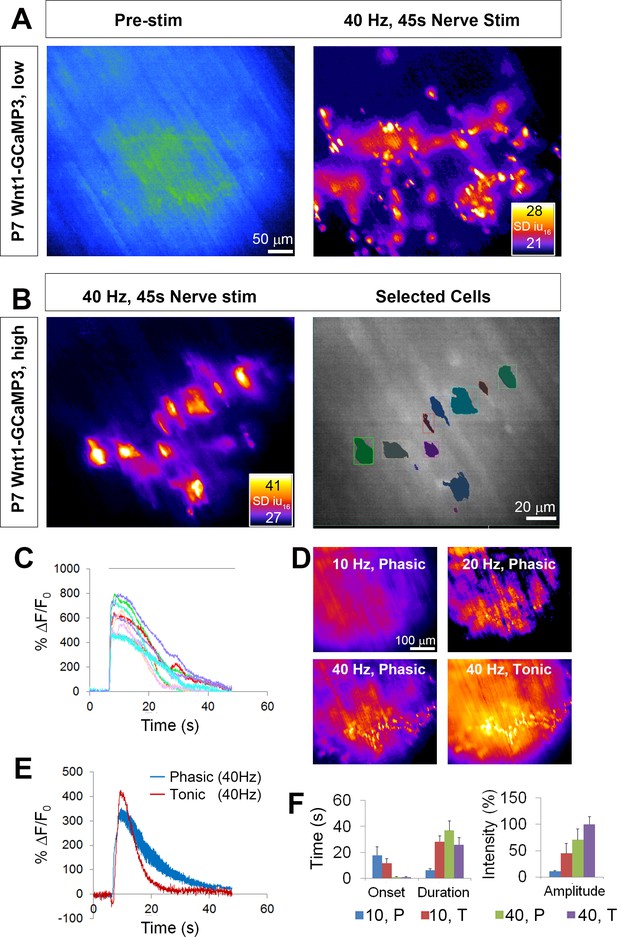
Wnt1-GCaMP3 mice exhibit activity-induced Ca2+responses in all perisynaptic glia cells of the neonatal diaphragm.
(A) (Left panel) An average intensity image generated before application of a stimulus (Pre-stim) shows the overall structure of GCaMP3-expressing Schwann cell elements. (Right panel) Map of standard deviation of 16-bit fluorescence intensity units (SD iu16) of a population of TPSCs imaged in response to high-frequency nerve stimulation at low magnification; fire CLUT heatmap in SD iu16. (B) Same muscle imaged at higher power showing these fluorescence responses in individual TPSCs (left panel) were color coded (right panel). (C) These cells in B were plotted as color-coded transients. Note the relatively higher signals in this graph, compared to other graphs in this study, as a result of imaging at 60X, vs. 20X in others. (D) SD maps resulting from tonic vs. phasic HFS. (E) Transients elicited by 40 Hz tonic (red) or (blue) phasic stimulation. Peak transient intensities were statistically non-significant (14.7 ± 0.4 vs. 13.5 ± 1.3 dB; p=0.21; n = 3; c = 18), and duration was longer (time to 50% decay = 15.4 ± 0.7 vs. 22.3 ± 3.7 s; p<0.05) in response to phasic vs. tonic stimulation. (F) Slower onsets and lower amplitudes of Ca2+ transients in TPSCs stimulated with 10 vs. 40 Hz stimulation (10 Hz phasic onset = 17.92 ± 6.3; 10 Hz tonic onset = 11.78 ± 3.4 s; 40 Hz phasic onset = 0.99 ± 0.34 s; 40 Hz tonic onset = 1.0 ± 0.38 s; p<5.3*10−19, 1-way ANOVA; every individual comparison significant except 40 Hz phasic onset vs. 40 Hz tonic onset, based on q, qcrit comparisons). 10 = 10 Hz; 40 = 40 Hz; T = tonic; p=phasic.
-
Figure 2—source data 1
These are fluorescence values of calcium transients of individual TPSCs taken at 60X (from P7 Wnt1-GCaMP3 mice), as depicted by the boxes in the right panel of Figure 2B, in response to 45 s of 40 Hz phrenic nerve stimulation, in the presence of the muscle-specific myosin inhibitor BHC.
Column A has time in seconds, columns B-K represent the fluorescence values of individual cells, in standard deviation of 16-bit intensity units (SD iu16). Column M has a background fluorescence trace. Columns O-X represent the fluorescence values of cells in B-K, minus the background fluorescence trace, normalized to a common starting value. Columns Z-AI represent the fluorescence/initial fluorescence ratio (∆f/f, in %) of the cells in B-K, used in Figure 2C. Finally, columns AK-AT represent the value in decibels of the cells in B-K.
- https://doi.org/10.7554/eLife.30839.005
-
Figure 2—source data 2
These are fluorescence values of calcium transients of individual TPSCs at P7 taken at 20X in response to 45 s of 40 Hz tonic or phasic phrenic nerve stimulation.
Averages of background-subtracted, normalized SD iu16 values were transformed into ∆f/f, in %, plotted and shown in Figure 2E. Below the plot, decibels were calculated for each of the samples and compared statistically.
- https://doi.org/10.7554/eLife.30839.006
-
Figure 2—source data 3
Mean values of the intensity of P7 TPSC calcium transients, in decibels, in response to 45 s of 10 Hz or 40 Hz phasic or tonic phrenic nerve stimulation, were collected and represented as % TPSC calcium transient in response to 45 s of 40 Hz tonic nerve stimulation.
The onset of these transients after the beginning of nerve stimulation, as well as the duration of the transients, were also collected and represented in these graphs in Figure 2F.
- https://doi.org/10.7554/eLife.30839.007
Perisynaptic glia at the neuromuscular junction exhibit activity-induced Ca2+signals in response to fatiguing, high-frequency nerve stimulation (HFS).
Brightfield video of a postnatal day 7 (P7) Wnt1-GCaMP3 diaphragm at 4X contracting in response to 45 s of 40 Hz tonic phrenic nerve stimulation (Top left video). Fluorescence video of same hemi-diaphragm at 20X contracting in response to HFS in the presence of the movement-blocking myosin inhibitor BHC shows TPSC Ca2+ responses (Top right video). Two consecutive fluorescence videos of same sample at 60X showing small populations of TPSCs, separated by a rest period between stimulations.
Perisynaptic glia at the neuromuscular junction exhibit activity-induced Ca2+signals in response to fatiguing levels of HFS even before the onset of fatigue.
A P7 Wnt1-GCaMP3 diaphragm was stimulated at 40 Hz for 0.1 s (top left video); 1 s (top middle video); 2 s (top right video); 5 s (bottom left video); 10 s (bottom middle video); 30 s (bottom right video). Each bout of HFS was separated by a rest period.
Schwann cells along distal axon branches, in addition to perisynaptic Schwann cells at the neuromuscular junction, exhibit Ca2+signals in response bath application of ATP.
A P7 Wnt1-GCaMP3 diaphragm was stimulated at 40 Hz for 45 s (left video) or treated with ATP (20 μM; right video). The boxes in each video surround a region that includes preterminal and distal axon branch-associated Schwann cells between NMJ-associated TPSCs, which exhibit a Ca2+ response in response to ATP but not nerve stimulation.
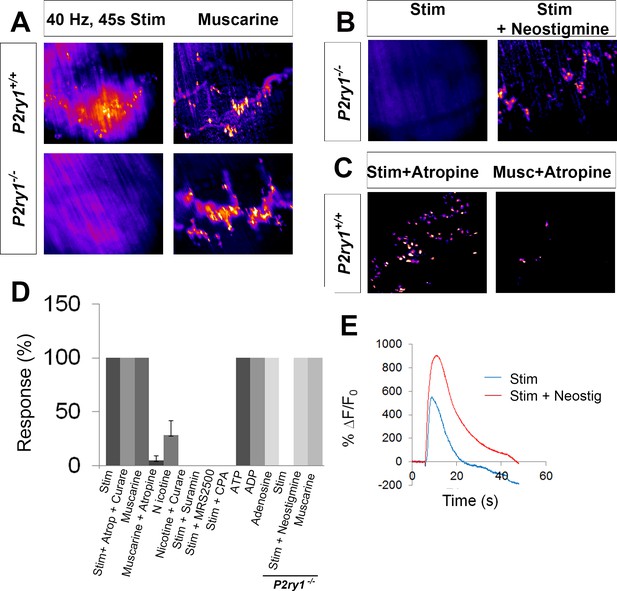
Activity-induced responses in perisynaptic glia at the NMJ are completely dependent on P2Y1R signaling.
(A) SD maps of activity-induced (left panels) or muscarine-induced (right panels) Ca2+ responses in TPSCs of P2ry1 wild-type (WT; top panels) or mutant (bottom panels) diaphragm. Note the complete absence of activity-induced Ca2+ responses in TPSCs of P2ry1 mutants; fire CLUT heatmap in SD iu16. (B) Addition of neostigmine restores this response. (C) Atropine largely blocks muscarine-induced, but not nerve stimulation-induced, TPSC Ca2+ responses. (D) Graph representing the relative number of α-BTX-associated TPSCs exhibiting Ca2+ responses in response to activity or drug treatment. p<5.3*10−19, 1-way ANOVA; Nicotine treatment induced a variable response (28.3 + 13.3% of stim-activated cells activated by nicotine; variance = 176.3). (E) WT mice exhibit elevated Ca2+ responses to activity in the presence of the cholinesterase-blocking drug neostigmine (peak TPSC Ca2+ intensities, 16.3 ± 1.4 vs. 20 ± 2.3 dB, stim vs. stim + neostigmine, p<0.05; n = 4; c = 22).
-
Figure 3—source data 1
The number of P7 TPSCs responding (displaying a calcium transient) to each of the conditions were collected and represented as the percent of TPSCs responding to 45 s of 40 Hz phrenic nerve stimulation.
These values were subjected to 1-way ANOVA and are plotted in Figure 3D.
- https://doi.org/10.7554/eLife.30839.014
-
Figure 3—source data 2
These are fluorescence values of calcium transients of individual TPSCs from P7 WT mice, taken at 20X in response to 45 s of 40 Hz tonic phrenic nerve stimulation, in the presence of absence of the wide spectrum cholinesterase inhibitor neostigmine.
Averages of background-subtracted, normalized SD iu16 values were transformed into ∆f/f, in %, plotted and shown in Figure 3E. Below the plot, decibels were calculated for each of the samples and compared statistically.
- https://doi.org/10.7554/eLife.30839.015
-
Figure 3—source data 3
These are the diameters in square microns of synaptophysin-immunoreactive presynaptic terminals of P7 P2ry1 WT and mutant mice, shown in Figure 3—figure supplement 1.
- https://doi.org/10.7554/eLife.30839.016
-
Figure 3—source data 4
These are the depths in microns of the junctional folds of the postsynaptic muscle membrane of P7 P2ry1 WT and mutant mice, shown in Figure 3—figure supplement 2.
- https://doi.org/10.7554/eLife.30839.017
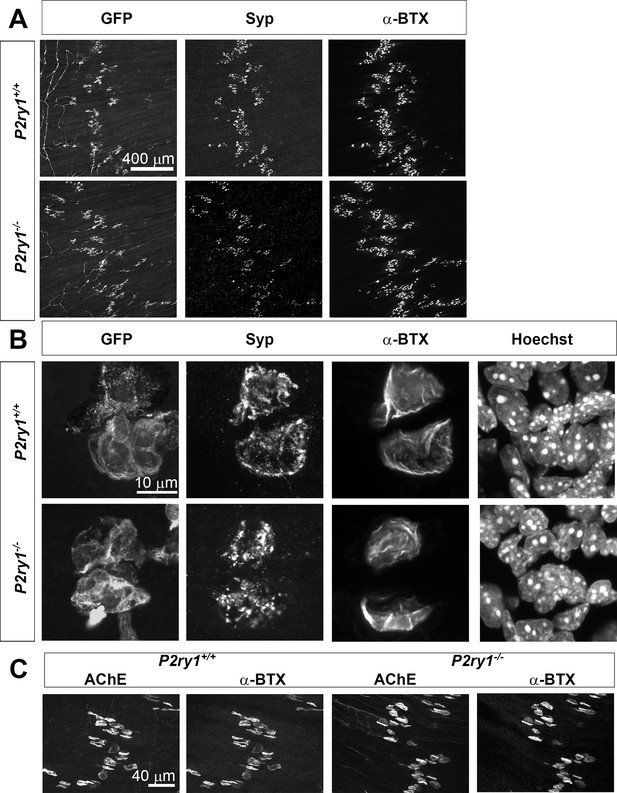
Normal expression of pre-, peri- and post-synaptic elements at the NMJ of P2ry1 mutant mice lacking activity-induced Ca2+responses in perisynaptic glia.
(A) Low-power micrographs of confocal images generated from P7 diaphragms of P2ry1 WT (top row) and mutant (bottom row) diaphragm stained with antibodies against GFP (to label GCaMP3-expressing perisynaptic Schwann cells), synaptophysin (Syp; to label presynaptic nerve terminals) and 633-conjugated α-BTX (to label postsynaptic ACh receptor clusters). All NMJs are innervated and display terminal/perisynaptic Schwann cells (TPSCs). (B) High-power micrographs of the same tissue also stained with bisbenzamide (Hoechst) exhibit similar patterns of synaptic staining between genotypes. Synaptophysin-immunoreactive presynaptic terminal area, 267 ± 43 vs. 272 ± 47 μm2, p=0.85, P2ry1 WT vs. mutant, terminals = 7, n = 3. (C) α-BTX-labeled NMJs in both P2ry1 WT (left panels) and mutant (right panels) exhibit robust AChE staining.
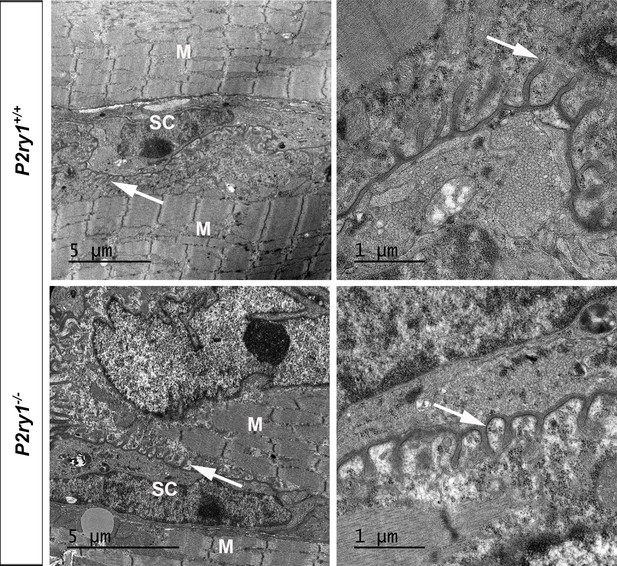
Normal ultrastructural appearance of the NMJ of P2ry1 mutant mice lacking activity-induced Ca2+responses in perisynaptic glia.
Low-power (left) and high-power (right) photomicrographs of NMJs from the diaphragm of P7 P2ry1 WT (top panels) and mutant (bottom panels). Junctional folds indicated by arrows; TPSCs (SC) and muscle cells (M). Individual fold depth, 0.49 ± 0.15 vs. 0.43 ± 0.07 μm, p=0.31, P2ry1 WT vs. mutant, folds = 8, n = 3.
Activity-induced Ca2+responses in perisynaptic glia at the neuromuscular junction are mediated by P2Y1Rs.
Three different P7 P2ry1 WT diaphragms exhibit Ca2+ responses to 45 s of 40 Hz (left column). Three different P7 P2ry1 mutant diaphragms fail to exhibit Ca2+ responses to HFS (middle column) but do exhibit responses to bath application of muscarine (right column).
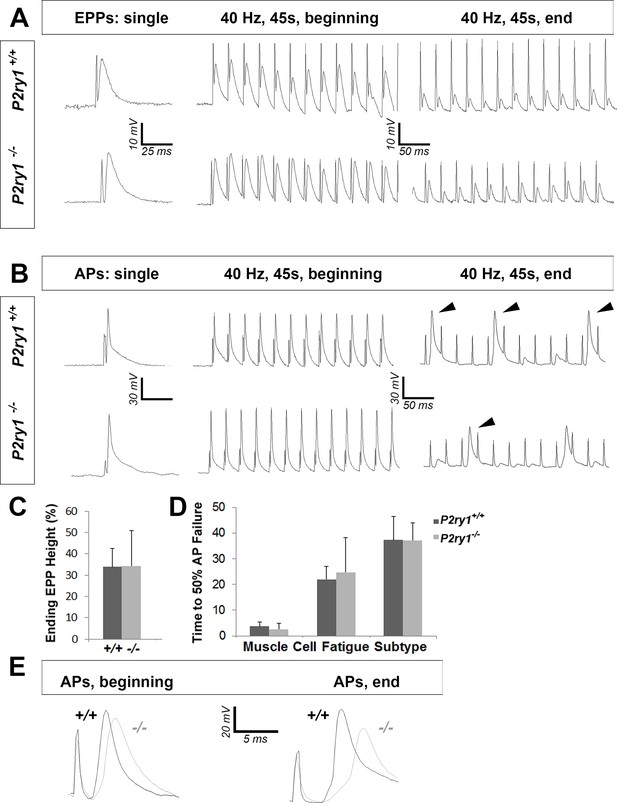
The loss of activity-induced Ca2+responses in perisynaptic glia disrupts postsynaptic but not presynaptic function at the NMJ.
(A) Phrenic nerve-evoked endplate potentials (EPPs) were measured in P7 diaphragm muscle from P2ry1 WT and mutant mice in response to basal (left panel) and high-frequency stimulation (HFS; middle and right panels). There was no difference in the amplitudes (24 ± 2.6 vs. 26.7 ± 1.5 mV; p=0.13; WT vs. mutant; n = 4; c = 16) of basal EPPs or in the amplitudes of EPPs at the end of a period of HFS. Up and downward deflections preceding each EPP are stimulation artifacts. (B) Phrenic nerve-evoked muscle action potentials (APs) were measured in P7 diaphragm muscle from P2ry1 WT and mutant mice in response to basal (left panel) and HFS (middle and right panels). There was no difference in the amplitudes (67.7 ± 7.1 vs. 67.8 ± 7.1 mV; p=0.49), the rise to peak (1.16 ± 0.2 vs. 1.07 ± 0.2 ms; p=0.33), or the time to 50% decay (2.42 ± 0.53 vs. 2.16 ± 0.82 ms; p=0.43) of basal APs, or in the percentage of successfully transmitted muscle APs at the end of a period of HFS (note the three successful APs in the WT and 2 APs in the mutant, at the end of HFS; arrowheads). (C) As shown in right panel of A, ending EPP heights are similar between genotypes (34 ± 8.6 vs. 34.3 ± 16.6% initial EPP; p=0.96; WT vs. mutant; n = 4; c = 20). (D) As shown in right panel of B, the time to 50% failure in response to HFS is similar between genotypes, in all subtypes of fatiguability (3.7 ± 1.6 vs. 2.5 ± 2.4 s for quick fatiguability; p=0.23; 22 ± 15.1 vs. 24.7 ± 13.5 s for intermediate fatiguability; p=0.67; 37.3 ± 9 vs. 37.2 ± 6.8 s for slow fatiguability; p=0.75; WT vs. mutant; n = 4; c = 27). (E) Muscle APs from the beginning (left) or end of a train of HFS from P2ry1 WT (black; +/+) or mutant (gray; -/-) mice. Muscle AP rise-to-peak was lengthened (2.0 ± 0.6 vs. 2.7 ± 0.7 ms; WT vs. mutant, p<0.05) and amplitude was reduced (58.1 ± 3.23 vs. 53.7 ± 4.5 mV; WT vs. mutant, n = 4, c = 13; p<0.05) at the end of a train of HFS.
-
Figure 4—source data 1
These are the amplitudes of intracellularly recorded muscle endplate potentials (EPPs), relative to initial EPP amplitudes, in %, at the end of a 45 s, 40 Hz train of phrenic nerve stimulation (each value represents the average of at least 3 EPPs for that particular cell, and each animal has 4–5 cells).
These values were calculated for P7 P2ry1 WT (Columns B-E) and mutant (columns H-L) and compared statistically. Single EPP amplitudes (basal) were also calculated for each genotype (Columns O-Q and U-X) and compared. This data is shown in Figure 4C.
- https://doi.org/10.7554/eLife.30839.020
-
Figure 4—source data 2
– These values represent the time at which different muscle cell types exhibit neural transmission failure, as measured by the time at which the number of successfully transmitted muscle action potentials (APs) dropped below 50% in response to 45 s of 40 Hz phrenic nerve stimulation.
Red represents cells with quick time to failure (presumptive Type IIB cells), green equals represents cells with an intermediate time to failure (IIA) and blue those with the slowest time to failure. Cells C49-51 represent this value from P7 P2ry1 WT and Cells I49-51 this value from P7 P2ry1 mutants. These values were rewritten in cells T-U to make the graph in Figure 4D.
- https://doi.org/10.7554/eLife.30839.021
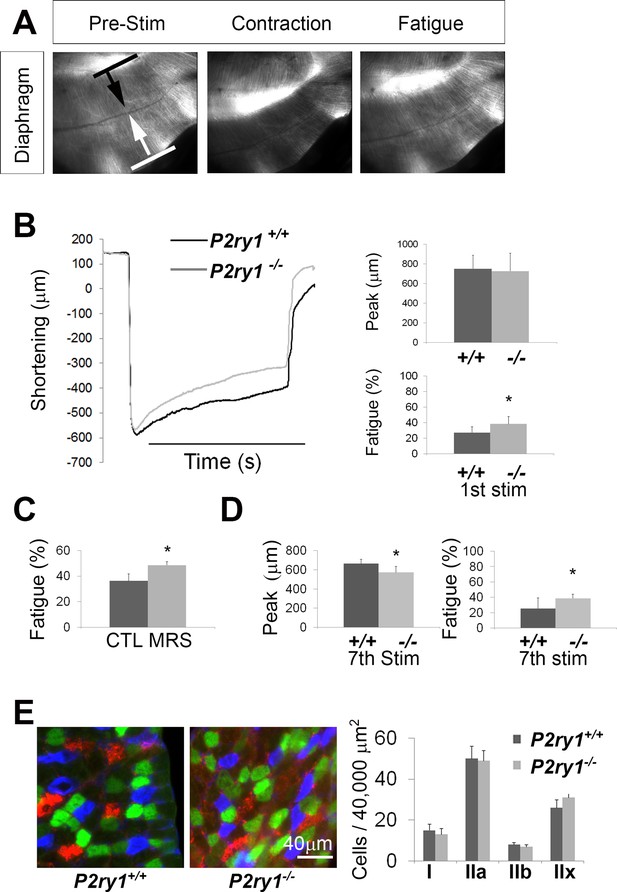
The loss of activity-induced Ca2+responses in perisynaptic glia leads to enhanced muscle fatigue.
(A) Images of a P7 hemi-diaphragm before phrenic nerve stimulation, at peak contraction, and during fatigue. Black and white arrows indicate sites used to compare fiber length changes. (B) The shortening of muscle fibers, as measured by the change in distance between the two sites indicated by arrows in A, is represented as a negative number. Peak length changes (shortening) are similar between genotypes (751.6 ± 136 μm vs. 726 ± 182 μm; p=0.75), but are maintained significantly less over time in P2ry1 mutants (ending length change = 72.6 ± 7.3 vs. 61.5 ± 9.5% peak length change; n = 9; P2ry1 WT vs. mutant; *p<0.05). (C) Fatigue is also enhanced by acute blockade of TPSC Ca2+ responses with the P2Y1R antagonist MRS2500 (ending length change = 63.7 ± 10.8 vs. 51.4 ± 5.3% peak length change; n = 4; untreated vs. MRS2500-treated; *p<0.05; data in graph presented as the failure to maintain peak shortening or 100% minus these values). (D) Peak length changes in response to the seventh bout of HFS are reduced in P2ry1 mutants (664.6 ± 43.7 μm vs. 564.4 ± 64.1 μm; *p<0.05), as is fatigue (ending length change = 74.5 ± 13.6 vs. 61.3 ± 5.8% peak length change; n = 4; P2ry1 WT vs. mutant; *p<0.05). (E) Image of transverse section of P7 diaphragm from P2ry1 WT and mutant mice, stained with antibodies against myosin heavy chain (MHC) Type I (blue), MHC Type IIA (green) and MHC Type IIB (red) antibodies (left panel). No difference between genotypes was observed in the number of each MHC muscle fiber subtype (15 ± 3 vs. 13 ± 3, p=0.46; Type I; 50 ± 6 vs. 49 ± 5, p=0.83; Type IIa; 8 ± 2 vs. 7 ± 2, p=0.59; Type IIb; 26 ± 4 vs. 31 ± 5, p=0.36; Type IIx; all values are P2ry1 WT vs. mutant; n = 3).
-
Figure 5—source data 1
These muscle shortening and fatigue curves were taken from brightfield videos of hemi-diaphragms of P7 P2ry1 WT and mutant mice subjected to 45 s of 40 Hz phrenic nerve stimulation.
The values represent the difference, in microns, of the distance between the two edges of the diaphragm, relative to their starting value. So for example, the starting difference is small because the two edges have not moved yet (i.e., have not contracted yet). When contraction occurs, the two edges move closer together, representing a negative distance from their starting positions (i.e., ‘shortening’). The peak values are the most negative numbers and are conceptually correlated to peak tension values. As the muscle fatigues, the values depart from this peak shortening value and accordingly become less negative. Fatigue curves are shown in the left side of Figure 5B. The values for peak contraction and ending contraction, relative to peak contraction (fatigue) were calculated and are shown in the pair of bar graphs in the right side of Figure 5B.
- https://doi.org/10.7554/eLife.30839.024
-
Figure 5—source data 2
These values for ending contraction, relative to peak contraction (fatigue) were taken from brightfield videos of hemi-diaphragms of P7 P2ry1 WT control or MRS2500-treated mice, subjected to 45 s of 40 Hz phrenic nerve stimulation in Figure 5C.
- https://doi.org/10.7554/eLife.30839.025
-
Figure 5—source data 3
These values for peak contraction and ending contraction, relative to peak contraction (fatigue) were taken from brightfield videos of hemi-diaphragms of P7 P2ry1 WT and mutant mice, subjected to 45 s of 40 Hz phrenic nerve stimulation for seven consecutive episodes.
The values comparing these genotypes that are shown in Figure 5D are for the 7th stimulation.
- https://doi.org/10.7554/eLife.30839.026
-
Figure 5—source data 4
These are the numbers of different muscle fiber subtypes in images of diaphragm muscle from P7 P2ry1 WT and mutant mice, based on myosin heavy chain immunostaining.
- https://doi.org/10.7554/eLife.30839.027
-
Figure 5—source data 5
These are fluorescence values of muscle calcium transients (from P7 Myf5-GCaMP3 mice) taken at 20X in response to 45 s of 40 Hz phrenic nerve stimulation for two episodes.
In one case, MRS2500 was added in between these two episodes. Values for each of the two episodes, in response to control or MRS2500 treatment, were plotted as ∆f/f, in %, based on background-subtracted, normalized SD iu16 values, and shown in Figure 5—figure supplement 1A,B.
- https://doi.org/10.7554/eLife.30839.028
-
Figure 5—source data 6
These are fluorescence values of muscle calcium transients (from P7 Myf5-GCaMP3 mice) taken at 20X in response to either 45 s of 40 Hz phrenic nerve or treatment with ADP, represented as SD map images in Figure 5—figure supplement 1C.
- https://doi.org/10.7554/eLife.30839.029
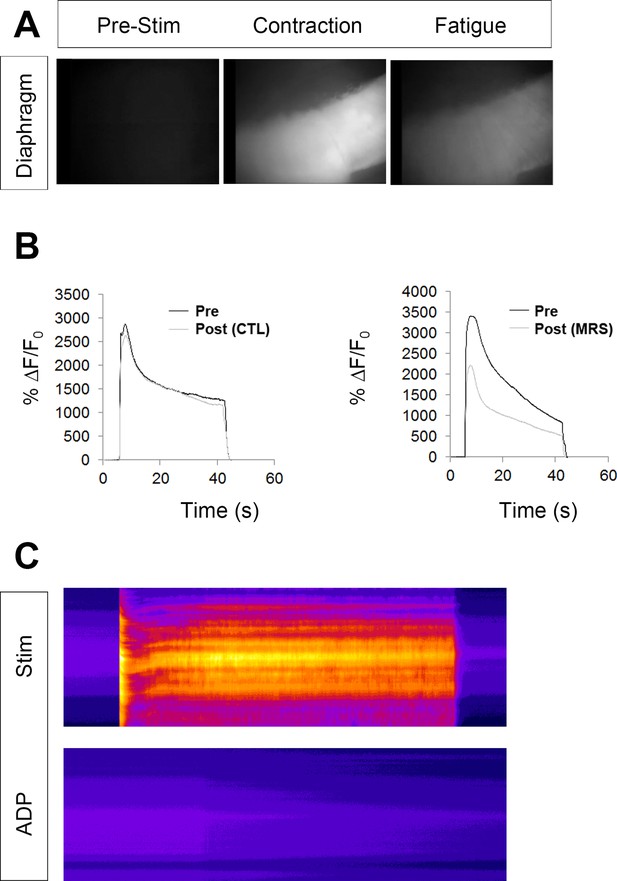
The loss of activity-induced Ca2+responses in perisynaptic glia leads to greater and quicker loss of peak muscle Ca2+transient intensity.
(A) Images of a P7 hemi-diaphragm from a Myf5-GCaMP3 mouse before phrenic nerve stimulation, at peak contraction, and during fatigue. (B) Left graph: Peak intensities (28.8 ± 0.4 vs. 29.5 ± 1.3 vs. dB, p=0.2), time to 50% decay (32.8 ± 8.7 vs. 26.7 ± 8.2 s, p=0.2), and ending relative to peak intensities (ending intensity = 45.0 ± 5.4 vs. 43.8 ± 3.1% peak intensity; second vs. first HFS; p=0.38, n = 3) are unchanged in response to a second HFS bout, vs. a first one. Right graph: peak intensities are reduced (27.3 ± 0.8 vs. 30.9 ± 0.8 dB p<0.005), time to 50% decay is enhanced (18.7 ± 1.9 vs. 23.7 ± 2.6 s; p<0.05), and ending relative to peak intensities are unchanged (ending intensity = 27.3 ± 4.3 vs. 24.3 ± 3% peak intensity; p<0.05; second vs. first HFS; p=0.18, n = 3), in response to a second HFS bout in the presence of MRS2500, vs. a first one in the absence of this drug. (C) In contrast to nerve stimulation of the same diaphragm (stim), treatment with 100 μM ATP fails to elicit a Ca2+ response in muscle cells expressing GCaMP3 (15.52 ± 4.1 vs. 0.01 ± 0.12 dB; p<0.005, n = 3).
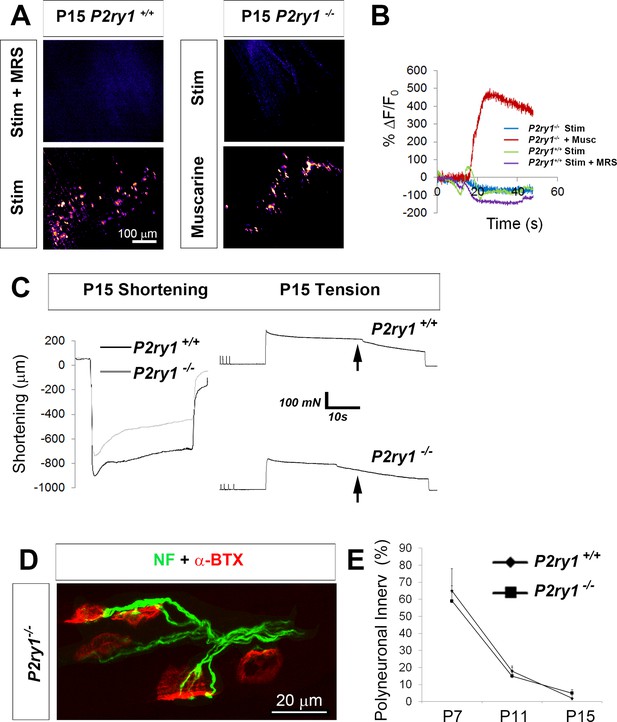
The loss of activity-induced Ca2+responses in perisynaptic glia does not affect the rate or magnitude of polyneuronal synapse elimination.
(A) Pharmacological or genetic disruption of P2Y1R signaling completely blocks activity-induced TPSC Ca2+ responses at P15: P2ry1 WT mice exhibited nerve stimulation-induced responses that were blocked after treatment with 1 μM MRS2500 (left images). P2ry1 mutant mice failed to exhibit nerve stimulation-induced Ca2+ responses in TPSCs but exhibited robust responses to bath-applied muscarine (right images). (B) Peak TPSC Ca2+ intensities in response to these manipulations: (5.4 ± 1.2 vs. 1.5 ± 0.56 dB, WT stim vs. mutant stim, p<0.005; 5.4 ± 1.2 vs. 0.1 ± 0.04 dB, WT stim vs. WT stim +MRS2500, p<0.0001; 15.6 ± 0.7 vs. 1.5 ± 0.56 dB, mutant stim vs. mutant +muscarine, p<0.0001, Student’s t with Bonferonni correction; c > 10 per n; n = 3–4; fluorescence units from fire CLUT heatmap in SD iu16. (C) Fatigue is enhanced in P15 P2ry1 mutants using optical measures (ending length change = 75.9 ± 8 vs. 58.6 ± 11.6% peak length change; n = 3; P2ry1 WT vs. mutant; p<0.05) or tension recording (ending force = 39.2 ± 5.1 vs. 32.3 ± 4.3% peak force; n = 3; P2ry1 WT vs. mutant; p<0.05). Drop in force occurs earlier in mutant vs. WT (arrows). (D) Polyneuronally innervated NMJs from a P7 P2ry1 mutant diaphragm; red = AlexaFluor 594-labeled α-BTX to label AChRs; green = neurofilament immunostaining to label presynaptic nerve terminals. (E) The number of polyneuronally innervated NMJs is similar between P2ry1 WT and mutant mice (P7: 65 ± 9 vs. 59 ± 13%, p=0.41; P11: 18 ± 5 vs. 15 ± 3%, p=0.34; P15: 2 ± 2 vs. 5 ± 3%, p=0.19 polyneuronally innervated NMJs; n = 5; P2ry1 WT vs. mutant).
-
Figure 6—source data 1
These are fluorescence values of calcium transients of individual TPSCs from P15 P2ry1 WT and mutant mice, or P15 P2ry1 WT mice treated with MRS2500, taken at 20X in response to 45 s of 40 Hz phrenic nerve stimulation or in response to muscarine.
Averages of background-subtracted, normalized SD iu16 values were transformed into ∆f/f, in %, plotted and shown in Figure 6B. Below the plot, decibels were calculated for each of the samples and compared statistically.
- https://doi.org/10.7554/eLife.30839.031
-
Figure 6—source data 2
These muscle shortening and fatigue curves were taken from brightfield videos of hemi-diaphragms of P15 P2ry1 WT and mutant mice subjected to 45 s of 40 Hz phrenic nerve stimulation and are shown in Figure 6C.
- https://doi.org/10.7554/eLife.30839.032
-
Figure 6—source data 3
These values represent the number of multiply innervated NMJs in the diaphragm at P7, P11 and P15 in P2ry1 WT and mutant mice and are shown in Figure 6D.
- https://doi.org/10.7554/eLife.30839.033
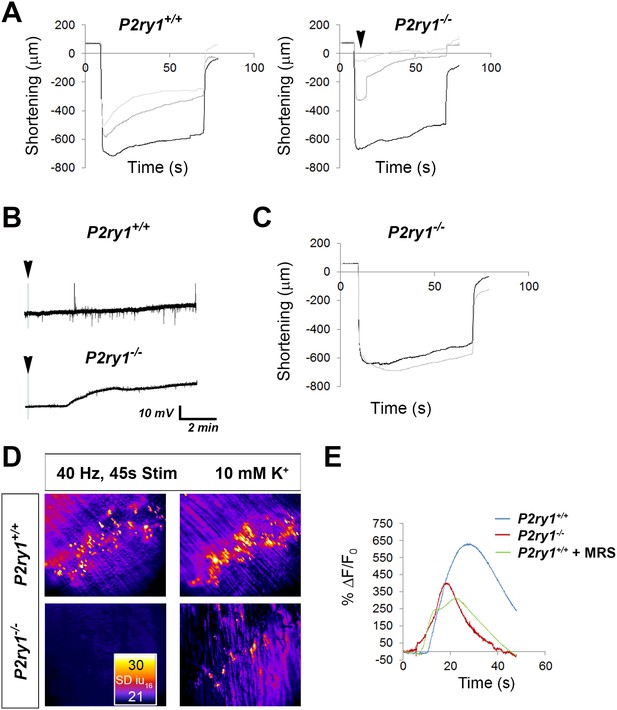
High [K+o] triggers greater muscle fatigue, greater muscle membrane depolarization, and reduced Ca2+responses in the absence of activity-induced Ca2+responses in perisynaptic glia.
(A) Muscle length changes from P7 diaphragm of P2ry1 WT (left) and mutant (right) mice were first recorded in response to HFS in normal or 5 mM [K+]o (black traces), and then recorded in response to 2 bouts of HFS (60 Hz, 45 s) in 10 mM [K+]o (dark and light grey traces = 1st and 2nd HFS, respectively). Peak length changes and fatigue are dramatically affected in P2ry1 mutants by high [K+o]: peak length in response to first HFS in 10 mM [K+]o as a percentage of peak length in response to control HFS in 5 mM [K+]o = 93 ± 20.7 vs. 53.8 ± 24%; n = 3; P2ry1 WT vs. mutant mice, p<0.05; peak length change in response to second HFS in 10 mM [K+]o = 74.2 ± 15.5 vs. 20.9 ± 11% control HFS; n = 3; P2ry1 WT vs. mutant mice; p<0.005; asterisk indicates this almost complete failure to contract; fatigue, or ending length change of second HFS in high 10 mM [K+]o = 41.3 ± 17.8 vs. 7.4 ± 6.8 peak length change of first HFS in 5 mM [K+]o; n = 3; P2ry1 WT vs. mutant mice, p<0.05). (B) Muscle length changes in P2ry1 mutants in response to HFS in lowered [K+]o show a statistically non-significant trend toward less fatigue (ending length change, 2.5 mM [K+]o (grey trace) vs. 5 mM [K+]o (black trace)=75.1 ± 6.2 vs. 83.9 ± 12.9% peak length change; n = 3, p=0.17). (C) Effect of high [K+]o on resting membrane potential (RMP). Representative muscle cell recording before and after (arrowhead) [K+]o was changed from 5 to 10 mM in P7 diaphragm from P2ry1 WT (left) and mutant (right). (D) SD intensity maps of TPSCs in response to HFS (left panels) in P2ry1 WT (upper) and mutant (lower) mice and in response to subsequent treatment with 10 mM [K+]o (right panels). Markedly fewer TPSCs responded to elevated [K+]o in P2ry1 mutants. (E) Peak Ca2+ transient intensities of TPSCs responding to 10 mM [K+]o after 3 bouts of HFS were significantly reduced in P2ry1 mutants or in WT mice treated with the P2Y1R antagonist MRS2500 (18.6 ± 2.5 vs. 14.5 ± 1.5 dB, WT vs. mutant, p<0.05, c > 10 per n; n = 4, Student’s t with Bonferonni correction; 18.6 ± 2.5 vs. 13.8 ± 0.8 dB, WT vs. WT + MRS2500, p<0.01, c > 10 per n; n = 4, Student’s t with Bonferonni correction; fluorescence units from fire CLUT heatmap in SD iu16).
-
Figure 7—source data 1
These muscle shortening and fatigue curves were taken from brightfield videos of hemi-diaphragms subjected to 45 s of 60 Hz phrenic nerve stimulation in the presence of normal or high levels of extracellular potassium in P7 P2ry1 WT mice.
Muscles were first stimulated for one episode in the presence of regular or 5 mM potassium chloride (5 mM; black line) and then stimulated for two episodes in the presence of 10 mM potassium chloride (dark and light grey lines). Fatigue curves are shown in the left side of Figure 7A. The values for peak contraction and ending contraction, relative to peak contraction (fatigue) were also calculated.
- https://doi.org/10.7554/eLife.30839.035
-
Figure 7—source data 2
These muscle shortening and fatigue curves were taken from brightfield videos of hemi-diaphragms subjected to 45 s of 60 Hz phrenic nerve stimulation in the presence of normal or high levels of extracellular potassium in P7 P2ry1 mutant mice.
- https://doi.org/10.7554/eLife.30839.036
-
Figure 7—source data 3
These muscle shortening and fatigue curves were taken from brightfield videos of hemi-diaphragms subjected to 45 s of 60 Hz phrenic nerve stimulation in the presence of normal or low levels of extracellular potassium in P7 P2ry1 mutant mice and shown in Figure 7C.
- https://doi.org/10.7554/eLife.30839.037
-
Figure 7—source data 4
These are fluorescence values of calcium transients of individual TPSCs from P7 P2ry1 WT and mutant mice, or P7 P2ry1 WT mice treated with MRS2500, taken at 20X in response to treatment with 10 mM potassium chloride.
Averages of background-subtracted, normalized SD iu16 values were transformed into ∆f/f, in %, plotted and shown in Figure 7E. Below the plot, decibels were calculated for each of the samples and compared statistically.
- https://doi.org/10.7554/eLife.30839.038
Treatment with high levels of potassium markedly enhances fatigue in P2ry1 mutant mice lacking activity-induced Ca2+responses in perisynaptic glia at the neuromuscular junction.
P7 P2ry1 WT diaphragm (top row) or P2ry1 mutant diaphragm (bottom row) were stimulated at 40 Hz for 45 s in 5 mM [K+]o (left column), then changed to 10 mM [K+]o, and stimulated two times more (right column = 2nd stimulation in HFS), each time separated by a 30 min rest period. Note the severe inability of the P2ry1 mutant video to contract in response to the second bout of HFS in 10 mM [K+]o.

Proposed model by which nerve stimulation-induced Ca2+responses in TPSCs regulate muscle fatigue.
Upon stimulation, presynaptic motor axon terminals (green) release acetylcholine (ACh) from synaptic vesicles (SVs), which elicits endplate potentials via postsynaptic nicotinic ACh receptors (nAChR), followed by activation of voltage-gated sodium channels (VGSC), leading to action potentials and contraction of muscle (brown). Nerve terminals also release ATP, which as itself or ADP stimulates in TPSCs (yellow) the release of calcium (Ca2+) from intracellular stores via P2Y1 receptors (P2Y1R). This signal leads to the movement into TPSCs of perisynaptic potassium (K+), produced by both neurons and muscle cells in response to stimulation. This regulation of perisynaptic K+ levels by TPSCs is proposed to reduce the inactivation of VGSCs by K+ in the neuromuscular synapse during repetitive stimulation, thus reducing muscle fatigue.
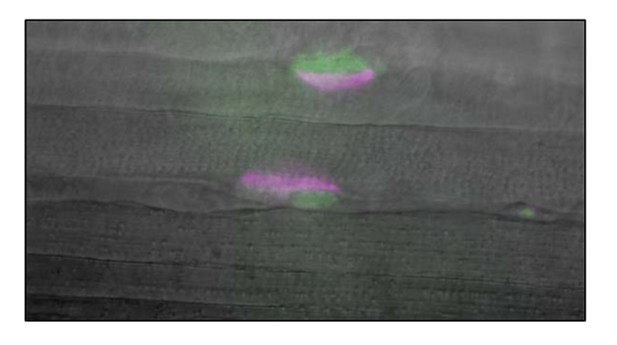
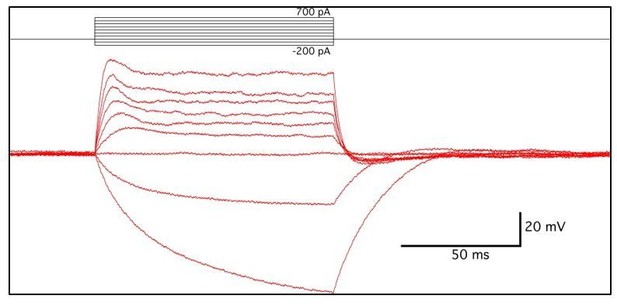
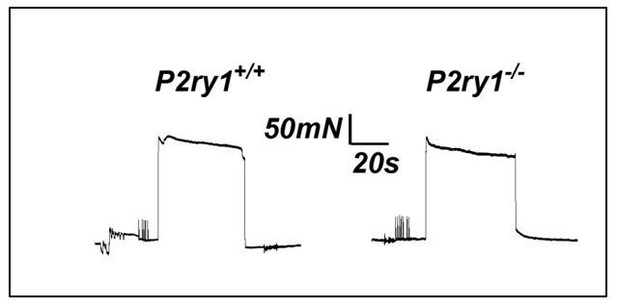
Tables
Reagent type (species) or resource | Designation | Source or reference | Identifiers |
---|---|---|---|
strain, strain background (mus musculus) | Wnt1-Cre | Jax | 9107 |
strain, strain background (mus musculus) | Myf5-Cre | Jax | 7893 |
strain, strain background (mus musculus) | conditional GCaMP3 | Jax | 14538 |
strain, strain background (mus musculus) | conditional GCaMP6f | Jax | 24105 |
strain, strain background (mus musculus) | conditional tdTomato | Jax | 7905 |
strain, strain background (mus musculus) | P2ry1 mutant | Jax | 9131 |
chemical compound, drug | BHC | Hit2lead | 5102862 |
Additional files
-
Source code 1
The logic underlying several methods used in Volumetry to generate standard deviation of fluorescence intensity map and spatio-temporal maps for TPSC Ca2+ imaging, as well as tissue distortion using edges for muscle shortening and fatigue.
Each method is broken down conceptually into several categories, including variables needed, storage buffers, and approach taken. This logic can be utilized across a wide variety of software programs.
- https://doi.org/10.7554/eLife.30839.041
-
Transparent reporting form
- https://doi.org/10.7554/eLife.30839.042