Optogenetically induced low-frequency correlations impair perception
Figures
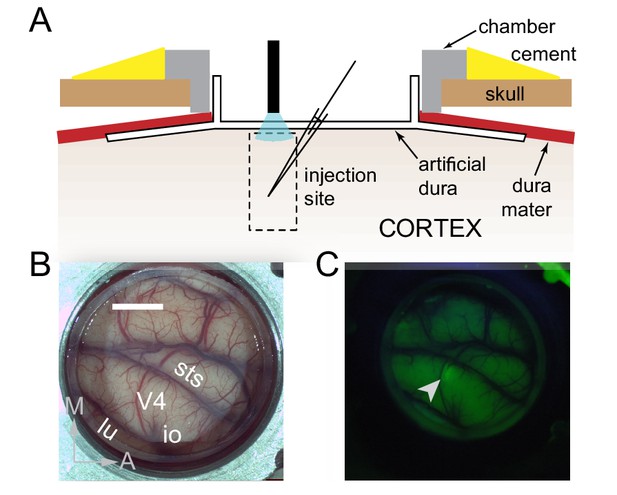
Surface Optogenetics and electrophysiology through an artificial dura.
(A) Schematic of an artificial dura (AD) chamber. A portion of the native dura mater (red) is resected and replaced with a silicone based optically clear artificial dura (AD). The optical clarity of the AD allows precisely targeted injections of viral constructs and subsequent optical stimulation and electrophysiological recordings. (B) An AD chamber is shown over dorsal V4 in the right hemisphere of Monkey A. sts = superior temporal sulcus, lu = lunate sulcus, io = inferior occipital sulcus. Area V4 lies on the pre-lunate gyrus between the superior temporal and lunate sulci. Scale bar = 5 mm; M = medial, A = anterior (C) EYFP expression at the first injection site (lenti-CaMKIIα-C1V1-ts-EYFP) after 4 weeks.
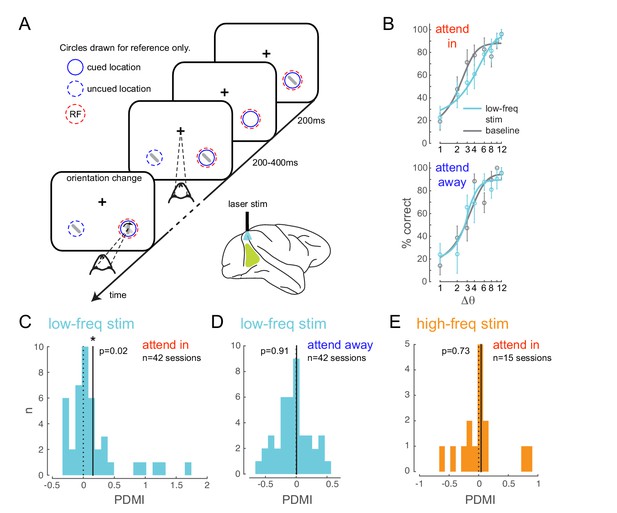
Optogenetically-induced low-frequency correlations cause a frequency- and spatially-selective impairment in an attention-demanding orientation discrimination task.
(A) Attention task: While the monkey maintained fixation, two oriented Gabor stimuli (schematized as oriented bars) flashed on and off simultaneously at two spatial locations: one at the RF of the opsin injection site, the other at a location of equal eccentricity across the vertical meridian. The monkey was cued to covertly attend to one of the two locations. At an unpredictable time, one of the two stimuli changed in orientation. The monkey was rewarded for making a saccade to the location of orientation change at either location (95% probability of change at cued location; 5% probability at un-cued location [foil trials]). If no change occurred (catch trials), the monkey was rewarded for maintaining fixation. On a random subset of trials, the opsin site was optically stimulated using a low-frequency (4-5 Hz) sinusoidally modulated laser light (). (B) Psychometric functions for an example behavioral session showing performance (hit rate) as a function of task difficulty (size of orientation change) for the baseline (no optical stimulation) condition in gray and low-frequency optical stimulation condition in blue. Top, monkey was instructed to attend to the site of optical stimulation; Bottom, monkey was instructed to attend to the contralateral hemifield. Error bars are std. dev. obtained by a jackknife procedure and corrected for the number of jackknives (20). The data has been fitted with a smooth logistic function. (C) The perceptual discrimination modulation index (PDMI; change in psychometric function threshold due to optical stimulation) in the low-frequency optical stimulation condition when the monkey was attending in to the site of optical stimulation across all behavioral sessions. The solid line represents the mean of the distribution. The PDMI distribution is significantly different from zero. (D–E) No significant change in PDMI either when the monkey was attending away from the site of optical stimulation (D) or due to high-frequency optical stimulation (E).
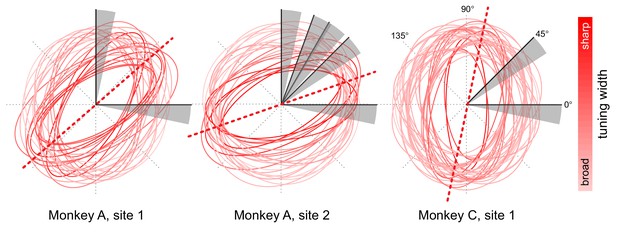
Orientation tuning properties at opsin injection sites.
Orientation tuning plots of single units and multi-unit activity at the different cortical injection sites (two in monkey A, one in monkey C) were fitted with ellipses (least-squares fit). The fitted ellipses are overlaid to illustrate the overlap in tuning at each injection site. The ellipses are color-coded such that more saturated colors correspond to units with sharper orientation tuning, as estimated from the aspect ratio of the fitted ellipse. The dotted red lines represent the average peak tuning at each site. The gray sectors represent the range of orientation changes that the monkeys had to detect with respect to baseline orientation (black line within each sector) across different behavioral sessions.
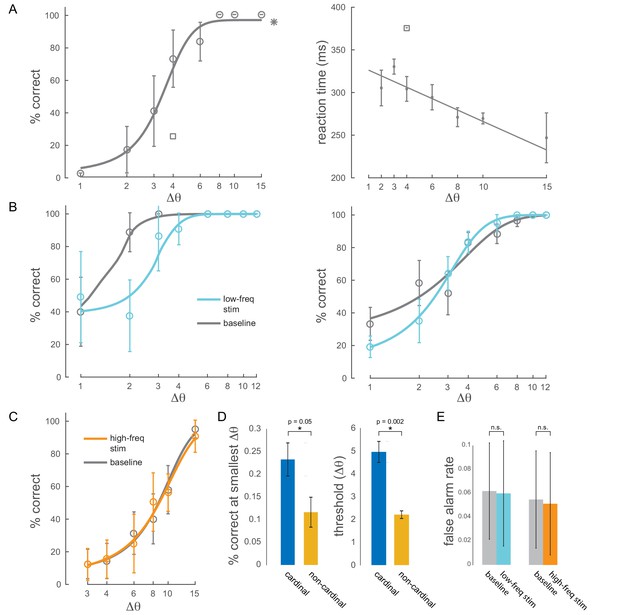
Behavioral performance.
(A) Left panel: example behavioral session showing performance (hit rate) as a function of task difficulty (size of orientation change) for the baseline condition (no optical stimulation). Square symbol: foil-trial performance. Star symbol: catch-trial performance. Error bars are std. dev. obtained by a jackknife procedure and corrected for the number of jackknives (20). The data has been fitted with a smooth logistic function. Right panel: reaction time as a function of task difficulty for the same session. The data has been fitted with a linear regression line. Performance is degraded and reaction times are slower for the foil trials, indicating that the animal was indeed deploying attention to the spatially cued location. (B) Two example sessions showing a behavioral impairment due to low-frequency (4-5Hz) optical stimulation when the monkey was attending in to the site of optical stimulation. Same format as in Figure 2B, top panel. (C) Example behavioral session showing no change in performance due to high-frequency (20Hz) optical stimulation. (D) Performance at the smallest target orientation change and discrimination thresholds for cardinal (0°, 90°) and non-cardinal non-target orientations. Mean +/- s.e.m. (E) False alarm rates for baseline, low-frequency stimulation and high-frequency stimulation conditions ( sessions for low-frequency; sessions for high-frequency).
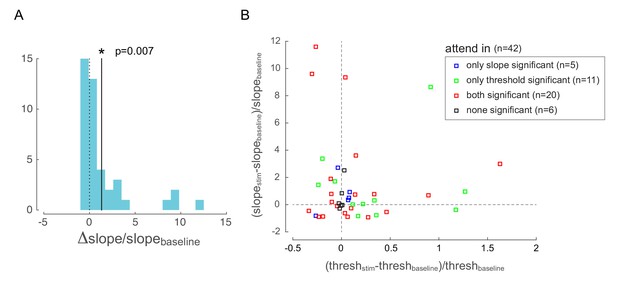
Behavioral changes with optical stimulation.
(A) Change in psychometric function slope due to low-frequency optical stimulation when the monkey was attending in to the site of optical stimulation. The solid line represents the mean of the distribution, which is significantly different from zero. (B) Change in psychometric function threshold and slope due to low-frequency optical stimulation when the monkey was attending in to the site of optical stimulation. Each point represents one behavioral session. Changes are expressed as a difference between stimulation and baseline conditions (normalized by baseline). The color code represents whether the changes is threshold and slope were significant in each behavioral session: red = both threshold and slope changes were significant; green = only threshold change was significant; blue = only slope change was significant; black = neither were significant.
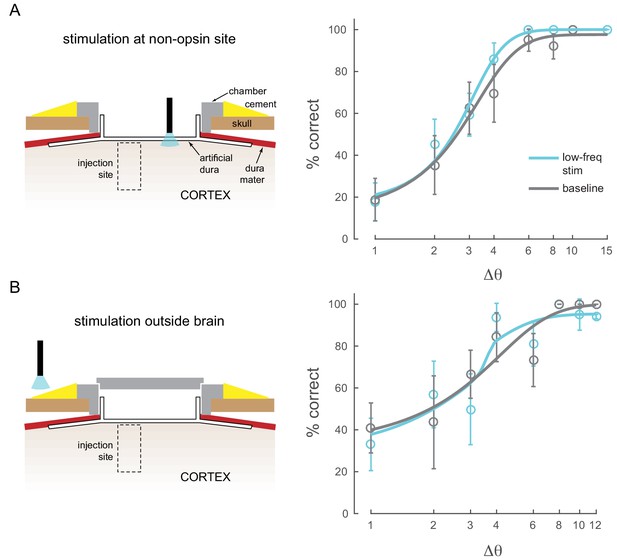
Other control conditions.
(A) Stimulation at a non-opsin site does not perturb behavior. (B) Stimulation with optical fiber outside the brain (with the opto-physiology chamber closed) does not perturb behavior.
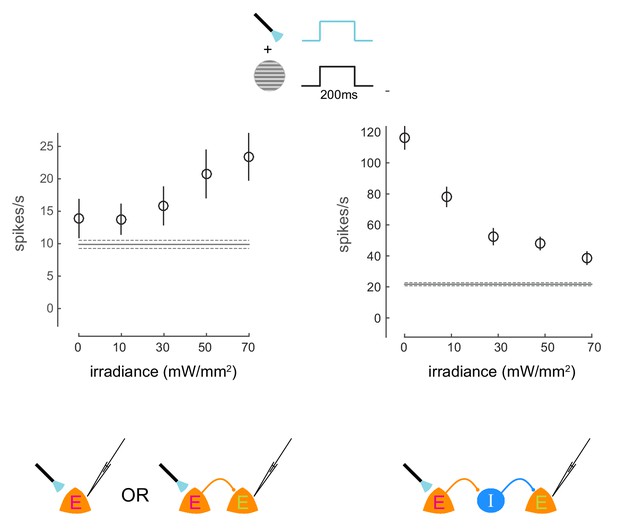
Irradiance response curves.
Response of two neurons to presentations of a visual stimulus and simultaneous optical stimulation. The visual stimulus was a 20% contrast Gabor stimulus presented for 200ms. The optical stimulation consisted of a concurrent light pulse of various irradiance values (x-axis). The first unit exhibits increase in firing rate with increasing intensity of optical stimulation. The second unit exhibits decrease in firing rate with increasing intensity of optical stimulation, due to indirect network effects. Schematics at the bottom illustrate possible network scenarios. E=excitatory neuron (magenta E = opsin expressing; green E = non-opsin expressing). I=inhibitory neuron. The horizontal line represents baseline firing-rate. Mean +/- s.e.m. in all plots.
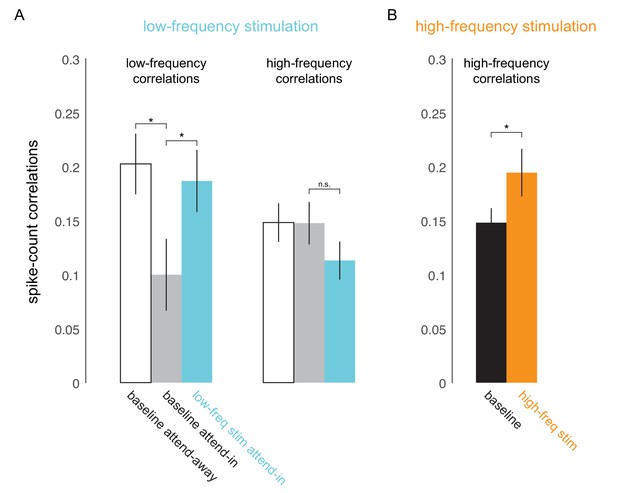
Optical stimulation at low- and high-frequencies induces low- and high-frequency correlated activity.
(A) Consistent with earlier reports (Mitchell et al., 2009), attention reduces baseline spike-count correlations at low frequencies (200ms counting window, ; left panel, white versus gray bar) but not at high frequencies (50ms window; right panel, white versus gray bar). Low-frequency optical stimulation increases low-frequency correlations (; left panel, gray versus blue bar) but not high-frequency correlations (; right panel, gray versus blue bar). (B) High-frequency optical stimulation increases high-frequency correlations (). pairs for baseline and low-frequency stimulation, pairs for high-frequency stimulation, collapsed across attention conditions. Mean +/- s.e.m. in all plots.
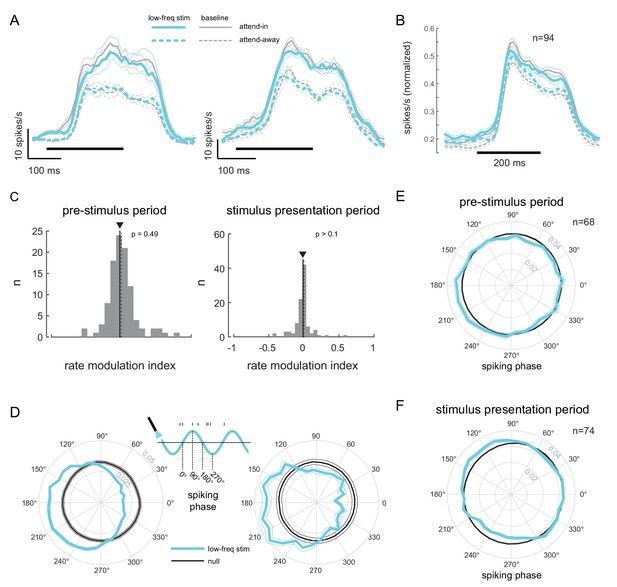
Low-frequency stimulation induces phase-locking without increasing firing rates.
(A) Peri-stimulus time histograms (PSTH) of two example units for the different experimental conditions. Both units show a robust firing rate modulation due to attention (solid versus dashed lines) but no rate increase due to low-frequency optical stimulation (blue versus gray lines). Horizontal bars represent stimulus duration. (B) Population data showing the same rate increase due to attention, but no significant increase due to optical stimulation (). Same convention as in (A). (C) Distribution of rate modulation indices for the low-frequency stimulation attend-in condition compared to the baseline attend-in condition for a 200ms pre-stimulus period (left panel) and 200ms stimulus presentation period (60-260ms after stimulus onset; right panel). The arrowheads depict the median of the distributions. Neither distribution is significantly different from zero (). (D) Phase plots for two example units showing the distribution of spiking activity with respect to the phase of the optical stimulation, during the pre-stimulus period. In gray is the null distribution obtained from a rate-matched Poisson process. Both units show significant deviations from the null distribution ( for both, Rayleigh test), indicative of phase locking. (E) Population phase-locking plot illustrating the bias in spiking activity to the downswing of optical stimulation during the pre-stimulus period (). Same convention as in D). The distribution of spiking phase is significantly different from null (, Rayleigh test). (F) Same as in (E), but for the stimulus presentation period (). The distribution of spiking phase is significantly different from null (, Rayleigh test).
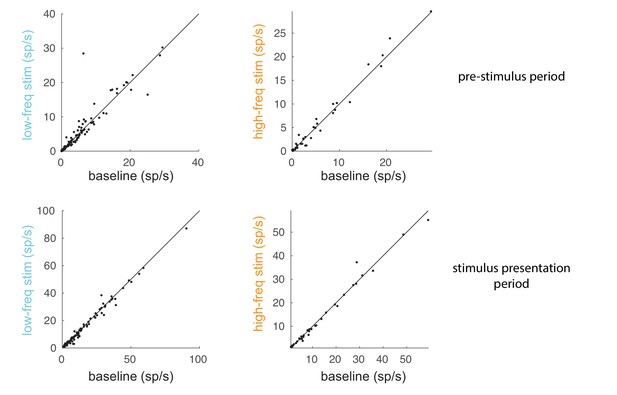
Comparison of spike rates between baseline and optical stimulation.
Comparison of spike rates between the optical stimulation conditions (low-frequency: left panels; high-frequency: right panels) and the baseline condition for a 200 ms pre-stimulus period (top panels) and 200 ms stimulus presentation period (60–260 ms after stimulus onset; bottom panels). Each dot represents a unit in the population of recorded units.
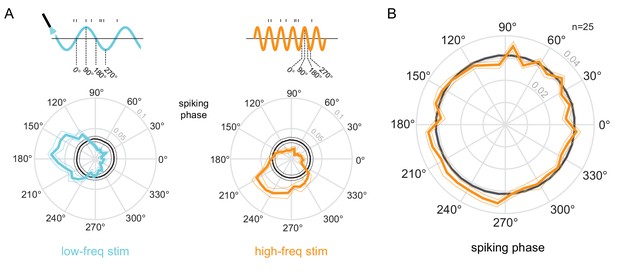
Phase-locking to optical stimulation.
(A) Phase plots for an example unit showing the distribution of spiking activity with respect to the phase of the low-frequency (5Hz, left) and high-frequency (20Hz, right) optical stimulation. In gray is the null distribution obtained from a rate-matched Poisson process. The unit shows significant deviations from the null distribution, indicative of phase locking to both stimulations ( for both stimulation conditions, Rayleigh test). (B) Population phase-locking plot illustrating the bias in spiking activity to the high-frequency optical stimulation (). Same convention as in (A).
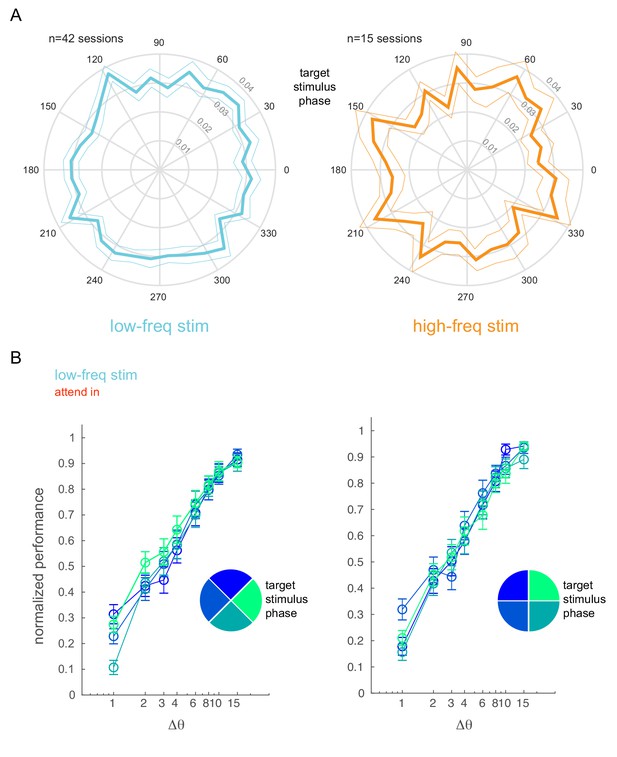
Behavioral performance is not affected by optical stimulation phase.
(A) Phase plots showing the distribution of target stimulus onset times with respect to the phase of the low-frequency (5 Hz, left) and high-frequency (20 Hz, right) optical stimulation. Mean ± s.e.m. (B) Normalized performance (pooled across all behavioral sessions) as a function of target stimulus phase. The target stimulus onset times with respect to the phase of the low-frequency stimulation is binned into four phase bins (legend). The panels show two different bin arrangements.
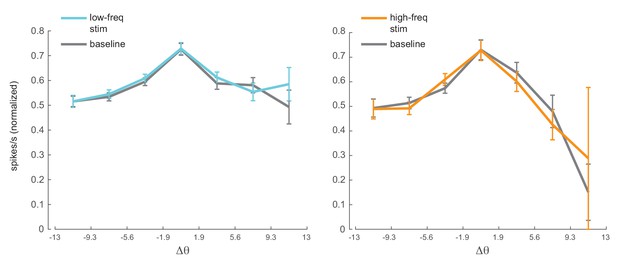
Optical stimulation does not change orientation tuning.
Orientation tuning curves for the neuronal population with and without optical stimulation (left panel, low-frequency stimulation condition, units; right panel, high-frequency stimulation condition, units). The spike rates were normalized to peak response across stimulation conditions (optical stimulation and baseline no-stimulation conditions). The population tuning curves were plotted after aligning the peak response of each neuron to zero and binning the data into orientation bins. The x-axis shows the edges of the orientation bins. Mean +/- s.e.m.
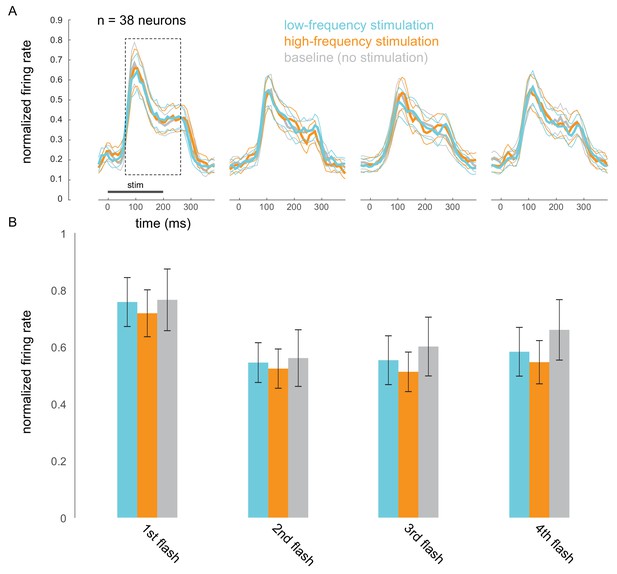
Optical stimulation does not cause frequency-specific adaptation.
(A) Responses evoked by the first four non-target stimuli, for trials in which attention was cued to the stimuli appearing at the opsin site. Responses were averaged and normalized for each neuron to the maximum response across all stimulus positions. Normalized responses were then averaged across 38 neurons recorded in 15 sessions. (B) The corresponding average firing rates over the 200 ms stimulus-evoked period indicated by the box in A (60–260 ms post stimulus onset).
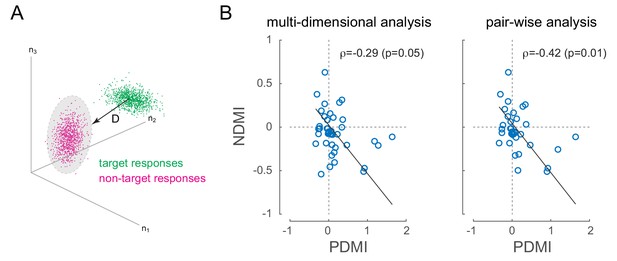
Optical modulation of neural discriminability correlates with behavioral perturbations.
(A) Schematic of neural discriminability analysis. The responses of a hypothetical set of 3 neurons to target (green) and non-target (magenta) stimuli are depicted as point clouds. Each dot represents a stimulus presentation. The discriminability (D) between the two response categories is defined as the Mahalanobis distance between the centroid of the target responses and the non-target point cloud. (B) Neural Discriminability Modulation Index (NDMI) due to optical stimulation is plotted against the corresponding PDMI (behavioral threshold change) for each experimental session. NDMI is calculated either from multi-dimensional clouds from all simultaneously recorded neurons (left panel; n = 42 sessions) or as the average of two-dimensional clouds from all pairs of simultaneously recorded neurons (right panel; n = 35 sessions). NDMI is negatively correlated with PDMI. Since both NDMI and PDMI are dependent measures, the data were fitted with a line whose slope was obtained from a Model II regression.
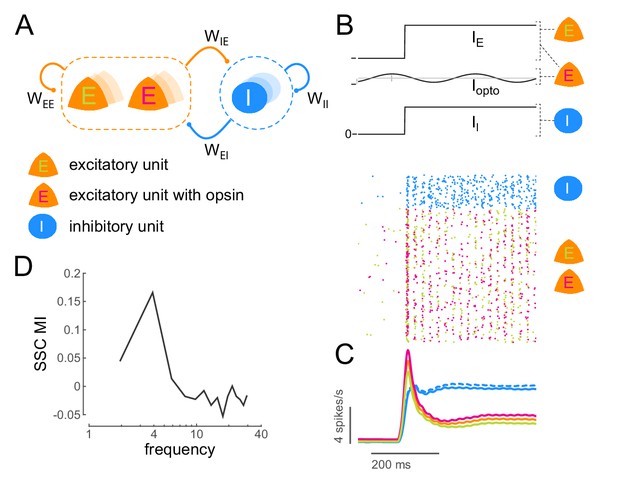
Low-frequency sub-threshold stimulation induces coherent activity in a computational model of E-I neurons.
(A) Schematic of a local conductance-based E-I network with mutually coupled excitatory (E) and inhibitory (I) units. A fraction (50%) of the E units are sensitive to 'optical' stimulation. , self-excitation among E units;, self-inhibition among I units;, excitation provided by E to I; , inhibition provided by I to E. (B) Simulation of a network of 800 E and 200 I units (). The raster plot shows the activity of all units in the model (blue, I; green, E without opsin; magenta, E with opsin) to a step input () and 4Hz sinusoidal optical stimulation (). (C) Population spiking rate averaged across 1000 simulations of the scenario in (B) with and without optical stimulation. (blue, I; orange, all E; green, E without opsin; magenta, E with opsin. solid lines, with optical stimulation; dashed lines, without optical stimulation) (D) Spike- spike coherence (SSC) among E units was calculated for the two conditions with and without optical stimulation and the change in SSC across the two conditions was calculated as a modulation index (SSC MI). SSC MI exhibits a peak at 4Hz due to optical stimulation.
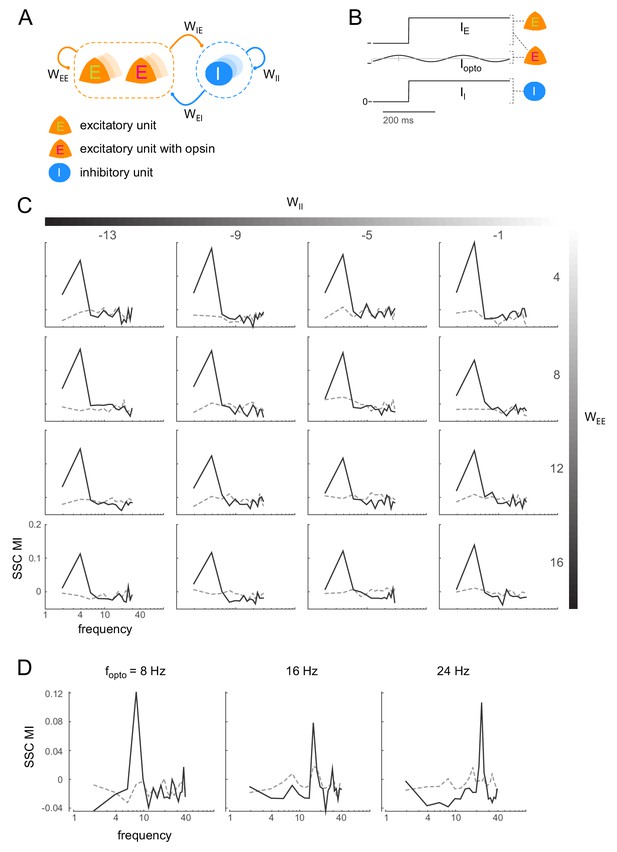
Induction of coherent activity in the E-I model is robust across network and stimulation parameters - I.
(A)-(B) same as Figure 6A-B. (C) Spike-spike coherence modulation index (SSC MI; see Figure 6D) as a function of varying the self-coupling parameters and , keeping the other two parameters fixed (). SSC MI exhibits a peak at the 4Hz optical stimulation frequency irrespective of the self-coupling parameters. The dotted lines represent the null SSC MI obtained by shuffling trial identities. (D) The peak SSC MI shifts with increasing optical stimulation frequency (, network parameters same as in Figure 6), indicating that the peak is not due to any intrinsic resonant activity in the network. Dotted lines same as in (C).
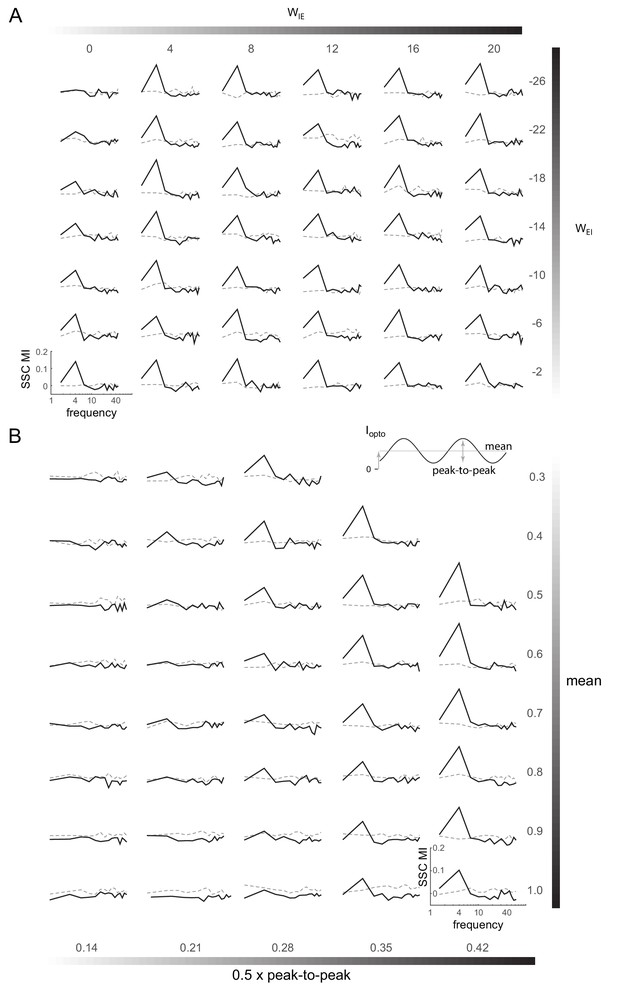
Induction of coherent activity in the E-I model is robust across network and stimulation parameters - II.
(A) Spike-spike coherence modulation index (SSC MI; see Figure 6D) as a function of varying the cross-coupling parameters and , keeping the other two parameters fixed (). (B) SSC MI as a function of the mean and peak-to-peak variation of the 4Hz sinusoidal optical stimulation (). Network parameters same as in Figure 6.
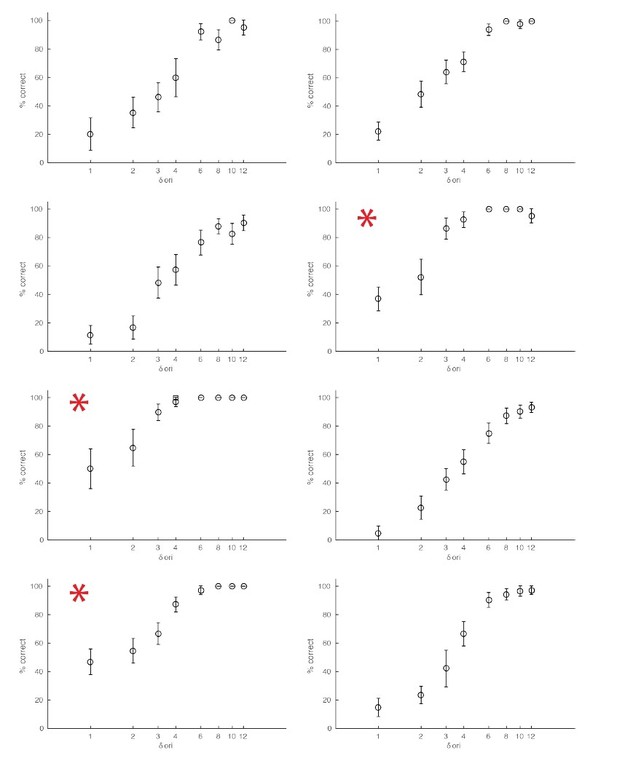
Examples of behavioral sessions without optogenetic stimulation.
The red asterisks mark behavioral sessions where the baseline orientation (orientation of the non-target stimuli) was either horizontal or vertical.
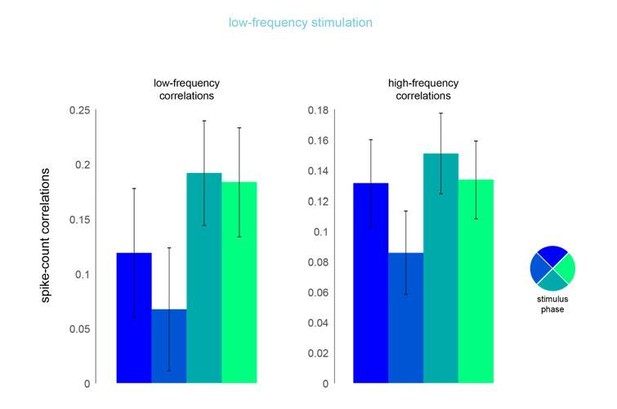
Phase dependence of spike-count correlations for both low- (left panel, 200ms counting window) and high-frequency correlations (right pane, 50ms counting window) for the low-frequency optical stimulation condition.
Additional files
-
Transparent reporting form
- https://doi.org/10.7554/eLife.35123.020