Clusters of cooperative ion channels enable a membrane-potential-based mechanism for short-term memory
Figures
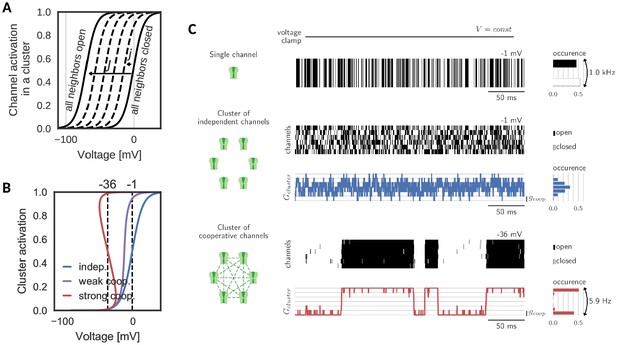
Gating of a cluster of cooperative channels.
(A) Model of cooperative gating: the activation of a channel in a cluster depends on the state of the surrounding channels. Opening of a neighboring channel leads to a shift of the activation curve by the coupling strength . When all neighbors are closed, the activation coincides with the one of an isolated channel, whereas when all neighbors are open, the activation undergoes the maximal shift . (B) Cluster activation: cooperative interactions inside a cluster increase the channel activity, so that the activation curve becomes steeper (weak coop.). Above a critical coupling strength, the activation starts to ‘bend over’ (strong coop.); whether a channel is open or closed is determined mostly by the state of its surrounding channels. Activation curve based on a self-consistency relation, for details see Materials and methods. (C) Top: Simulation of a voltage clamp experiment with an isolated two-state channel shows fast, random switching between the conducting (black) and non-conducting (white) state. Operated at the half-activation potential , the channel spends about half of the time in the open state. Middle: In a cluster of independent channels, their asynchronous gating results in a fluctuation of the cluster conductance around half of the total conductance. Bottom: Cooperative channels have a strong preference for the state of the surrounding channels. In a cluster, they open and close in synchrony acting like a macrochannel. Its slow switching frequency demonstrates the stability of the open and closed cluster state. As the cooperative channels are more active compared to the independent ones, they are clamped at more hyperpolarized voltages: −1 mV (independent) and 36 mV (cooperative). The details of the jump process simulation are given in the section Materials and methods and parameters are summarized in Table 1.
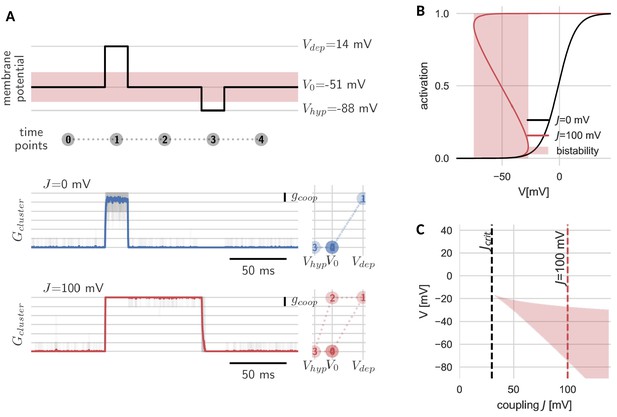
Hysteresis and bistability of a cluster of cooperative channels.
(A) Probing an initially closed cluster (time point 0), both independent (blue) and cooperative (red) channels open in response to a depolarizing voltage pulse (1). However, returning to the base line voltage (2), independent channels rapidly close again, whereas a cluster of cooperative channels ‘sticks’ to the open configuration. Only a strong hyperpolarizing pulse (3) can close the cooperative channels and restore the initial condition (4). Tracking membrane potential versus conductance during the pulse protocol (right panels), the hysteresis in gating for the cooperative channels becomes apparent; whether the cluster is open or closed at baseline voltage depends on the previous voltage pulse. The transparent lines correspond to 20 repetitions of the pulse protocol and the solid lines are the respective means. (B) Strong coupling among channels changes the activation in a cluster (red) with respect to an isolated channel (black) and induces a voltage regime of bistability (red shaded). (C) Clusters only become bistable beyond a critical coupling strength (black dashed) and for increasing coupling, the bistable range extends to lower voltages. Cluster and channel parameters are summarized in Table 1.
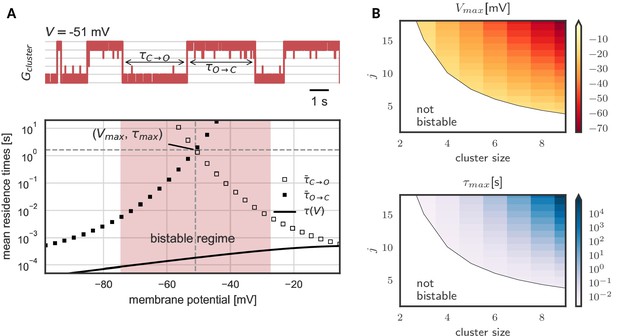
Prolonged life times of cluster states in the bistable regime.
(A) Top: Voltage-clamped in the bistable regime, a cluster of five cooperative channels can stay in the open or closed state for multiple seconds until it spontaneously switches. The noise source are spontaneous single channel gating events, visible as the fast fluctuations around the stable states. Bottom: Entering the bistable regime, the residence times in the open and closed state increase over multiple orders of magnitude. The point of maximal stability is located at the center; here, a cluster is expected to stay open or closed for seconds, exceeding by far the single channel time constant (black line). Mean life times are derived from a first passage analysis, see Materials and methods. (B) Stability of the open and closed cluster configuration depend on the cluster size and the intra channel coupling . Larger clusters with stronger intra-channel coupling can be stable for multiple seconds and their bistable range moves to more hyperpolarized voltages. Note that small clusters with weak coupling are not bistable (white region). Cluster and channel parameters are summarized in Table 1.
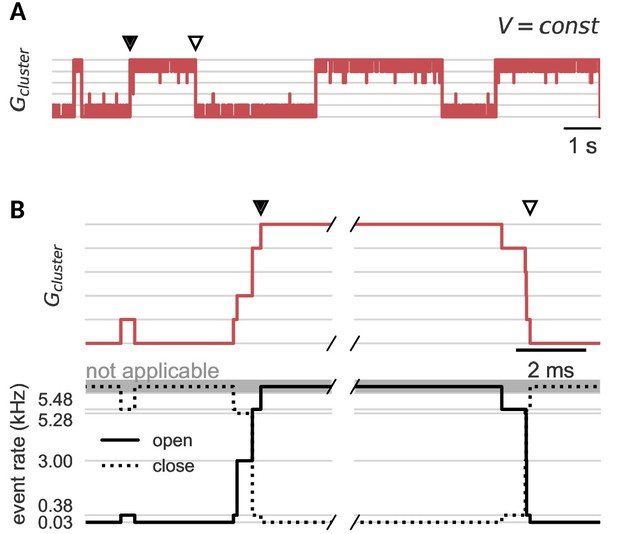
Channel noise can open and close a cluster.
(A) Clamped at a fixed voltage in the bistable regime, a cluster of cooperative channels slowly switches between the open and closed state. In both cases, when a cluster opens (black dot) or closes (white dot), this quasi-synchronous gating of all channels is triggered by channel noise and amplified by cooperativity. (B) Zoom on the exemplary opening (left) and closing (right) events in A. The cooperative interactions spread single channel switches by changing the rates for the next opening (solid) or closing (dashed) event. Either channels open and facilitate further openings or channels close and decrease the facilitation of their neighbors. Note that the cluster is stable against single channel gating events; when only one channel opens, the increased opening rate (0.03 kHz to 0.38 kHz) is still ten-fold smaller than the closing rate (5.48 kHz). Cluster parameters are summarized in Table 1.
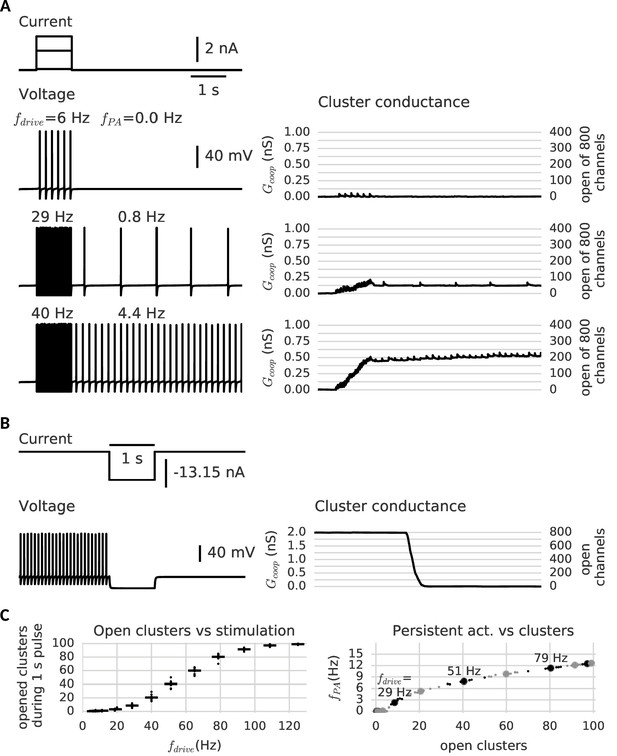
Clusters of cooperative channels mediate persistent activity at different levels in a model neuron.
(A) Current stimulation with different amplitudes to test the response of clusters to different firing rates. Left: Voltage traces demonstrating stimulated firing at increasing frequencies. Whereas the neuron returns to rest after a low amplitude stimulation, after a stronger drive the neuron continues to spike at a stable frequency , which increases with the frequency of the stimulated firing . Right: Persistent activity is mediated by the conductance of the clusters, which builds up during high-frequency spiking and remains stable during low-frequency spiking and at rest. (B) A strong hyperpolarizing step closes the clusters and stops persistent firing. (C) Left: The cooperative clusters track the input strength; more clusters open when the cell fires at higher frequencies. Firing below 20 Hz, however, does not open clusters. The number of open clusters can differ across trials (black dots) because of stochastic channel gating. Right: Persistent activity increases with the number of open clusters, thereby allowing the neuron to represent the input strength after the stimulus has ended, for example 3 Hz after 29 Hz firing and 9 Hz after 51 Hz firing. Big dots denote the mean for each stimulation strength, small dots the individual trials. The simulation procedure is described in Materials and methods and parameters are summarized in Table 1.
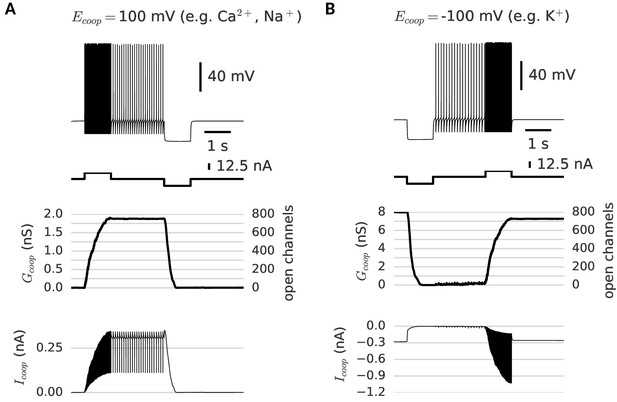
Depending on their reversal potential, clusters of cooperative channels can mediate de- or hyperpolarization-activated persistent activity.
(A) Depolarization-activated persistent activity. After stimulated spiking, the neuron continues to spike, because of the depolarizing current (=100 mV) through the persistently open clusters (bottom trace). (B) Hyperpolarization-activated persistent activity. A very distinct mnemonic firing behavior is possible with clusters of cooperative channels that have the same dynamics as the ones in A, but conduct a hyperpolarizing current (=-100mV). When such clusters are open initially, they provide a standing leak current that prevents the neuron from firing (bottom trace). A strong hyperpolarizing pulse persistently closes the clusters and thereby activates low-frequency spiking. Strong stimulated spiking reopens the clusters and hence silences the neuron. For a summary of cluster parameters, see Table 1.
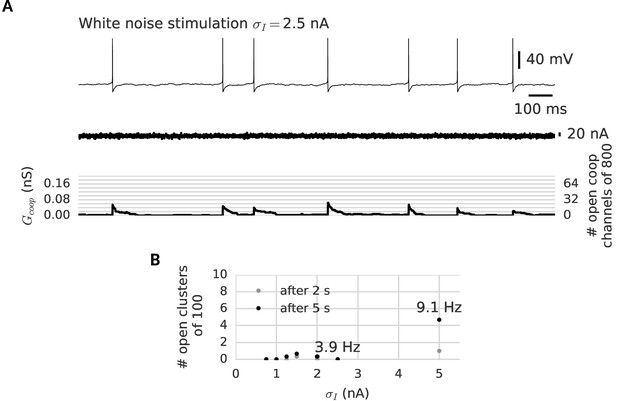
Clusters are robust against noise in the membrane potential.
(A) A neuron is stimulated for 2 s with a white noise current (middle) to induce membrane potential fluctuations and spontaneous spikes (top). Despite the noisy membrane potential, the clusters remain in their closed state (bottom). Even spontaneous spikes only open a few channels transiently in each cluster and cannot switch a whole cluster persistently to the open state (B) The clusters remain closed after exposure to 5 s of noise stimulation. Only an unphysiologically high noise level leads to the opening a few clusters by spontaneous firing at around 10 Hz (see indicated firing frequencies). For details on the white noise stimulation see Materials and methods. There are 100 clusters of eight cooperative channels each. Further parameters are summarized in Table 1.
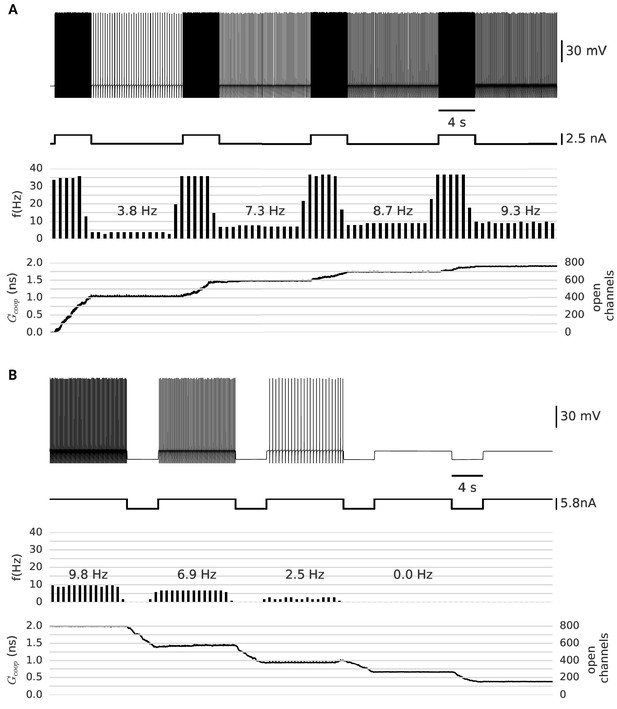
Clusters of strongly cooperative ion channels mediate graded persistent activity in a simple neuron model.
(A) Repeated stimulation drives fast spiking in the neuron, which is followed by self-sustained, stable low-frequency activity at increasing rates (top). With each pulse, the driven, fast spiking switches more clusters to the open state and adds further stable conductances (bottom). The built-up conductance allows a persistent current to flow and sustains activity beyond stimulation. (B) In the same manner, strong hyperpolarization closes the clusters and allows to reduce the frequency of persistent activity until finally the cells stops firing. The simulation procedure is described in Materials and methods and parameters are summarized in Table 1.
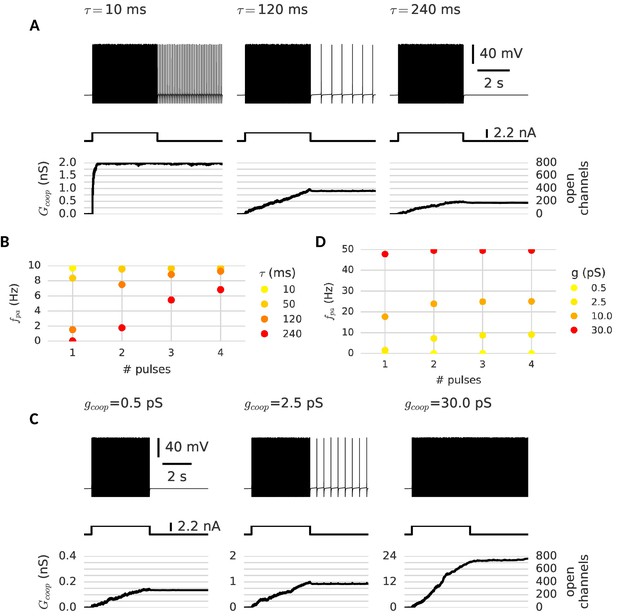
Memory dynamics with different properties of cooperative channels.
The channel’s time constant and their conductance change the memory dynamics of the neuron probed with the multi-pulse protocol for graded persistent activity. (A) Variation of the time constant: When channels are fast (left, τ = 10 ms), the neuron is already maximally active after the first pulse - a few spikes suffice to open all clusters and there is only one level of persistent activity. Slower channels allow for a graded opening of channels and intermediate levels of persistent activity (middle, τ = 120 ms). If the channels are too slow and too few clusters open during the first pulse, the neuron remains silent (right, τ = 240 ms). (B) Frequency of persistent activity after each pulse for different time constants. (C) Variation of the channel conductance: When channels are low conducting, the persistent current remains subthreshold and is thus insufficient to drive persistent activity (left). At an intermediate conductance, the clusters mediate low frequency persistent activity (middle). When the provided conductance is too large, the high frequency persistent activity can open further clusters and is no longer stable. (D) Frequency of persistent activity after each pulse for different channel conductances. Other cluster parameters are summarized in Table 1.
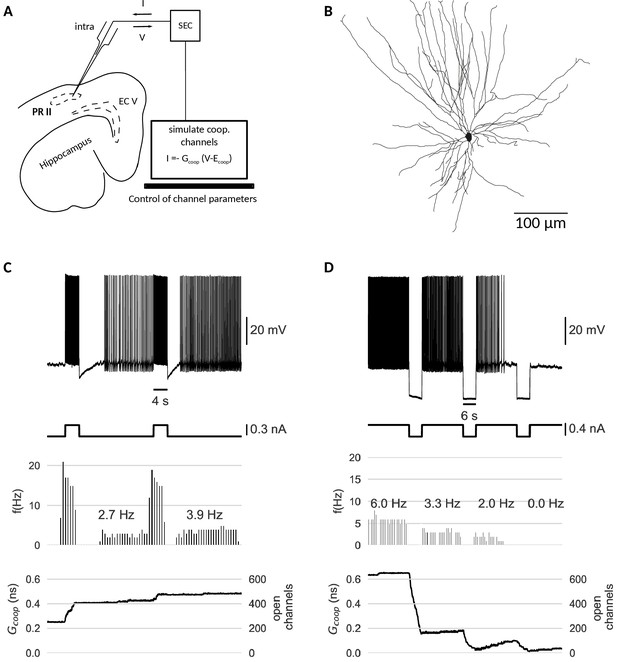
Dynamic clamp experiment.
(A) Intracellular recording of a perirhinal cortex neuron in dynamic clamp mode. The computer simulates the state of clusters of cooperative channels given the measured membrane potentials and emulates the effect of their conductance to the neuron’s activity by inserting a corresponding current. (B) Neurolucida drawing of the recorded neuron. (C) Graded persistent activity of the recorded neuron mediated by the multi stable conductance of the clusters of cooperative channels. (D) Hyperpolarization brings the cell back to rest via intermediate levels of persistent firing. In control recordings with identical, yet- independent channels, no persistent firing was observed (Figure 6—figure supplement 1). Slice preparation, electrophysiological setup and the dynamic clamp emulation of the clusters are described in Material and methods. Cluster parameters are summarized in Table 1.
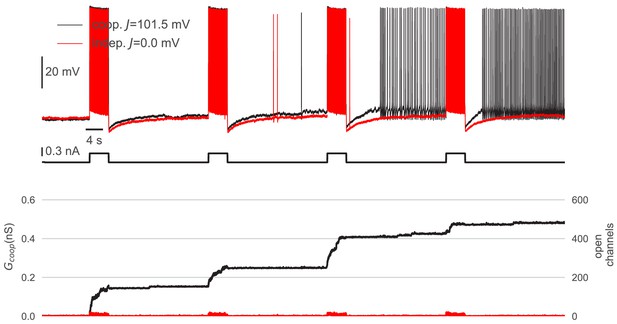
Cooperative interactions are necessary to mediate graded persistent activity.
Control experiment to demonstrate the requirement of cooperative channel interactions for graded persistent activity in a perirhinal cortex neuron. Top: When the channels gated independently (red, J=0 mV), the neuron only fired during the stimulating pulses and otherwise remained silent. When, in contrast, the channels cooperate strongly (black, J=101.5 mV), the cell produced graded persistent activity (this trace is the full trace of the extract shown in Figure 6). Bottom: The independent channels reacted to spiking activity only in a transient way and remained closed after the stimulus. In contrast, cooperative channels opened and facilitated their neighbors to open as well. In this way, spiking could open whole clusters, which remained open after the stimulus and drove persistent activity. A detailed description of the dynamic clamp experiment is given in Material and methods. Cluster parameters are summarized in Table 1.
Tables
Cluster and cooperative channel parameters.
: number of clusters, : cluster size, : overall coupling, : channel-channel coupling, : half activation, : width of the channel activation, : time constant of the channel with maximum at attained at and width , : single channel conductance and : reversal potential.
Figure | k | gcoop | Ecoop | ||||||||
---|---|---|---|---|---|---|---|---|---|---|---|
mV | mV | mV | mV | ms | mV | mV | pS | mV | |||
Figure 1 (independent) | 1 | 6 | 0 | 0 | −1 | 15 | 0.5 | −1 | 30 | - | - |
Figure 1 (weak coop.) | 1 | 6 | 22.5 | 4.5 | −1 | 15 | 0.5 | −1 | 30 | - | - |
Figure 1 (strong coop.) | 1 | 6 | 70 | 14 | −1 | 15 | 0.5 | −1 | 30 | - | - |
Figure 2 (independent) | 1 | 6 | 0 | 0 | −1 | 15 | 0.5 | −1 | 30 | - | - |
Figure 2 (cooperative) | 1 | 6 | 100 | 20 | −1 | 15 | 0.5 | −1 | 30 | - | - |
Figure 3 | 1 | 5 | 100 | 25 | −1 | 15 | 0.5 | −1 | 30 | - | - |
Figure 4 | 100 | 8 | 80 | 11.4 | −30 | 10 | 120 | −30 | 20 | 2.5 | 100 |
Figure 5 | 100 | 8 | 80 | 11.4 | −30 | 10 | 120 | −30 | 20 | 2.5 | 100 |
Figure 6c | 90 | 8 | 101.5 | 14.5 | −10 | 15 | 100 | −10 | 30 | 1 | 100 |
Figure 3—figure supplement 1 | 1 | 5 | 100 | 25 | −1 | 15 | 0.5 | −1 | 30 | - | - |
Figure 4—figure supplement 1A | 100 | 8 | 80 | 11.4 | −30 | 10 | 120 | −30 | 20 | 2.5 | 100 |
Figure 4—figure supplement 1B | 100 | 8 | 80 | 11.4 | −30 | 10 | 120 | −30 | 20 | 10 | −100 |
Figure 4—figure supplement 2 | 100 | 8 | 80 | 11.4 | −30 | 10 | 120 | −30 | 20 | 2.5 | 100 |
Figure 5—figure supplement 1A,B | 100 | 8 | 80 | 11.4 | −30 | 10 | 10–240 | −30 | 20 | 2.5 | 100 |
Figure 5—figure supplement 1C,D | 100 | 8 | 80 | 11.4 | −30 | 10 | 120 | −30 | 20 | 0.5–10 | 100 |
Figure 6—figure supplement 1 (independent channels, red) | 90 | 8 | 0 | 0 | −10 | 15 | 100 | −10 | 30 | 1 | 100 |
Figure 6—figure supplement 1 (cooperative channels, black) | 90 | 8 | 101.5 | 14.5 | −10 | 15 | 100 | −10 | 30 | 1 | 100 |