Proteome dynamics during homeostatic scaling in cultured neurons
Figures
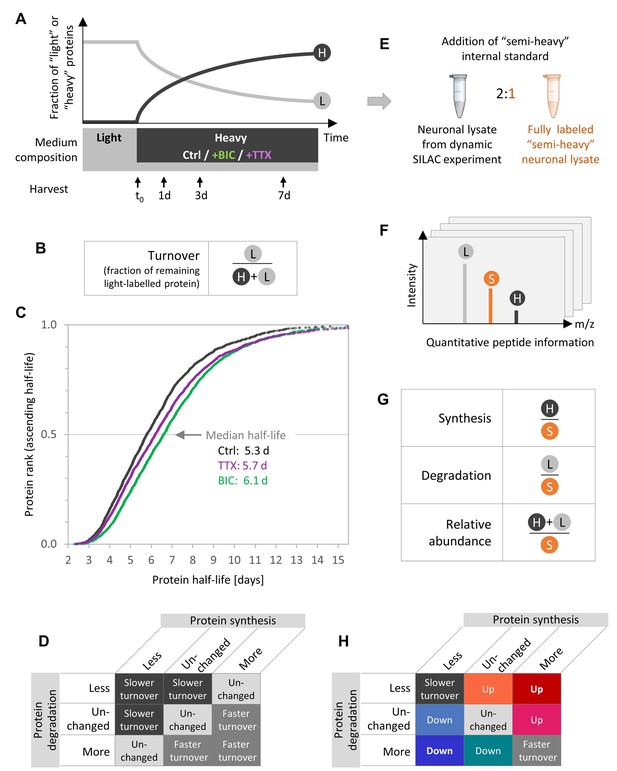
Experimental design to quantify protein synthesis and degradation during homeostatic scaling.
(A) Dynamic SILAC experiment. Primary hippocampal cells were grown for 18–19 days in a medium containing natural amino acids (light) and then switched to a medium containing heavy isotopically labeled arginine and lysine together with BIC or TTX or without a treatment (Ctrl). Upon the medium change, heavy amino acids were incorporated into nascent proteins, whereas the fraction of light pre-existing proteins decayed over time. The cells were harvested 1, 3 and 7 days after the medium change as well as just before the medium change (t0). (B) Protein turnover rates are estimated using the fraction of remaining pre-existing protein signal (light [L]) relative to the total protein signal (heavy and light [H+L]). (C) Calculated protein half-lives in primary hippocampal cultures under basal neuronal activity (Ctrl, black), during BIC-induced homeostatic down-scaling (green) and during TTX-induced homeostatic up-scaling (violet). Protein half-lives for the different treatments are displayed in ascending order and the median half-life for each treatment is indicated. N = 3 biological replicates. Protein half-life distributions are significantly different for BIC- and TTX-treated neurons than for untreated controls (Mann-Whitney test, p<0.001). (D) Combinations of possible changes in protein synthesis and degradation rates and resulting changes in calculated half-lives. (E) To enable accurate and independent quantification of ‘light’ and ‘heavy’ signals, a fully ‘semi-heavy’-labeled neuronal cell lysate was spiked into each sample to serve as an internal standard (IS). (F) Schematic MS1 spectrum for a dynamic SILAC sample with IS spike-in. For each condition and each time point, each peptide shows three signals, the light (L) pre-existing peptide, the heavy (H) new peptide and the semi-heavy (S) IS peptide. (G) Quantification of protein synthesis, degradation and abundance. Protein synthesis is quantified using the heavy (H) signal normalized to the semi-heavy (S) IS, whereas protein degradation is assessed using the light (L) signal of pre-existing proteins normalized to the IS and protein abundance is quantified using the sum of light and heavy signal normalized to the IS. (H) Combinations of possible changes in protein synthesis and degradation rates and resulting changes in protein turnover or protein abundance.
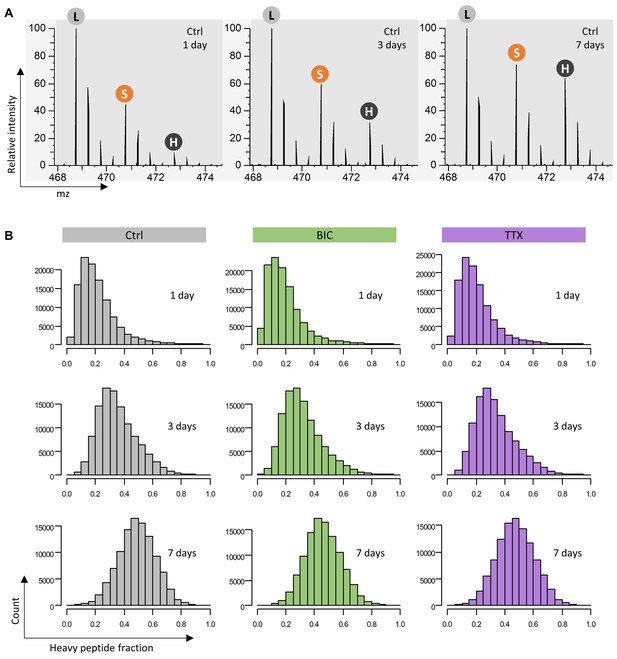
Heavy amino acid incorporation.
(A) Exemplary MS1 spectra of the peptide ‘ILGDYDIK’ (tryptic product of protein Synapsin-2) after 1, 3 and 7 days of heavy SILAC pulse. The monoisotopic peaks of the light (L), semi-heavy (S) and heavy (H) isotopologues are indicated. (B) Distributions of heavy peptide fractions for each time point and treatment condition.
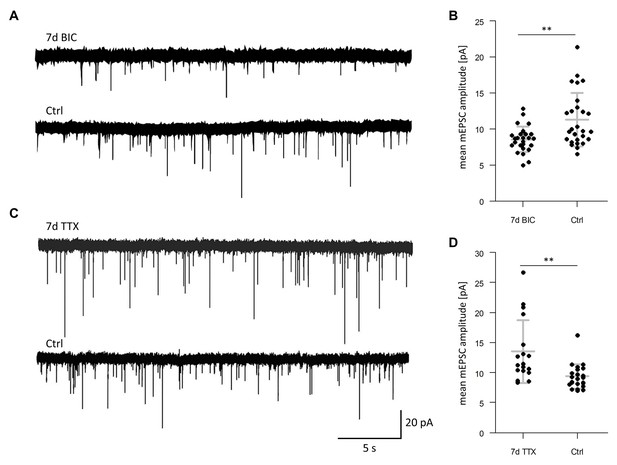
Electrophysiological recordings.
(A) Representative electrophysiological recordings of miniature excitatory postsynaptic currents (mEPSCs) from hippocampal cultures treated with BIC for 7 days or from untreated controls (Ctrl). (B) Analysis of mEPSC amplitude for groups, as indicated. N = 26 cells for BIC and N = 27 cells for Ctrl, each from seven biological replicates. BIC-treated neurons exhibited significantly decreased mEPSCs amplitudes compared to control neurons (unpaired t-test, p=0.009). (C) Representative electrophysiological recordings of mEPSCs from hippocampal cultures treated with TTX for 7 days or from untreated controls. (D) Analysis of mEPSC amplitude for groups, as indicated. N = 18 cells for TTX and N = 24 cells for Ctrl, each from six biological replicates. TTX-treated neurons exhibited significantly increased mEPSCs amplitudes compared to control neurons (unpaired t-test, p=0.005).
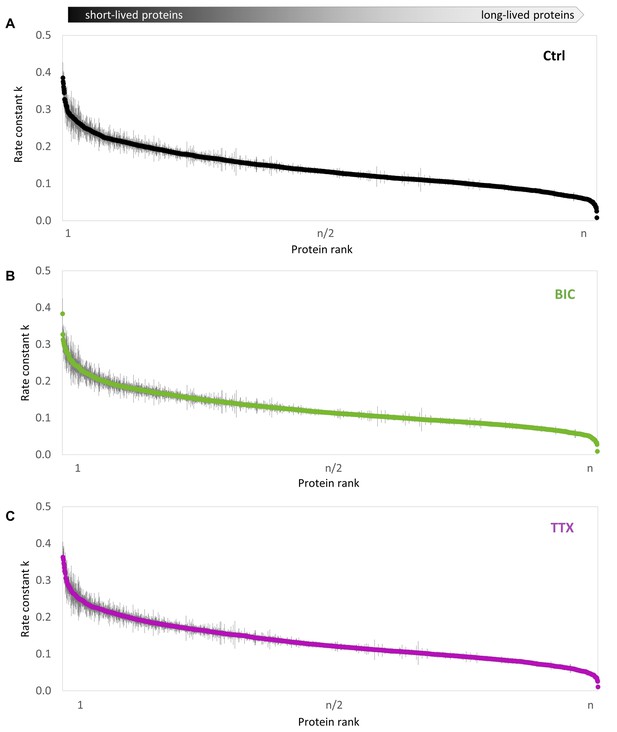
Precision of protein half-life determination.
Protein half-lives were determined using a linear fit of the natural log transformed fraction of pre-existing proteins over time. Rate constants k (negative value of the slope of the fit) and the SE (standard error of the slope) are shown for each protein group for untreated (A), BIC-treated (B) and TTX-treated (C) cultures.
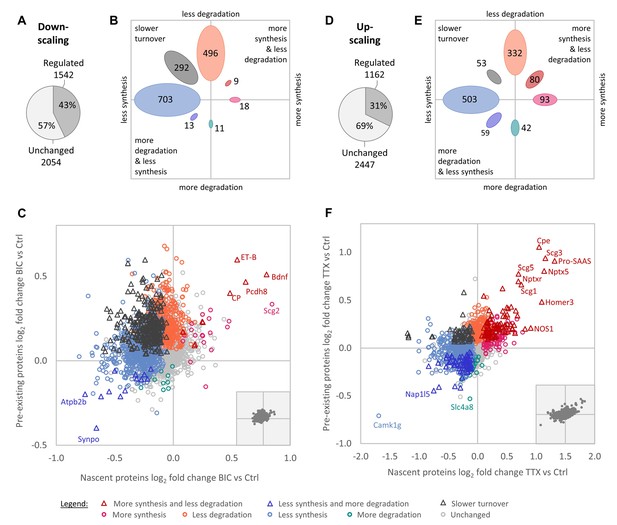
Regulation of protein synthesis and degradation rates during homeostatic scaling.
(A–C) Regulation during BIC-induced homeostatic down-scaling. (A) Numbers of proteins with significantly altered (‘regulated’) synthesis and/or degradation rates. (B) Numbers of proteins that show the indicated types of regulation. (C) Behavior of individual proteins showing changes in protein synthesis (nascent proteins; x-axis) and degradation (pre-existing proteins; y-axis). Significantly regulated proteins (1% false discovery rate [FDR]) are displayed in color and proteins that are not significantly changed are displayed in light gray. Some individual proteins of interest are indicated by name. The inset shows the distribution of all quantified proteins with equal axis dimensions. (D–F) Regulation during TTX-induced homeostatic up-scaling. (D) Numbers of proteins with significantly altered (‘regulated’) synthesis and/or degradation rates. (E) Numbers of proteins that show the indicated types of regulation. (F) Behavior of individual proteins showing changes in protein synthesis (nascent proteins; x-axis) and degradation (pre-existing proteins; y-axis). Significantly regulated proteins (1% FDR) are displayed in color and proteins that are not significantly changed are displayed in light gray. Some individual proteins of interest are indicated by name. The inset shows the distribution of all quantified proteins with equal axis dimensions.
-
Figure 2—source data 1
Protein regulation during homeostatic up- and down-scaling.
- https://cdn.elifesciences.org/articles/52939/elife-52939-fig2-data1-v1.xlsx
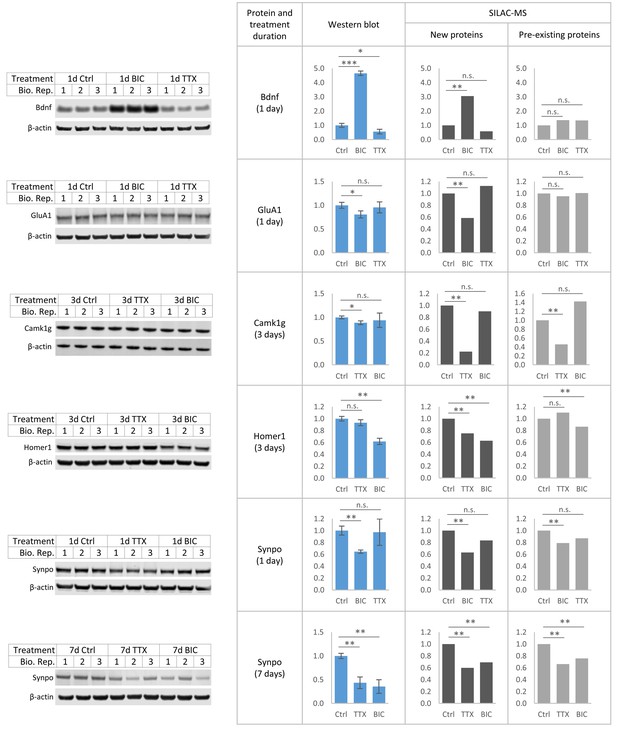
Validation of selected proteins by western blot.
Western blot analysis for Bdnf, GluA1, Camk1g, Homer1 and Synpo, as well as β-actin as loading control. Treatment and treatment duration are indicated. All signals were normalized to the corresponding β-actin signals. For statistical analysis of the western blot data, a two-sided t-test was performed. ***, p<0.001; **, p<0.01; *, p<0.05; n.s., not significant. Error bars represent the standard deviation between biological replicates (N = 3). Quantitative results of the western blot analysis (blue) are in excellent agreement with the SILAC-MS results for new proteins (dark gray) and pre-existing proteins (light gray). For SILAC-MS analysis: **, FDR < 1%; n.s., not significant.
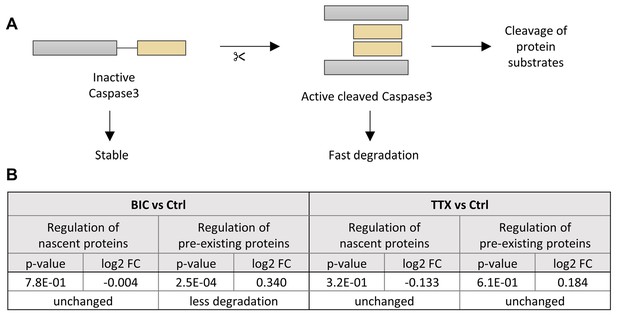
Analysis of Caspase-3 stability.
(A) Upon induction of apoptosis, Caspase-3 is proteolytically cleaved into the small and large subunit. Two copies of each subunit form the active heterotetramer (active cleaved Caspase-3). While inactive Caspase-3 is comparably stable, the active cleaved Caspase-3 is rapidly degraded (Tawa et al., 2004). (B) During homeostatic scaling, no increase in Caspase-3 degradation was observed (analysis of entire time course), indicating that there is no increase in apoptosis.
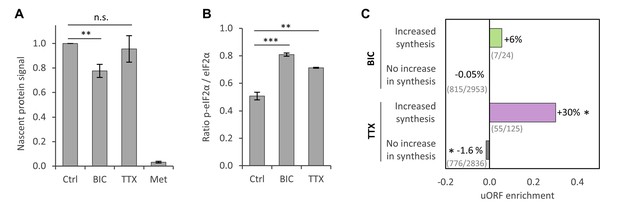
Regulation of protein synthesis during homeostatic scaling.
(A) Quantification of the biotin-derived nascent protein signal via western blot. Error bars represent the standard error of the mean (SEM). N = 5 biological replicates. Protein synthesis was significantly reduced in BIC-treated hippocampal cultures compared to untreated controls (paired t-test, p<0.05), whereas the change in protein synthesis was not statistically significant for TTX-treated hippocampal cultures. (B) Quantification of phosphorylated as well as unmodified eIF2α via western blot. Error bars represent the SEM. N = 3 biological replicates. Phosphorylation of eIF2α at Ser51 was significantly increased after 24 hr of treatment with BIC (unpaired t-test, p-value<0.001) and TTX (unpaired t-test, p-value<0.01). (C) uORF enrichment analysis for the sets of proteins that do or do not show increased protein synthesis during BIC-induced down-scaling or TTX-induced up-scaling. uORF-containing genes are significantly over-represented in the group of proteins that showed increased synthesis during TTX treatment (hypergeometric test, p<0.05) and significantly under-represented in the group of proteins that did not show increased synthesis during TTX treatment (hypergeometric test, p<0.05). Numbers of uORF-containing transcripts per gene subset are shown in brackets. The rat genome was used as reference.
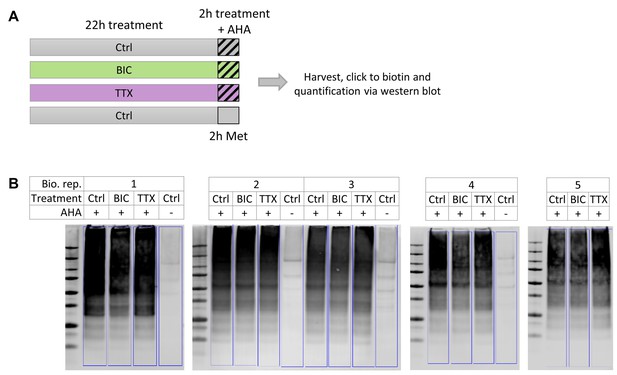
Quantification of nascent proteins via western blot.
(A) Primary hippocampal cultures were treated with BIC, TTX or vehicle (Ctrl) for 24 hr. During the last 2 hr, newly synthesized proteins were labeled with AHA (4 mM). A negative control sample was treated with methionine (Met; 4 mM) instead of AHA. AHA-labeled proteins were covalently bound to biotin via a click reaction and quantified by western blot. (B) Western blots against biotin-tagged nascent proteins.
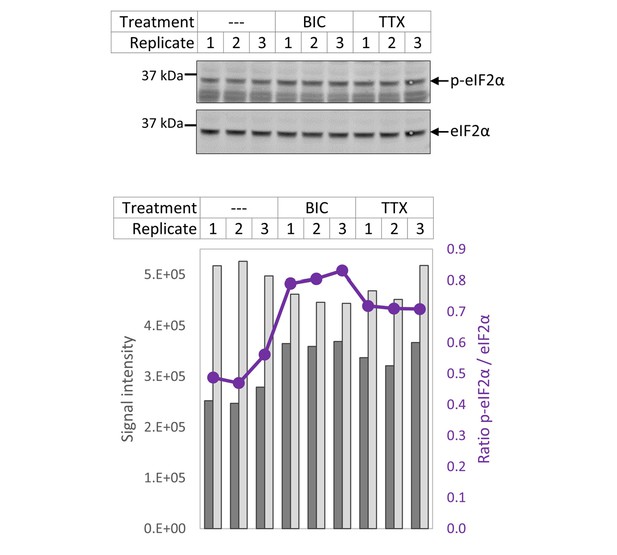
eIF2α phosphorylation.
Quantification of p-eIF2α and unmodified eIF2α via western blot.
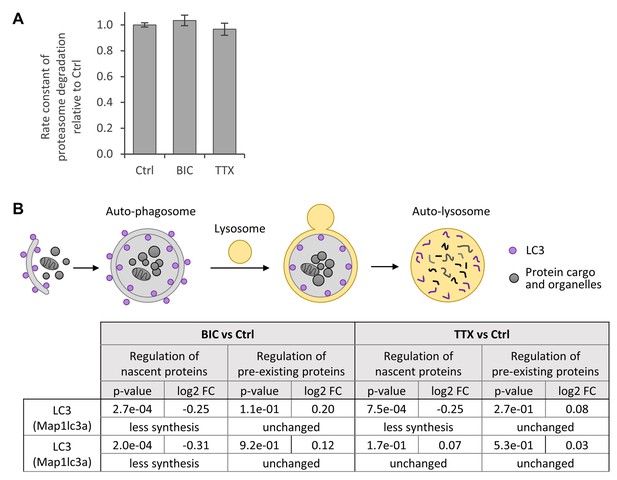
Autophagy and proteasomal degradation.
(A) Proteasome activity during homeostatic scaling. Proteasome activity was measured in the lysates of primary hippocampal cultures that were treated with BIC or TTX for 7 days as well as in the lysates of untreated controls. The bar diagram represents the rate constant of proteasomal degradation and the error bars indicate the SEM. No difference in proteasome activity was observed (unpaired t-test; N = 6 biological replicates). (B) Changes in autophagy during homeostatic scaling. The degradation rate of microtubule-associated protein 1 light chain 3 (LC3) serves as marker for ongoing autophagy (Tanida et al., 2005). LC3-phospholipid conjugates are localized to the membrane of autophagosomes. LC3 inside the autophagosomes is degraded during autophagy together with the protein cargo. No change in LC3 degradation was observed during BIC or TTX treatment (N = 3 biological replicates).
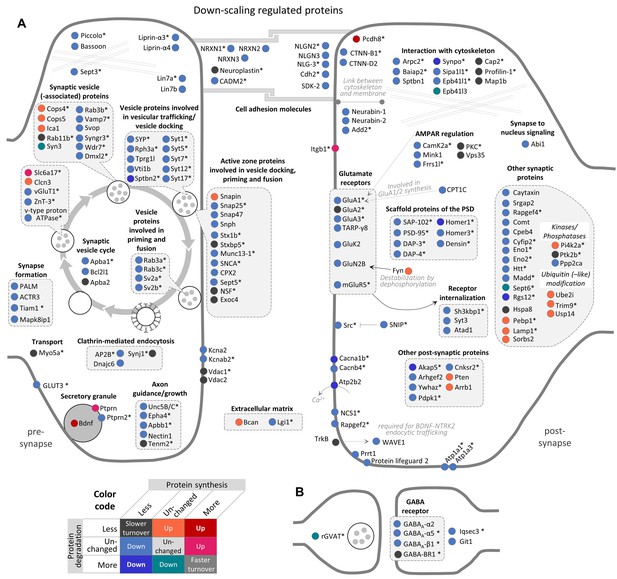
Regulation of synaptic proteins during BIC-induced homeostatic down-scaling.
Down-scaling regulated proteins associated with excitatory glutamatergic synapses (A) or inhibitory GABAergic synapses (B). Changes in protein synthesis and/or degradation are indicated by a color code. ‘Warm’ reddish colors indicate a change in synthesis/degradation that leads to increased protein copy numbers and ‘cold’ blueish colors indicate a change that leads to decreased protein copy numbers. Source data are provided in Figure 4—source data 1. Synaptic proteins that are also regulated by TTX (see Figure 5) are marked with an asterisk.
-
Figure 4—source data 1
Synaptic protein regulation during homeostatic down-scaling.
- https://cdn.elifesciences.org/articles/52939/elife-52939-fig4-data1-v1.xlsx
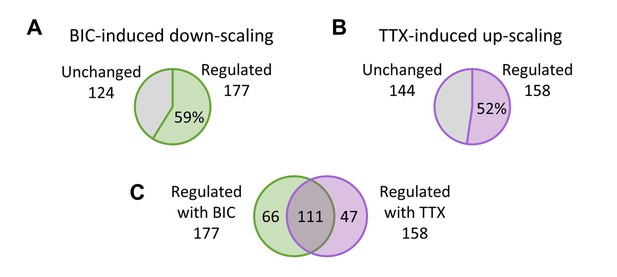
Regulation of synaptic proteins during homeostatic scaling.
Number and percentage of synaptic proteins that have changed synthesis and/or degradation rates during BIC-induced down-scaling (A) or TTX-induced up-scaling (B). (C) Overlap of synaptic proteins with changed synthesis and/or degradation rates upon BIC and TTX treatment.
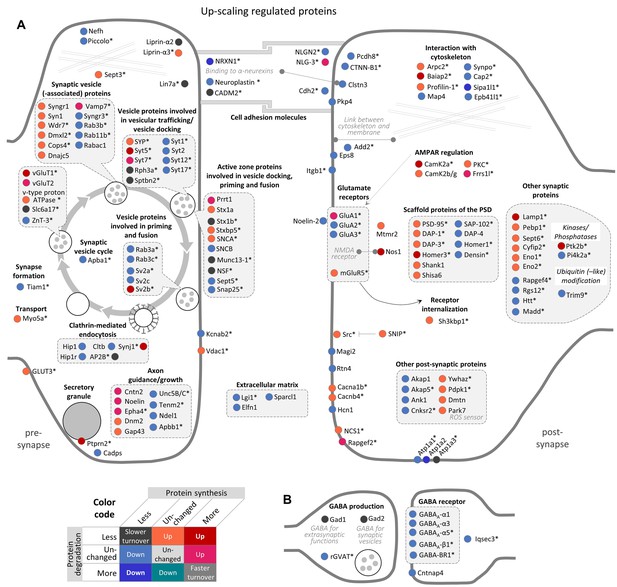
Regulation of synaptic proteins during TTX-induced homeostatic up-scaling.
Up-scaling regulated proteins associated with excitatory glutamatergic synapses (A) or inhibitory GABAergic synapses (B). Changes in protein synthesis and/or degradation are indicated by a color code. ‘Warm’ reddish colors indicate a change in synthesis/degradation that leads to increased protein copy numbers and ‘cold’ blueish colors indicate a change that leads to decreased protein copy numbers. Source data are provided in Figure 5—source data 1. Synaptic proteins that are also regulated by BIC (see Figure 4) are marked with an asterisk.
-
Figure 5—source data 1
Synaptic protein regulation during homeostatic up-scaling.
- https://cdn.elifesciences.org/articles/52939/elife-52939-fig5-data1-v1.xlsx
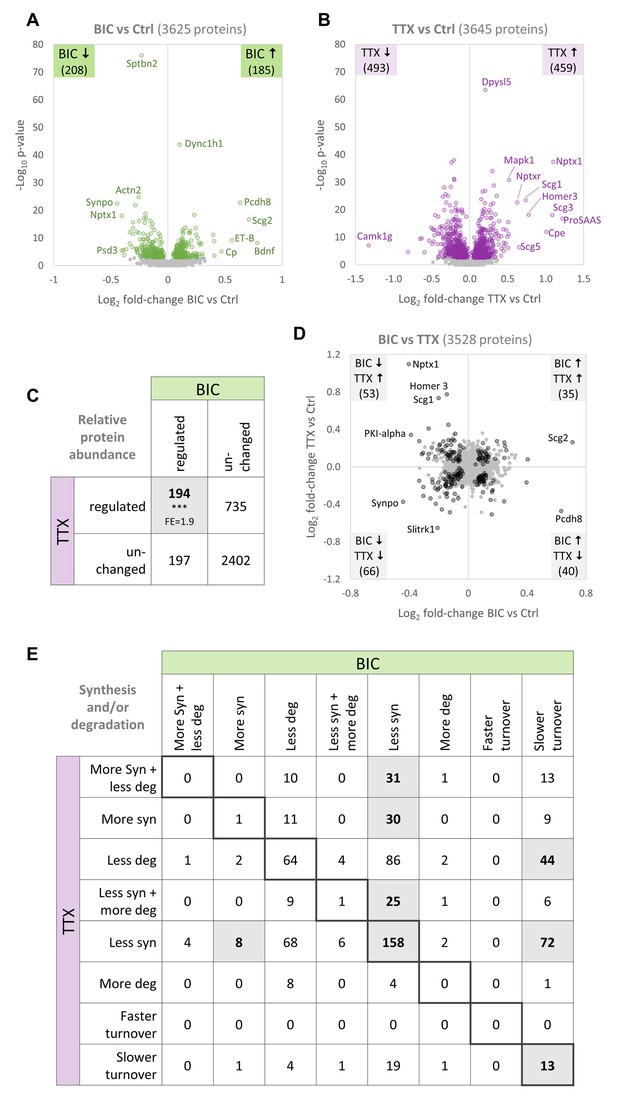
Comparison of protein changes during homeostatic up-scaling and down-scaling.
(A–B) Differential regulation of protein abundance during (A) BIC-induced down-scaling or (B) TTX-induced up-scaling compared to untreated controls. Significantly regulated proteins (FDR <0.01) are shown in color. Proteins that were not significantly regulated are shown in gray. (C) Numbers of proteins that were differentially regulated or unchanged in abundance during BIC-induced down-scaling and TTX-induced up-scaling. Significantly more proteins were regulated by both treatments than expected by chance (Fisher’s exact test, ***p<0.001). Fold-enrichment (FE) is indicated. (D) Fold-changes in relative protein abundance during TTX-induced up-scaling (y-axis) versus BIC-induced down-scaling (x-axis). Proteins that are significantly regulated by both treatments (BIC and TTX) are shown in black and proteins that are not significantly regulated are shown in gray. Source data are provided in Figure 6—source data 1. (E) Detailed comparison of changes in protein synthesis and/or degradation. Significantly over-represented combinations are highlighted. P-values and fold-enrichment are given in Figure 6—figure supplement 1B.
-
Figure 6—source data 1
Changes in protein abundance during homeostatic up- and down-scaling.
- https://cdn.elifesciences.org/articles/52939/elife-52939-fig6-data1-v1.xlsx
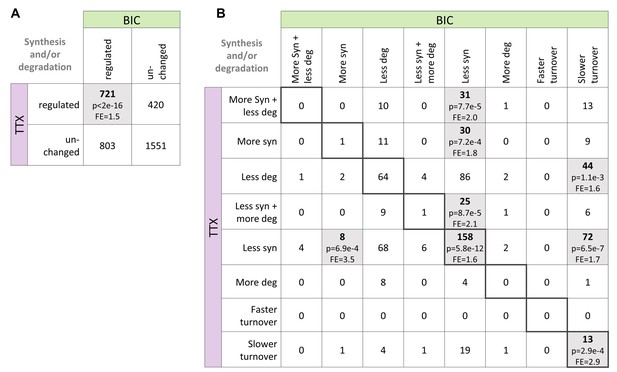
Comparison of changes in protein synthesis and/or degradation during homeostatic up- and down-scaling.
(A) Numbers of proteins with significant changes in protein synthesis and/or degradation during BIC-induced down-scaling and TTX-induced up-scaling. Significantly more proteins were affected by both treatments than expected by chance (Fisher’s exact test). P-value and fold-enrichment (FE) are indicated. (B) Detailed comparison of changes in protein synthesis and/or degradation. Significantly over-represented combinations are highlighted, and p-values and fold-enrichment (FE) are indicated.
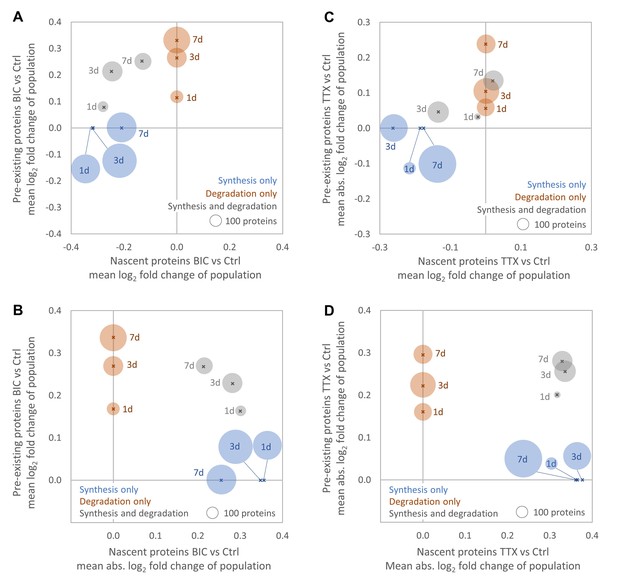
Temporal changes in protein synthesis and degradation during homeostatic scaling.
Global changes in protein synthesis and/or degradation after 1, 3 and 7 days of BIC-induced down-scaling (A, B) or TTX-induced up-scaling (C, D). For each time point, the average fold-change (A, C) and the average strength of regulation (B, D) of all proteins that are significantly regulated by synthesis (blue), degradation (brown) or both (grey) are shown. The circle size is proportional to the number of regulated proteins.
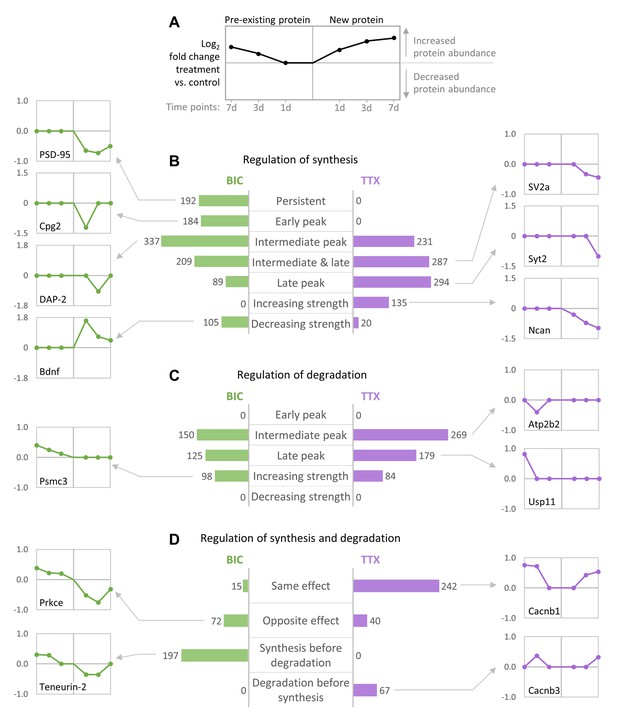
Temporal profiles of protein synthesis and degradation during homeostatic scaling.
Predominant temporal profiles of pre-existing and newly synthesized proteins observed during BIC-induced down-scaling and TTX-induced up-scaling. Proteins were clustered using their temporal profiles of pre-existing and newly synthesized proteins (k-means clustering), and the resulting clusters of proteins with similar profiles were manually assigned to upper-level groups (see Figure 8—figure supplements 1,2). (A) Description of graphs in panels (B–D) showing exemplary profiles. (B–D) The bar diagrams show the numbers of individual proteins assigned to upper-level groups that are mainly characterized by changes in synthesis (B), degradation (C) or both (D). Representative temporal profiles of proteins regulated during BIC-induced down-scaling (green) or TTX-induced up-scaling (purple) are shown.
-
Figure 8—source data 1
Temporal protein regulation during homeostatic up- and down-scaling.
- https://cdn.elifesciences.org/articles/52939/elife-52939-fig8-data1-v1.xlsx
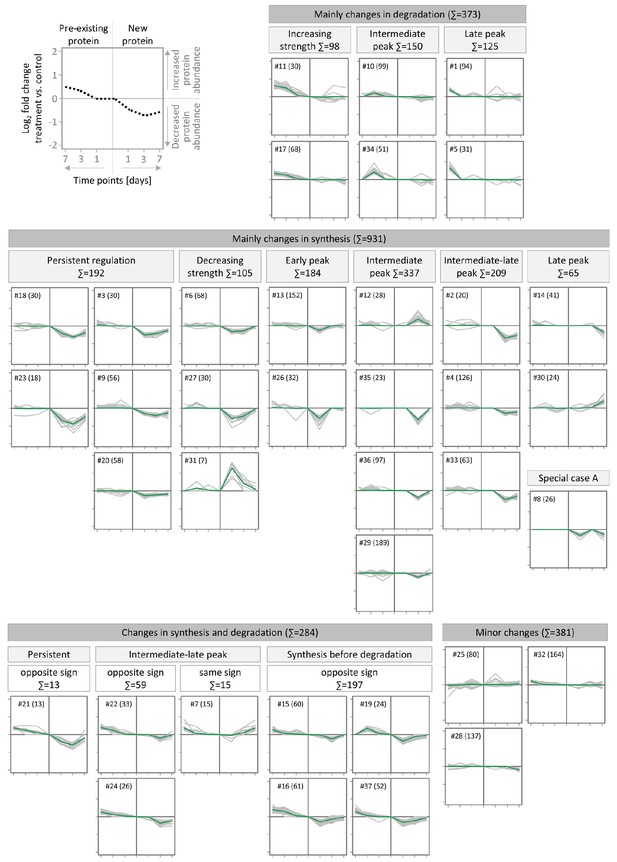
Clusters of temporal protein profiles observed during BIC-induced down-scaling.
Proteins were clustered using their temporal profiles of pre-existing and newly synthesized proteins by kmeans clustering. For each cluster, the temporal profiles of all members are shown in gray and the average profile is shown in green. The cluster number (#) and number of protein members (in brackets) are indicated. Clusters with similar profiles were manually assigned to upper-level groups. The schematic in the top left panel provides the axis information for the displayed clusters. The dashed line represents the temporal profile of an exemplary protein.
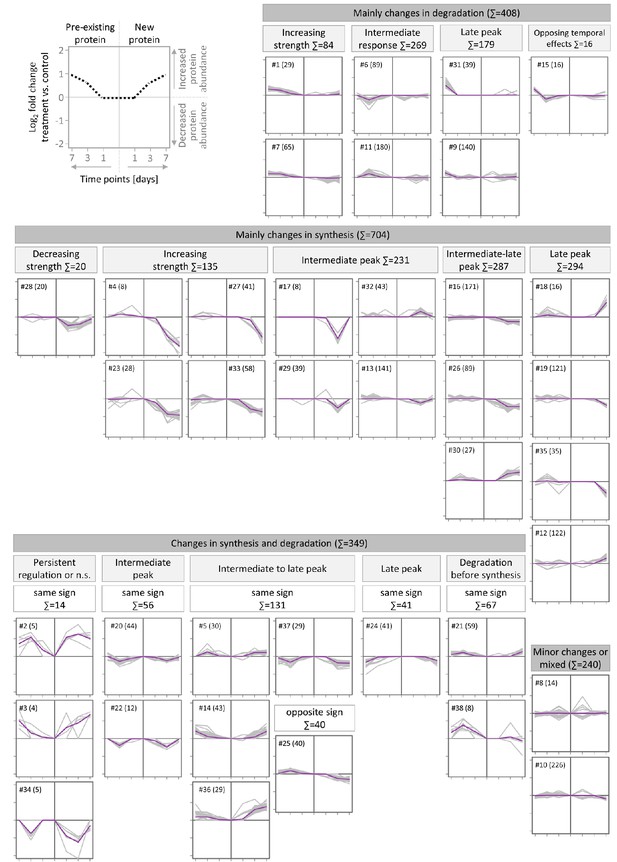
Clusters of temporal protein profiles observed during TTX-induced up-scaling.
Clusters of temporal protein profiles observed during TTX-induced up-scaling. Proteins were clustered using their temporal profiles of pre-existing and newly synthesized proteins by kmeans clustering. For each cluster, the temporal profiles of all members are shown in gray and the average profile is shown in green. The cluster number (#) and number of protein members (in brackets) are indicated. Clusters with similar profiles were manually assigned to upper-level groups. The schematic in the top left panel provides the axis information for the displayed clusters. The dashed line represents the temporal profile of an exemplary protein.
Additional files
-
Supplementary file 1
GO term over-representation of proteins with distinct regulation.
- https://cdn.elifesciences.org/articles/52939/elife-52939-supp1-v1.xlsx
-
Supplementary file 2
MS settings.
- https://cdn.elifesciences.org/articles/52939/elife-52939-supp2-v1.xlsx
-
Supplementary file 3
Sample overview and MaxQuant search parameters.
- https://cdn.elifesciences.org/articles/52939/elife-52939-supp3-v1.xlsx
-
Transparent reporting form
- https://cdn.elifesciences.org/articles/52939/elife-52939-transrepform-v1.docx