Synergistic roles of Synaptotagmin-1 and complexin in calcium-regulated neuronal exocytosis
Figures
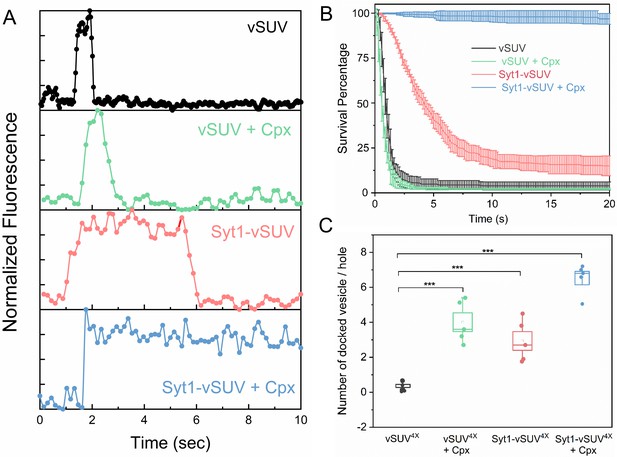
Syt1 and Cpx co-operatively clamp SNARE-mediated vesicle fusion under physiologically-relevant conditions.
The effect of Syt1 and Cpx on SNARE-driven fusion was assessed using a single-vesicle docking and fusion analysis with a pore-spanning bilayer setup (Ramakrishnan et al., 2019; Ramakrishnan et al., 2018). (A) Representative fluorescence (ATTO647N-PE) traces showing the behavior of small unilamellar vesicles (SUV) containing VAMP2 (vSUV) or Syt1 and VAMP2 (Syt1-vSUV) on t-SNARE containing bilayer in the presence or the absence of Cpx. (B) The time between docking and fusion was measured for each docked vesicle and the results for the whole population are presented as a survival curve. vSUVs (black curve) are diffusively mobile upon docking (Figure 1—figure supplement 2) and fuse spontaneous with a half-time of ~1 s. Addition of soluble Cpx (2 µM) does not change this behavior (green curve). Inclusion of Syt1 in the v-SUV (red curve) does not block fusion but increases the time from docking-to-fusion (~5 s half-life), in effect delaying the kinetics of fusion. When included together Syt1 and Cpx (blue curves) fully arrest fusion to produce stably docked SUVs that attach and remain in place during the entire period of observation. (C) Syt1 and Cpx, both individually and collectively increase the number of docked vesicles. In all cases, a mutant form of VAMP2 (VAMP24X) which eliminated fusion was used to unambiguously estimate the number of docked vesicles after the 10 min interaction phase. The average values and standard deviations from three to four independent experiments are shown for each condition. In sum, 500–1000 vesicles were analyzed for each condition.
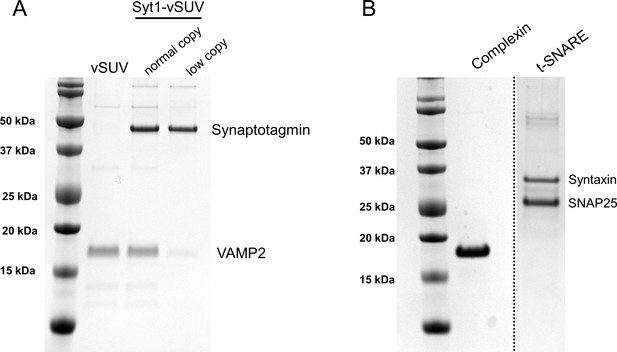
Coomaisse-stained SDS-PAGE analysis of the proteins used in this study.
(A) Gel image of the VAMP2 ±Syt1 vesicles. Based on the densitometry analysis, we estimated each vesicle contain 74 ± 4 and 26 ± 6 copies of outward-facing VAMP2 and Syt1 respectively under ‘normal’ physiologically-relevant condition and 13 ± 2 and 26 ± 6 copies of VAMP2 and Syt1 under low-copy conditions. (B) In all fusion experiments, co-purified t-SNAREs containing 1:1 complex of Syntaxin1a and SNAP25 was reconstituted into the free-standing bilayer and full-length Complexin 1 (2 µM final concentration) was added in solution.
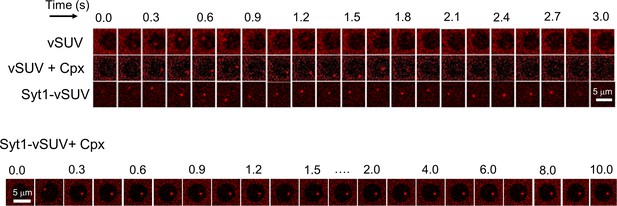
Representative time-lapse fluorescence (ATTO647N-PE) images showing the behavior of VAMP2- containing SUVs in presence of Syt1 and/or Cpx.
Representative time-lapse fluorescence (ATTO67N-PE) images of a docked SUVs showing that all VAMP2-containg SUVs (vSUVs) are diffusively mobile upon docking and fuse spontaneous within 1 s. This behavior is un-altered upon addition of 2 µM Cpx (vSUV + Cpx). Inclusion of Syt1 in the v-SUVs (Syt1-vSUV) introduces a significant docking-to-fusion delay, but most vesicles proceed to fuse spontaneously. In contrast, Cpx, when added along with Syt1 (Syt1-vSUV + Cpx), produces a stable clamp and the vesicles are docked in-place and largely immobile and do not fuse during the observation period. Images corresponding to a single 5 µm suspended bilayer is shown.
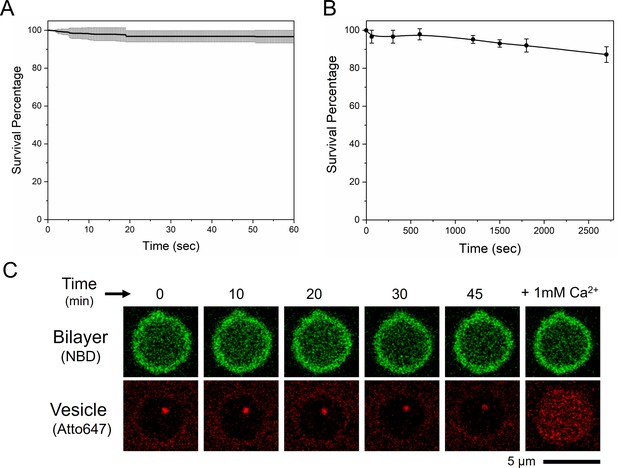
Survival analysis showing that vSUVs in the presence of Syt1 and Cpx dock and remain stably clamped for up to 1 hr*.
The vesicle fluorescence (ATTO647N-PE) were continuously imaged for the first 60 s post-docking with 150 ms frame rate (A), beyond which the vesicles were imaged only every 10 min for up to 1 hr (B) to minimize photo-bleaching. Average and standard deviation from three independent experiments are shown. (C) Representative fluorescence (ATTO647N-PE) image (bottom row) showing that Syt1/Cpx clamped vesicles remain Ca2+-sensitive even 1 hr post-docking. At each time point, we also recorded the NBD-fluorescence (top row) to verify the stability of the bilayer. Images corresponding to a single 5 µm suspended bilayer is shown. *Note: 1 hr is the maximum time-point we have tested so far considering the stability of the suspended bilayer.
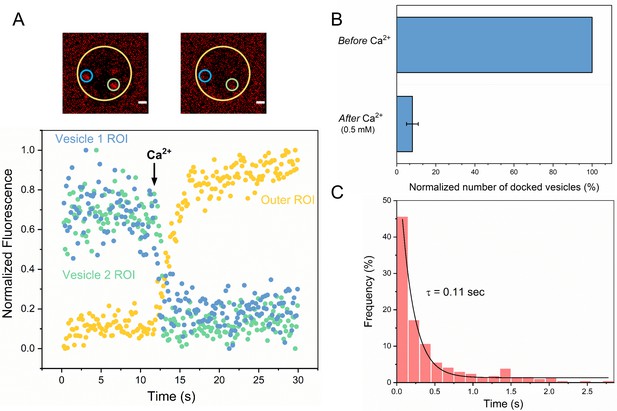
Syt1/Cpx clamped vesicles fuse synchronously and rapidly following Ca2+-addition.
(A) Representative fluorescence images (top) and quantitation of change in fluorescence signal (bottom) before and after addition of 1 mM Ca2+ shows that vesicles clamped by Syt1/Cpx are sensitive to Ca2+. Fusion was attested by a burst and sudden decrease in fluorescence (ATTO647N-PE) intensity as the lipids diffuse away. To visualize this, fluorescence was simultaneously monitored in a circular region of interest (ROI) encompassing the docked vesicle (vesicle ROI, green and blue circles) and in a surrounding annular ROI (outer ROI, yellow circle). Corresponding to actual fusion events, we observed a sudden decrease of fluorescence intensity in the vesicle ROI with a concomitant increase of fluorescence in the annular outer ROI. Note that the two docked vesicles fuse synchronously in response to Ca2+-influx. (B) End-point analysis at 1 min post Ca2+-addition shows that >90% of all clamped vesicles fuse following Ca2+ addition. (C) Kinetic analysis shows that the Syt1/Cpx clamped vesicles fuse rapidly following Ca2+-addition with a characteristic time constant of 0.11 s. This represents the temporal resolution limit of our recordings and the true Ca2+-triggered fusion rate is likely well below 0.1 s. Thus, Syt1 and Cpx synchronize vesicle fusion to Ca2+-influx and accelerate the underlying fusion process. The average values and standard deviations from three independent experiments (with ~1000 vesicles in total) is shown.
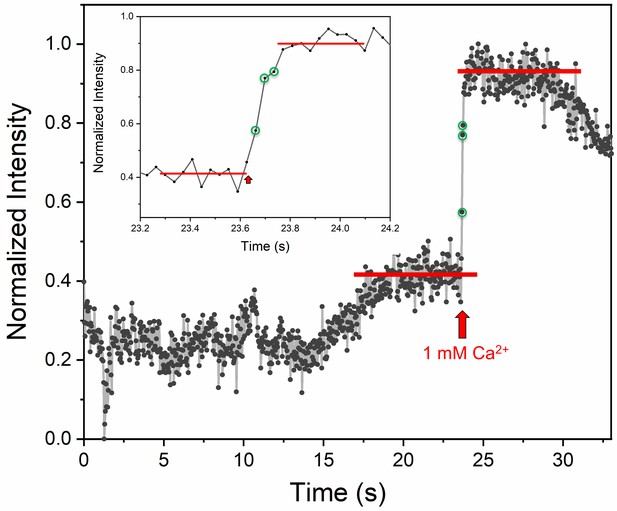
Ca2+-sensor dye, Calcium Green, introduced in the suspended bilayer (via a lipophilic 24-carbon alkyl chain) was used to monitor the arrival of Ca2+ at/near the docked vesicles.
A representative fluorescence trace acquired at a speed of ~36 msec per frame using confocal microscopy equipped with resonant scanner focused at or near the bilayer membrane is shown. The rise in fluorescence intensity upon Ca2+ binding was observed ~3 frames (~100 ms) after the addition of Ca2+ at the top of chamber. This was used to benchmark and to accurately estimate the Ca2+-triggered fusion rate.
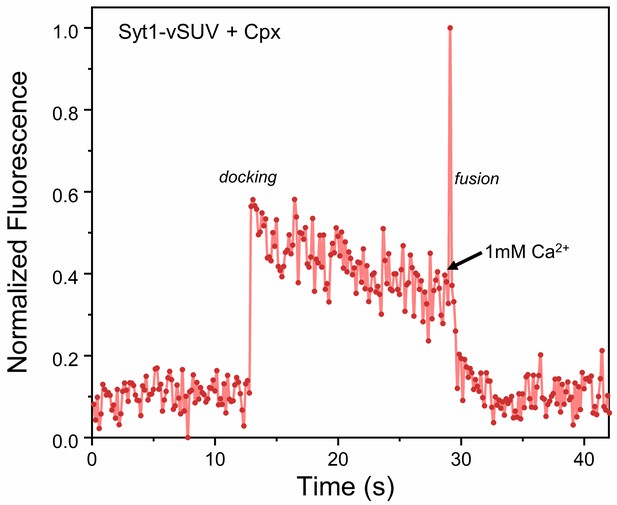
The effect of Syt1 and Cpx on SNARE-driven fusion assessed using a content-release assay with Sulforhodamine B loaded vesicles.
Sulforhodamine B is largely self-quenched when encapsulated inside a SUV. Fusion of vesicle results in dilution of the probe, which is accompanied by increasing fluorescence. Representative fluorescence trace showing that in the presence of 2 µM Cpx, the Syt1-vSUV (loaded with Sulphorhodamine B) dock and remain in place, until triggered to fuse by Ca2+ (1 mM) addition. This is in line with the lipid mixing data (Figure 2) showing that Syt1 and Cpx act together to establish Ca2+-regulated exocytosis.
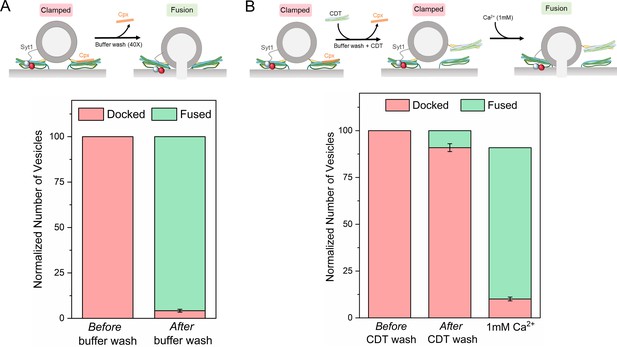
Syt1 and Cpx bind and clamp different pools of SNAREpins.
(A) In situ removal of Cpx from the Syt1/Cpx clamped state by extensive (40X) buffer wash triggers spontaneous fusion of the docked vesicles. This further confirms that both Syt1 and Cpx are required to produce a stable clamped state. (B) Inclusion of soluble cytoplasmic domain of t-SNAREs (CDT) blocked the spontaneous fusion events triggered by elimination of Cpx from the Syt1/Cpx clamped vesicles. The CDT-treated vesicles remain sensitive to Ca2+ influx and most of the vesicles fuse rapidly and synchronously following the addition of 1 mM Ca2+. This indicates that a sub-set of SNAREpins are protected against CDT even in the absence of Cpx, implying that Syt1 and Cpx likely engage and clamp different set of SNAREpins. It further shows that the Syt1-associated SNAREpins are sufficient to catalyze rapid Ca2+-triggered vesicle fusion. Data (average ± standard deviation) obtained from four to five independent experiments with at least 200 vesicles in total are shown. Note: Only single SNAREpins clamped by either Syt1 or Cpx is shown for illustrative purposes.
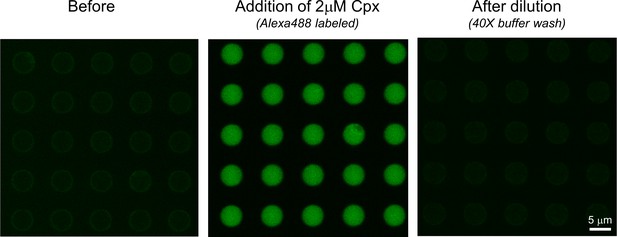
Representative fluorescence images showing that the extensive (40X) buffer wash results in complete washout of Cpx from clamped Syt1/Cpx vesicles.
This was assessed using fluorescently (Alexa488) labeled Cpx and fluorescence recorded prior to the addition of Cpx (left panel), upon addition of Cpx (middle) and after dilution by 40X buffer wash (right panel) are shown. Images corresponding to multiple 5 µm suspended bilayer is shown. Note: The vesicle and bilayer fluorescence are not shown for clarity.
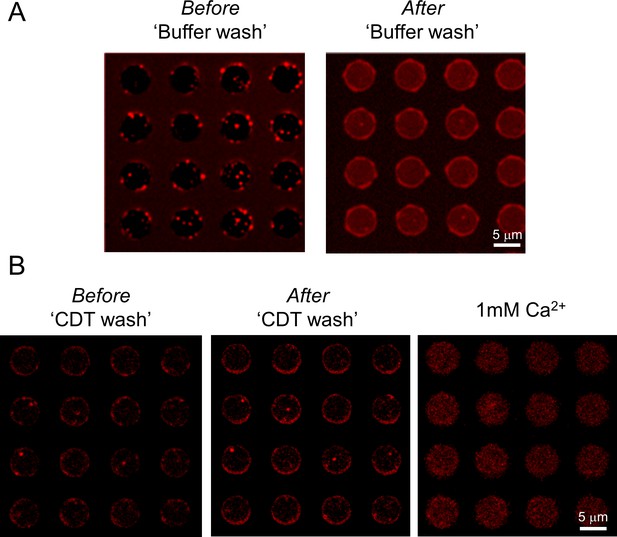
Respresentative fluorescence images showing the effect of CDT wash and subsequent Ca2+ addition.
(A) Representative fluorescence (ATTO647N) image showing that clamped Syt1/Cpx vesicles (left panel) fuse spontaneously upon wash out of Cpx by dilution. Upon fusion, the ATTO647N dye on the vesicles mixes with suspended bilayer resulting in increase of the background ATTO647N fluorescence (right panel). (B) In the presence of excess inhibitory soluble cytoplasmic domain of the t-SNARE complex (CDT), the vesicles remain docked even after the removal of previously-bound Cpx by the buffer wash (middle panel). Subsequent addition of Ca2+ (1 mM) triggered rapid and synchronous fusion of these docked vesicles. Images corresponding to multiple 5 µm suspended bilayer is shown.
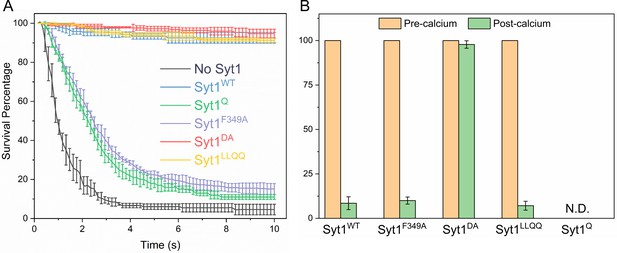
Molecular determinants of Syt1 clamp and its reversal by Ca2+.
To focus on the Syt1 component of the fusion clamp, all fusion analysis was carried out using vesicles containing low copy VAMP2 (~13 copies) with normal number (~25 copies) of Syt1 molecules (wild-type or targeted mutations) in the absence of Cpx. (A) Survival analysis shows that disrupting the Syt1-SNARE primary interface (Syt1Q, green curve) or destabilizing Syt1 oligomerization (Syt1F349A, purple curve) abrogates the Syt1 clamp, whilst the Syt1-Cpx-SNARE tripartite interface (Syt1LLQQ, yellow curve) and the Ca2+-binding motif on the Syt1 C2B domain (Syt1DA, red curve) are not involved in establishing the fusion clamp. (B) Addition of Ca2+ triggered rapid fusion of the majority (>90%) of Syt1LLQQ and the remainder (~15%) of the docked Syt1F349A vesicles, very similar to the behavior of the Syt1WT vesicles. Predictably, blocking Ca2+-binding to C2B domain rendered the vesicle Ca2+-insensitive, with the majority of Syt1DA remaining un-fused. We did not have sufficient number of docked Syt1Q vesicles to do a quantitative analysis, but qualitatively, the few that remained docked failed to fuse following Ca2+-addition. This implies that both C2B binding to Ca2+ and SNAREs are required for the Ca2+-activation, but the ability to form oligomers or Syt1-Cpx-SNARE tripartite interface are not crucial for the Ca2+-triggered reversal of the Syt1 clamp. The average values and standard deviations from four independent experiments are shown for each condition. In total,~250 vesicles were analyzed for each condition.
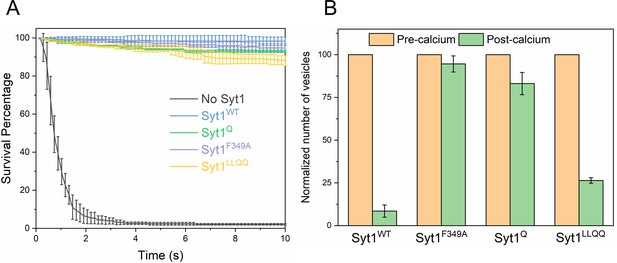
Syt1-clamped ‘central’ SNAREpins are required for Ca2+-evoked fusion.
The effect of targeted Syt1 mutations was assessed under physiologically-relevant SUV conditions (~74 copies of VAMP2 and ~25 copies of Syt1 wild type or mutant) in the presence of 2 μM Cpx. (A) Survival analysis shows neither the disruption of the Syt1-SNARE primary interface (Syt1Q, green curve) and the Syt1-Cpx-SNARE tripartite interface (Syt1LLQQ, yellow curve) nor destabilizing Syt1 oligomerization (Syt1F349A, purple curve) has any effect on the fusion clamp in the presence of Cpx, with stably docked vesicles observed under all conditions. (B) End-point analysis indicates that the Syt1Q and Syt1F349A vesicles are insensitive to Ca2+ and do not fuse upon addition of 1 mM Ca2+. In contrast, rapid and synchronous Ca2+-triggered fusion was observed with the majority of the docked Syt1WT and Syt1LLQQ vesicles. Notably, the LLQQ mutation does have a small but significant effect on Ca2+-triggered release. This implies that the Syt1-Cpx-SNARE tripartite interface is not necessary for clamp, but might be involved in the Ca2+-activation process. Overall, the data suggest that the Syt1 clamped SNAREpins are required for Ca2+-triggered exocytosis and in the absence of Syt1 clamp, Cpx irreversibly blocks vesicle fusion. The average values and standard deviations from three independent experiments are shown for each condition. In total,~200 vesicles were analyzed for each condition.
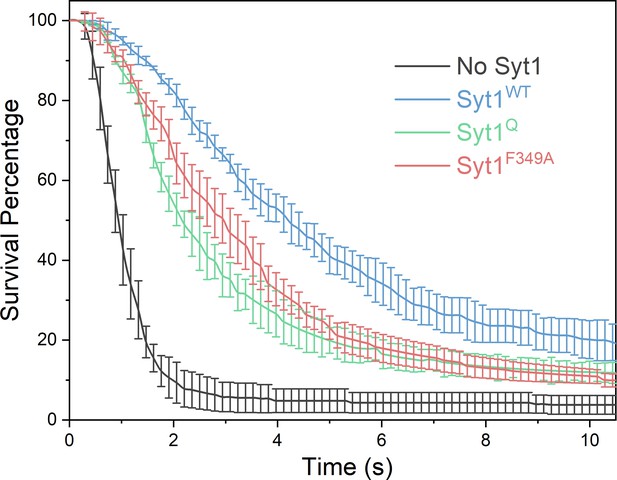
Syt1 non-clamping mutants introduce delay in fusion kinetics.
Survival analysis under physiologically-relevant SUVs (~74 copies of VAMP2 and ~25 copies of Syt1 wild type or mutant) in the absence of Cpx shows that Syt1 mutations that abrogate the fusion clamp i.e. primary interface (Syt1Q, green curve) or oligomerization (Syt1F349A, red curve) still introduce a meaningful (~2–3 s) delay in the fusion kinetics although less than observed with Syt1WT (blue curve). This delay might be sufficient for Cpx to bind and irreversibly block all SNARE driven fusion (Figure 5A). The average values and standard deviations from three independent experiments, from a total of ~150 vesicles each condition, is shown.
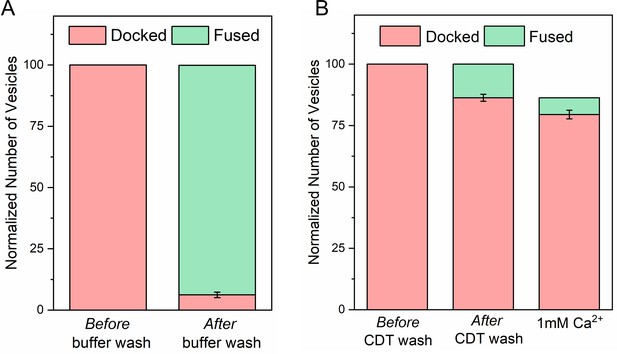
Cpx irreversibly blocks SNARE mediated fusion in the absence of Syt1 clamp.
(A) In situ removal of Cpx from the clamped Syt1Q vesicles by extensive (40X) buffer wash triggers spontaneous fusion of the docked vesicles (B) Inclusion of soluble cytoplasmic domain of t-SNAREs (CDT) blocked the spontaneous fusion events triggered by elimination of Cpx from the clamped Syt1Q vesicles. These CDT-treated vesicles are insensitive to Ca2+ and fail to fuse following the addition of 1 mM Ca2+. This shows in the Syt1Q vesicles, with no Syt1 clamp, all SNAREpins are arrested by Cpx alone and the SNARE-driven fusion is irreversibly blocked by CDT following the Cpx washout. Average and standard deviation from three independent experiments with at least 100 vesicles in total is shown.
Videos
Representative video showing the docking, diffusion and spontaneous fusion of a vSUV to free-standing t-SNARE containing lipid bilayer containing t-SNARE complex.
The movie was acquired using a Leica confocal scanning microscope at a speed of 7 frames/sec and the ATTO647N-PE fluorescence was used to track the fate of the vesicle. For clarity, a single hole corresponding to 5 µm suspended bilayer marked by the white circle is shown.
Representative video showing the docking, slow diffusion and delayed spontaneous fusion of a Syt1- containing vSUV to free-standing lipid bilayer containing t-SNAREs.
The movie was acquired using a Leica confocal scanning microscope at a speed of 7 frames/sec and the ATTO647N-PE fluorescence was used to track the vesicle. For clarity, a single hole corresponding to 5 µm suspended bilayer marked by the white circle is shown.
Representative video showing the immobile docking of a Syt1-vSUV to a free-standing t-SNARE containing lipid bilayer in the presence of 2 µM Cpx.
The ATTO647N-DOPE introduced in the SUV was used to track the vesicle and the movie was acquired using a Leica confocal scanning microscope at a speed of 7 frames/sec. For clarity, a single hole corresponding to 5 µm suspended bilayer marked by the white circle is shown.
Representative video showing the docking, diffusion and spontaneous fusion of a vSUV to free-standing t-SNARE containing lipid bilayer in the presence of 2 µM Cpx.
The movie was acquiredusing a Leica confocal scanning microscope at a speed of 7 frames/sec and the ATTO647N-PE fluorescence was used to track the vesicle. For clarity, a single hole corresponding to 5 µm suspended bilayer marked by the white circle is shown.
Representative video showing the rapid and synchronous fusion of multiple Syt1/Cpx clamped vesicles to free-standing t-SNARE containing lipid bilayer triggered by Ca2+-influx (1 mM free).
The ATTO647N-DOPE introduced in the SUV was used to track the fate of the vesicle and the movie was acquired using a Leica confocal scanning microscope at a speed of 7 frames/sec. For clarity, a single hole corresponding to 5 µm suspended bilayer marked by the white circle is shown.
Tables
Survival probabilities at specific points in time post-docking (Kaplan Meier estimators) for the VAMP2-containing vesicles (vSUV) in the presence of Cpx, Syt1 or both. The corresponding survival curves are shown in Figure 1B
Time (s) (post-docking) | vSUV | vSUV + Cpx | Syt1-vSUV | Syt1-vSUV + Cpx |
---|---|---|---|---|
0.588 | 0.7978 | 0.4945 | 0.9915 | 1.0000 |
1.029 | 0.4134 | 0.2277 | 0.9519 | 0.9989 |
2.058 | 0.0941 | 0.0544 | 0.8045 | 0.9963 |
3.087 | 0.0583 | 0.0319 | 0.6361 | 0.9936 |
4.116 | 0.0491 | 0.0267 | 0.5110 | 0.9917 |
5.145 | 0.0439 | 0.0243 | 0.3984 | 0.9888 |
7.497 | 0.0421 | 0.0215 | 0.2482 | 0.9821 |
9.996 | 0.0388 | 0.0211 | 0.1853 | 0.9814 |
15.141 | 0.0381 | 0.0209 | 0.1547 | 0.9794 |
20.139 | 0.0379 | 0.0201 | 0.1505 | 0.9682 |
The survival curves in Figure 1B were compared pair-wise using the log-rank test to determine the statistical significance (p-values) of the observed effects of Syt1 and Cpx on v-SUV fusion
vSUV | vSUV + cpx | Syt1-vSUV | Syt1-vSUV + cpx | |
---|---|---|---|---|
vSUV | n/a | p=0.731 | p<0.001 | p<0.0001 |
vSUV + Cpx | p=0.731 | n/a | p<0.0001 | p<0.0001 |
Syt1-vSUV | p<0.001 | p<0.001 | n/a | p<0.001 |
Syt1-vSUV + Cpx | p<0.0001 | p<0.0001 | p<0.001 | n/a |
Survival probabilities at specific points in time post-docking (Kaplan Meier estimators) for the different Syt1 mutants under low-copy VAMP2 conditions in the absence of Cpx. The corresponding survival curves are shown in Figure 4A
Time (s) (post-docking) | vSUV | Syt1WT | Syt1F349A | Syt13DA | Syt1Q | Syt1LLQQ |
---|---|---|---|---|---|---|
0.588 | 0.7925 | 0.9965 | 0.9597 | 0.9936 | 0.9612 | 0.9975 |
1.029 | 0.4679 | 0.9799 | 0.8951 | 0.9931 | 0.8353 | 0.9963 |
2.058 | 0.1697 | 0.9546 | 0.5992 | 0.9857 | 0.5403 | 0.9854 |
3.087 | 0.0899 | 0.9546 | 0.3822 | 0.9794 | 0.3193 | 0.9525 |
4.116 | 0.0662 | 0.9341 | 0.2745 | 0.9762 | 0.2163 | 0.9416 |
5.145 | 0.0606 | 0.9341 | 0.2209 | 0.9722 | 0.1732 | 0.9416 |
7.497 | 0.0549 | 0.9255 | 0.1663 | 0.9597 | 0.1253 | 0.9160 |
9.996 | 0.0459 | 0.9189 | 0.1514 | 0.9475 | 0.1152 | 0.9124 |
The survival curves in Figure 4A were compared pair-wise using the log-rank test to determine the statistical significance (p-values) of the effects of the Syt1 mutants under low-copy VAMP2 conditions in the absence of Cpx.
vSUV | Syt1WT | Syt1F349A | Syt13DA | Syt1Q | Syt1LLQQ | |
---|---|---|---|---|---|---|
vSUV | n/a | p<0.0001 | p<0.004 | p<0.0001 | p<0.009 | p<0.0001 |
Syt1WT | p<0.0001 | n/a | p<0.0001 | p=0.912 | p<0.0001 | p=0.885 |
Syt1F349A | p<0.004 | p<0.0001 | n/a | p<0.0001 | p=0.113 | p<0.0001 |
Syt13DA | p<0.0001 | p=0.912 | p<0.0001 | n/a | p<0.0001 | p=0.915 |
Syt1Q | p<0.009 | p<0.0001 | p=0.113 | p<0.0001 | n/a | p<0.0001 |
Syt1LLQQ | p<0.0001 | p=0.885 | p<0.0001 | p=0.915 | p<0.0001 | n/a |
Survival probabilities at specific points in time post-docking (Kaplan Meier estimators) for the different Syt1 mutants under normal VAMP2 conditions in the presence of 2 μM Cpx. The corresponding survival curves are shown in Figure 5A.
Time (s) (post-docking) | vSUV | Syt1WT | Syt1F349A | Syt1Q | Syt1LLQQ |
---|---|---|---|---|---|
0.588 | 0.6714 | 0.9959 | 0.9936 | 0.9913 | 0.9878 |
1.029 | 0.2981 | 0.9936 | 0.9910 | 0.9821 | 0.9810 |
2.058 | 0.0617 | 0.9927 | 0.9857 | 0.9734 | 0.9688 |
3.087 | 0.0391 | 0.9905 | 0.9794 | 0.9646 | 0.9566 |
4.116 | 0.0267 | 0.9852 | 0.9762 | 0.9573 | 0.9471 |
5.145 | 0.0243 | 0.9841 | 0.9722 | 0.9445 | 0.9444 |
7.497 | 0.0215 | 0.9833 | 0.9597 | 0.9379 | 0.8943 |
9.996 | 0.0195 | 0.9824 | 0.9475 | 0.9301 | 0.8808 |
The survival curves shown in Figure 5A were compared pair-wise using the log-rank test to determine the statistical significance (p-values) of the effects of the Syt1 mutants under normal VAMP2 conditions in the presence of 2 μM Cpx.
vSUV | Syt1WT | Syt1F349A | Syt1Q | Syt1LLQQ | |
---|---|---|---|---|---|
v-SUV | n/a | p<0.0001 | p<0.0001 | p<0.0001 | p<0.0001 |
Syt1WT | p<0.0001 | n/a | p=0.971 | p=0.912 | p=0.151 |
Syt1F349A | p<0.0001 | p=0.971 | n/a | p=0.865 | p=0.906 |
Syt1Q | p<0.0001 | p=0.912 | p=0.865 | n/a | p=0.426 |
Syt1LLQQ | p<0.0001 | p=0.151 | p=0.906 | p=0.426 | n/a |
Additional files
-
Source data 1
Ramakrishan et al_2020_Source Data.
- https://cdn.elifesciences.org/articles/54506/elife-54506-data1-v1.xlsx
-
Transparent reporting form
- https://cdn.elifesciences.org/articles/54506/elife-54506-transrepform-v1.pdf