Structure of substrate-bound SMG1-8-9 kinase complex reveals molecular basis for phosphorylation specificity
Figures
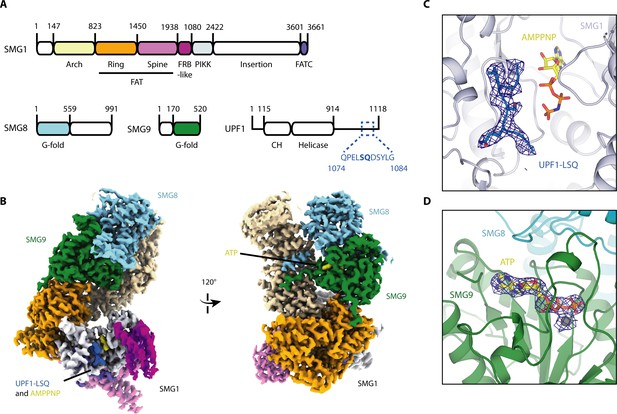
Cryo-EM reconstruction of SMG1-8-9 bound to UPF1-LSQ.
(A) Domain organization of SMG1, SMG8, SMG9 and UPF1. White parts are not resolved in the reconstruction. The sequence and location of UPF1-LSQ is indicated with blue text and dotted lines. (B) Segmented cryo-EM reconstruction of substrate-bound SMG1-8-9. Two different views are shown; proteins and domains are colored as in A. (C) A zoomed-in view of SMG1 showing the kinase active site with bound AMPPNP and UPF1-LSQ. Reconstructed density for UPF1-LSQ is shown as a blue mesh. (D) Zoom-in showing ATP bound to SMG9 with reconstructed density displayed as a blue mesh.
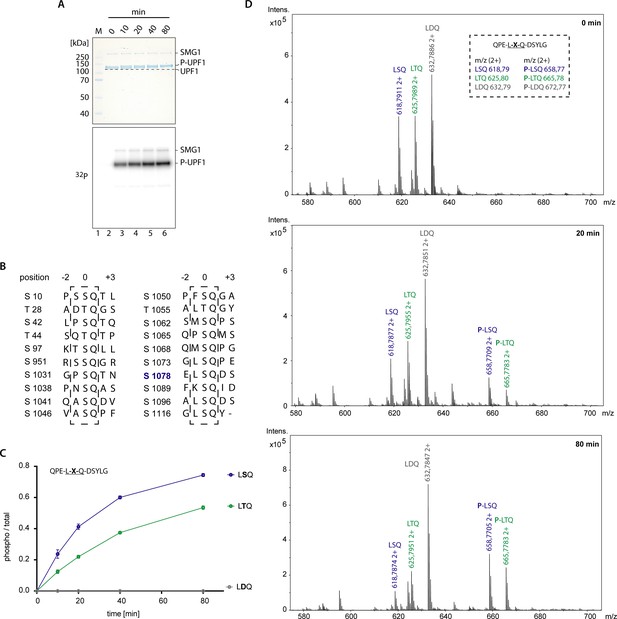
SMG1-8-9 activity and UPF1 SQ motifs.
(A) Radioactive phosphorylation assay using SMG1-8-9 and full-length UPF1. Coomassie-stained SDS-PAGE showing a change in migration behavior for UPF1 over time as phosphorylation proceeds. The corresponding radioactive signal is shown in the lower panel indicating an increase of UPF1 phosphorylation over time. (B) Alignment showing all SQ motifs present in UPF1 N- and C-terminus including positions −2 to +3. Note the high variance amongst position −1 residues. (C) Mass spectrometry-based phosphorylation assay with UPF1-LSQ and the indicated position 0 variations. The peptide sequence is indicated with the varying position marked as ‘X’. Error bars representing standard deviations calculated from independent experimental triplicates are shown. Phosphorylation was abolished when the phospho-acceptor residue was changed from Ser to Asp. This shows Ser1081 was not recognized for phosphorylation and confirms specificity toward the SQ motif. In addition, phosphorylation was decreased when Ser was changed to Thr, consistent with previous data for ATM, ATR and DNA-PK (Kim et al., 1999; O'Neill et al., 2000). (D). M/z spectra for representative single measurements at indicated time points of the experiment shown in C. The inset lists the expected sizes for the three peptides used in this experiment. Peaks corresponding to unphosphorylated and phosphorylated peptides are highlighted. Note the appearance and increase of intensity of peaks corresponding to phosphorylated peptides over time.
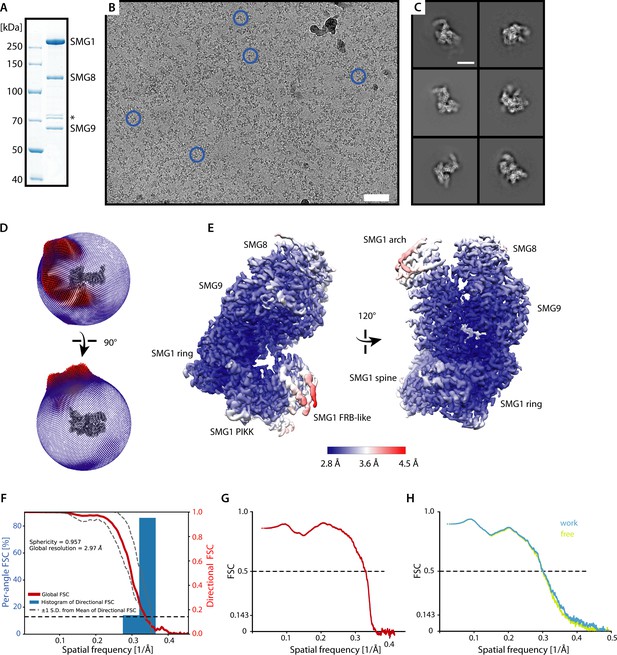
Cryo-EM analysis of SMG1-8-9 bound to UPF1-LSQ.
(A) Coomassie-stained SDS-PAGE showing purified SMG1-8-9. The asterisk indicates contaminants. (B) Representative micrograph of the collected data set with some SMG1-8-9 particles indicated by blue circles. Scale bar ≈ 500 Å. (C) Representative 2D averages of picked particles. Scale bar ≈ 100 Å. (D) Spherical angular distribution of particles contributing to the final reconstruction with larger red rods indicating more prominent particle views and smaller blue rods indicating rarer particle views. (E) Map of SMG1-8-9 colored according to estimated local resolution shown in two different views as in Figure 1B. Large parts of the complex including the kinase domain and the bound UPF1 peptide are resolved to around 3 Å. Important features of the map are indicated. (F) Three-dimensional FSC plot and further analysis of orientation bias. The red line represents the estimated global masked half-map FSC curve indicating a nominal overall resolution of 2.97 Å according to the gold standard FSC cut off of 0.143 (Rosenthal and Henderson, 2003). The spread of directional resolution values is defined as ± 1σ (shown as dashed grey lines). Overall isotropy of the map is confirmed by a sphericity of 0.957 (out of 1) (Tan et al., 2017). (G) Model vs. map FSC plot for the real spaced refined model. (H) Model vs. map FSC plots for half map 1 ("work") used for real space refinement after displacing atoms of the final model (σ = 0.5 Å) and half map 2 not used for refinement ("free"). The good agreement of the two curves indicates that no substantial overfitting took place.
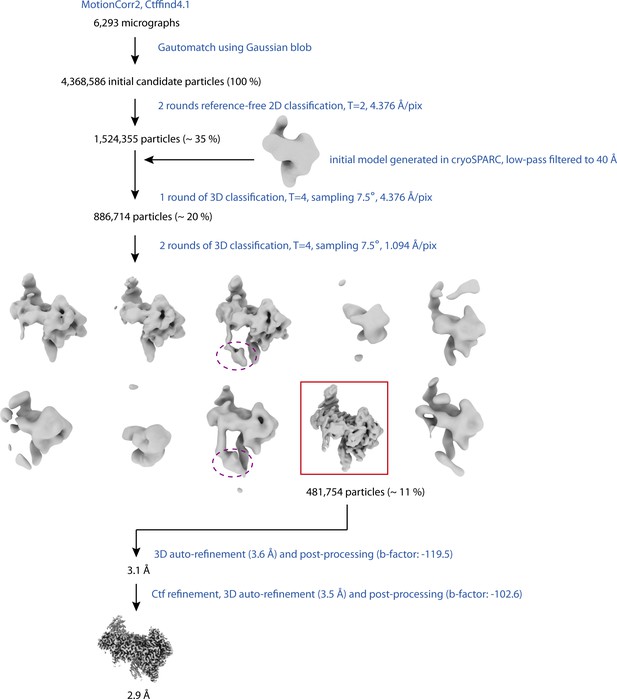
Cryo-EM data processing scheme.
Processing steps are indicated in blue; particle numbers of classes used for downstream processing steps (in brackets: percentage with respect to initial candidate particles) and resolutions are in black. The class selected for the 3D refinement after the last step of 3D classification is indicated by a red rectangle. Density for the unmodelled, C-terminal part of SMG8 appearing in two other 3D classes not used for the final reconstruction is highlighted in magenta. This part of SMG8 has been suggested to contribute to kinase regulation (Zhu et al., 2019).
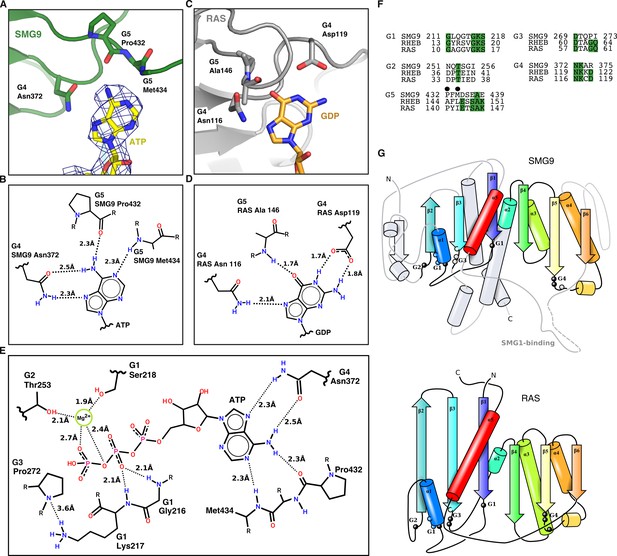
SMG9 is a G-fold containing protein binding ATP and exhibits distinct differences to the bona fide GTPase RAS.
(A) Detailed view of ATP purine ring recognition by SMG9 with important residues highlighted and reconstructed density indicated. (B) Two-dimensional sketch of adenine base recognition by SMG9 shown in panel A with G motifs, key residues and distances indicated. (C) Detailed view of GTP recognition by the GTPase RAS with important residues highlighted (PDB ID 1ZVQ). (D) Two-dimensional sketch of guanine base recognition shown in panel C by RAS with G motifs, key residues and distances indicated for comparison with panel B (based on PDB ID 1ZVQ). (E) Two-dimensional sketch of the overall recognition of ATP by SMG9. The G1-G3 motifs of SMG9 coordinate the phosphate moieties of ATP, similarly to the corresponding motifs of canonical GTPases, but with an important exception, in that they lack the typical residues crucial for catalysis. Another difference with canonical G-fold is that the G4 and G5 motifs responsible for the recognition of the base have rearranged to preferentially bind an adenine base rather than a guanine. (F) Sequence alignment of G motifs of RHEB and RAS GTPases with SMG9. Residues highlighted in A are indicated by a black dot. In the G4 motif, the aspartic acid that specifically engages the guanine moiety in GTPases has diverged to an arginine residue. Instead, Asn372 in the SMG9 G4 motif has shifted so that it engages the side-chain carbonyl group to interact with the adenine amino group (compare panels A and B with C and D). In the G5 motif, the SAK consensus sequence in RAS and RHEB has diverged in SMG9, with the main-chain carbonyl and amine groups of the upstream residues, Pro432 and Met434, forming adenine-specific interactions (compare panels A and B with C and D). (G) Three-dimensional sketches of SMG9 (top) and RAS/RHEB (bottom) highlighting similarities in their overall topology. Positions of the G motifs are highlighted.
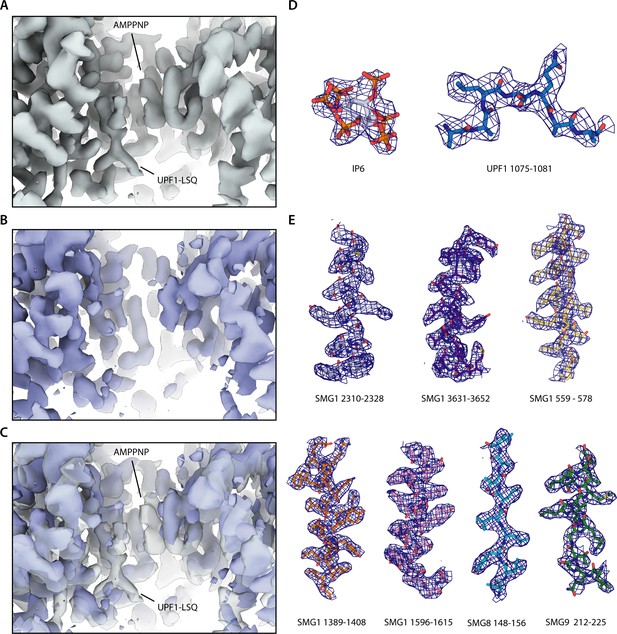
Quality of the reconstructed density.
(A) Active site density reported in this study. Densities attributed to either AMPPNP or UPF1 peptide are indicated. (B) Active site density of the apo SMG1-8-9 reconstruction reported previously (EMDB 10347). (C) Overlay of the active site densities shown in A and B with the active site density reported in this study shown in transparent grey and density of apo SMG1-8-9 (EMDB 10347) shown in blue. Densities attributed to either AMPPNP or UPF-LSQ are indicated. (D) Model of IP6 and UPF1-LSQ with the corresponding density shown as a blue mesh. Colors of the model as in Figure 1. (E) Additional parts of the model with the corresponding density shown as in B.
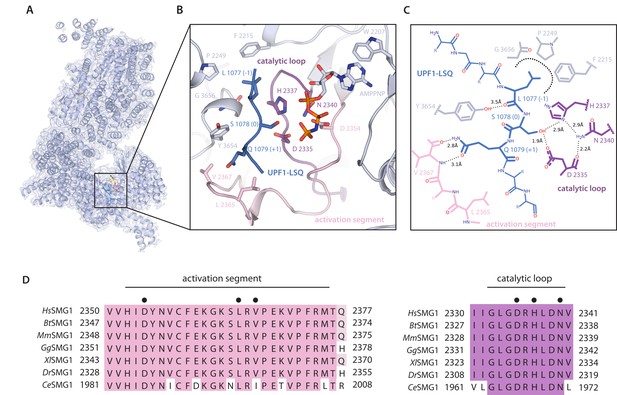
Organization of the substrate-bound kinase active site.
(A) The structure of the entire complex is shown overlayed with transparent reconstructed density. The black box indicates the location of the kinase active site. (B) SMG1 active site with important residues shown as sticks. Activation segment and catalytic loop as indicated; the p-loop was omitted for clarity. UPF1-LSQ is shown in blue with positions of important residues highlighted. (C) Two-dimensional sketch of the SMG1 active site with key kinase-substrate interactions indicated. (D) Activation segment (pink) and catalytic loop (magenta) regions of a SMG1 sequence alignment are shown, indicating a high level of conservation across Homo sapiens, Bos taurus, Mus musculus, Gallus gallus, Xenopus laevis, Danio rerio and Caenorhabditis elegans. Key residues shown in B are highlighted by a black dot and activation segment and catalytic loop are indicated.
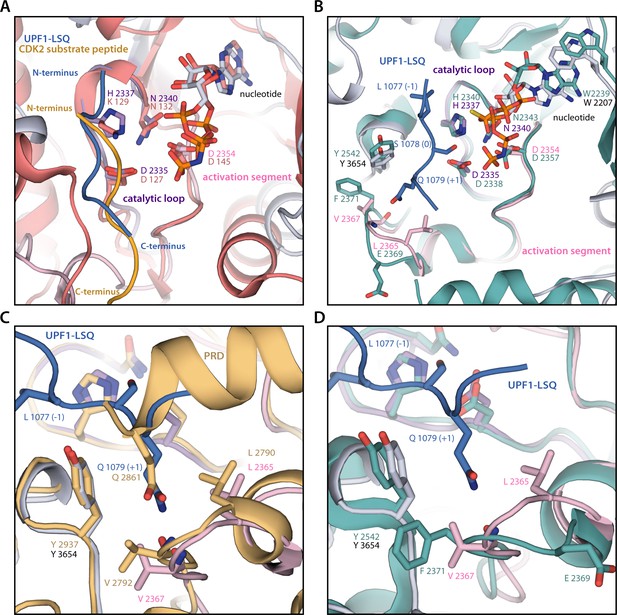
Comparison of SMG1 substrate-bound active site to other protein kinases.
(A) Superposition of substrate-bound SMG1 active site with substrate-bound CDK2 active site (PDB ID, 3QHW). SMG1 catalytic loop is colored in magenta, SMG1 activation segment is in pink, CDK2 is shown in salmon and the CDK2 substrate peptide is in orange. Directionality of the substrates and important catalytic residues are indicated and colored as described above. P-loops were omitted for clarity. The SMG1 kinase contains features that have previously been associated with a model preferring the presence of a ‘dissociative’ transition state, such as a positive charge closely involved in β-phosphate coordination, namely K2155 (residue not shown) (Wang and Cole, 2014). (B) Superposition of substrate-bound SMG1 active site with nucleotide-bound mTOR active site (PDB ID, 4JSP). SMG1 active site is colored as described in panel A and mTOR is in green. Important active site residues are indicated. P-loops were omitted for clarity. (C) Close-up of the active site hydrophobic cage after superposing the model of the substrate-bound SMG1 active site with Chaetomium thermophilum Tel1ATM (PDB ID, 6SKY). SMG1 active site is colored as described in panel A and CtTel1ATM is colored in light orange. Key residues are indicated. (D) Close-up of the active site hydrophobic cage based on the superposition of SMG1 and mTOR shown in B. Color scheme as in B and important residues are indicated. View is similar to C.
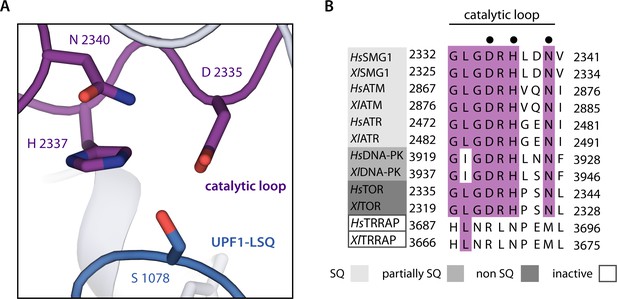
Recognition of UPF1-LSQ phospho-acceptor residue Ser1078 by SMG1 catalytic loop residues.
(A) Close-up showing how residues of the SMG1 catalytic loop coordinate Ser1078 of UPF1-LSQ. (B) Alignment of catalytic loop sequences from Homo sapiens and Xenopus laevis for all PIKKs colored according to conservation. PIKKs are grouped by phosphorylation site specificity and residues highlighted in subfigure C are indicated by a black dot.
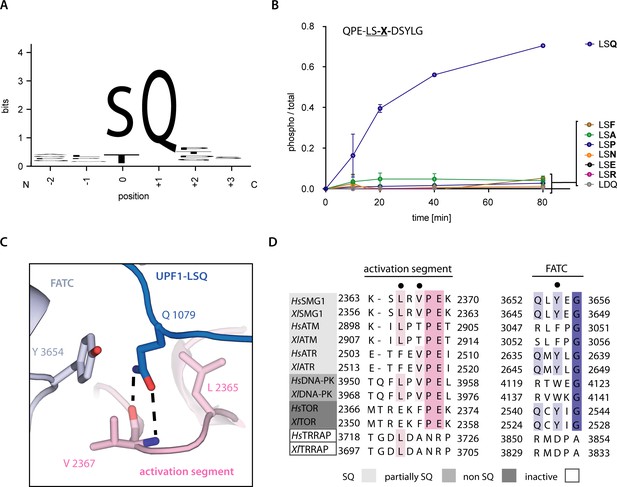
Recognition of position +1 glutamine of UPF1-LSQ.
(A) Sequence logo derived from an alignment of all SQ motifs present in human UPF1 with the respective residue positions indicated. The heights of single letters correspond to the observed frequency at that position and the overall height of a stack of letters indicates the level of conservation (Figure 1—figure supplement 1B and Figure 4—figure supplement 2; Crooks et al., 2004). (B) Mass spectrometry-based phosphorylation assay comprising UPF1-LSQ and the indicated position +1 variations. The peptide sequence is indicated in the upper left with the varied position marked as ‘X’. Error bars representing standard deviations calculated from independent experimental triplicates are shown. (C) Zoom-in of the SMG1 active site showing the recognition of UPF1 position +1 glutamine by SMG1 residues located in the activation segment and FATC domain. Residues of interest are shown as sticks. Colors as in Figure 2. (D) Alignment of PIKK sequences from Homo sapiens and Xenopus laevis with the activation segment and FATC domain sequences shown and colored according to conservation. PIKKs are grouped by phosphorylation site specificity and residues highlighted in subfigure C are indicated by a black dot.
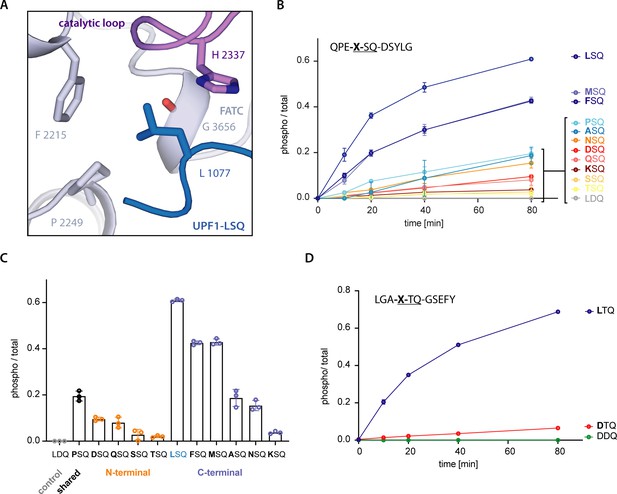
SMG1 preferentially selects for substrates with hydrophobic residues in position -1.
(A) Recognition of position -1 residue of UPF1-LSQ by SMG1. Important residues are shown as sticks and colored as in Figure 2. (B) Mass spectrometry-based phosphorylation assay with UPF1-LSQ and derivatives varied in position -1. The peptide sequence is indicated in the upper left with the varying position marked as “X”. Error bars representing standard deviations calculated from independent experimental triplicates are shown and curves are colored according to hydrophobicity of the position -1 residue (Eisenberg et al., 1984). The most hydrophobic peptides are in blue while non-hydrophobics are in red. (C) Final time points of experiment shown in B. Peptides grouped and colored according to the location of the respective position -1 residue in UPF1 N- or C-terminus. Individual data points are shown as circles and error bars representing standard deviations are indicated. (D) Mass spectrometry-based phosphorylation assay with UPF1 N-terminus phosphorylation site 28 and the indicated position -1 variation. The peptide sequence is shown in the upper left with the varied position marked as “X”. Error bars represent standard deviations resulting from independent experimental triplicates. A tyrosine residue was added to the C-terminal end of the wildtype sequence to increase absorbance at = 280 nm.
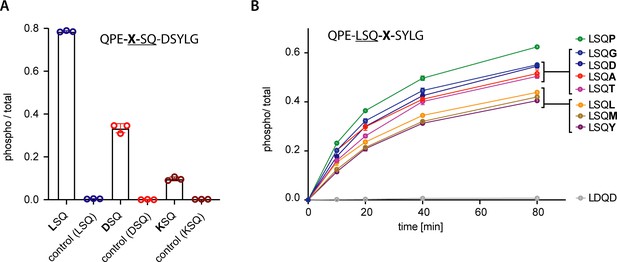
In vitro phosphorylation of UPF1-LSQ and derivatives.
(A) Mass spectrometry-based end-point measurements of single peptides combined with a control. Data points corresponding to one experiment are shown in the same color. One preferred (LSQ) and two suboptimal (DSQ, KSQ) motifs were chosen to evaluate how the absence of more optimal, competing motifs affects phosphorylation efficiency toward single peptides. The results indicate that decreased phosphorylation of peptides with non-hydrophobic position −1 residues is not only caused by competition with more optimal substrates (compare assay shown in Figure 4B). A peptide with the sequence QPELDQDSYLG was used as a control in all three experiments. Reactions were quenched and analyzed after 80 min. The peptide sequence is shown with the varied position marked as ‘X’ (B) Mass spectrometry-based phosphorylation assay with UPF1-LSQ and derivatives varying in position +2. The peptide sequence is indicated with the varying position marked as ‘X’. Error bars representing standard deviations calculated from independent experimental triplicates are shown.
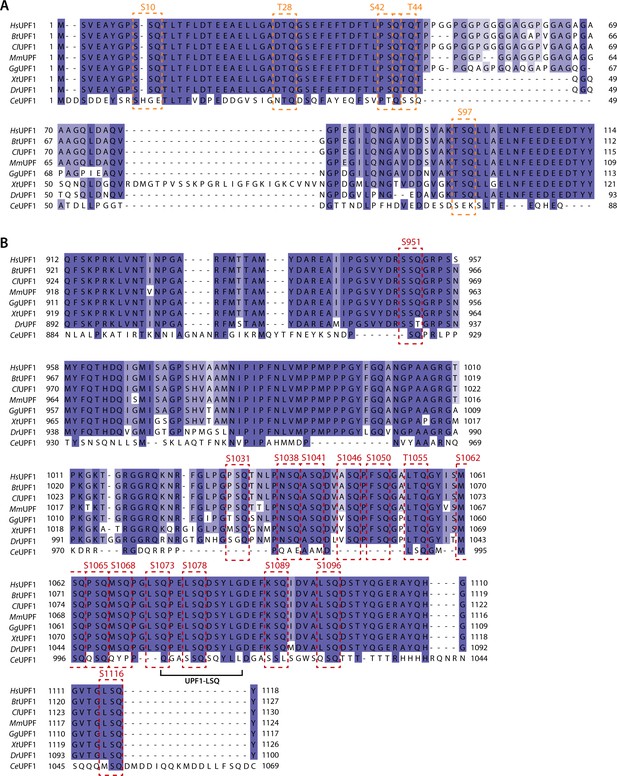
Alignment of UPF1 N- and C-terminal sequences from Homo sapiens, Bos taurus, Canis lupus, Mus musculus, Gallus gallus, Xenopus tropicalis, Danio rerio and Caenorhabditis elegans.
The alignment is colored according to conservation. (A) Sequence alignment of UPF1 N-terminus. SQ motifs are highlighted by orange boxes with the phosphorylation site position indicated on top. (B) As in A, but for UPF1 C-terminus and using red color to indicate SQ motifs. Location and range of UPF1-LSQ are indicated.
Tables
Reagent type (species) or resource | Designation | Source or reference | Identifiers | Additional information |
---|---|---|---|---|
Gene (Homo sapiens) | SMG1 | Shigeo Ohno lab | Uniprot Q96Q15 | |
Gene (Homo sapiens) | SMG8 | Shigeo Ohno lab | Uniprot Q8ND04 | |
Gene (Homo sapiens) | SMG9 | Shigeo Ohno lab | Uniprot Q9H0W8 | |
Cell line (Homo sapiens) | HEK293T | ATCC | ||
Strain, strain background (Escherichia coli) | BL21 Star (DE3) pRARE | EMBL Heidelberg Core Facility | Electrocompetent cells | |
Peptide, recombinant protein | UPF1- LSQ (peptide 1078) and derivatives, UPF1- peptide 28 and derivatives | in-house as described in the Materials and methods section | ||
Chemical compound, drug | AMPPNP | Sigma-Aldrich | ||
Chemical compound, drug | ATP | Sigma-Aldrich | ||
Software, algorithm | RELION | doi: 10.7554/eLife.42166 | RELION 3.0 | |
Software, algorithm | Cryosparc | doi: 10.1038/nmeth.4169 | Cryosparc2 | |
Software, algorithm | CtfFind | doi: 10.1016/j.jsb.2015.08.008 | CtfFind4.1.9 | |
Software, algorithm | Cryosparc | doi: 10.1038/nmeth.4169 | Cryosparc2 | |
Software, algorithm | UCSF Chimera | UCSF, https://www.cgl.ucsf.edu/chimera/ | ||
Software, algorithm | UCSF ChimeraX | UCSF, https://www.rbvi.ucsf.edu/chimerax/ | ||
Software, algorithm | COOT | http://www2.mrc-lmb.cam.ac.uk/personal/pemsley/coot/ | ||
Software, algorithm | PHENIX | https://www.phenix-online.org/ | PHENIX 1.17 | |
Software, algorithm | PyMOL | PyMOL Molecular Graphics System, Schrodinger LLC | PyMOL 2.3.2 | https://www.pymol.org/ |
Additional files
-
Supplementary file 1
Cryo-EM data collection, refinement and validation statistics.
- https://cdn.elifesciences.org/articles/57127/elife-57127-supp1-v2.docx
-
Transparent reporting form
- https://cdn.elifesciences.org/articles/57127/elife-57127-transrepform-v2.pdf