Reduced purine biosynthesis in humans after their divergence from Neandertals
Figures
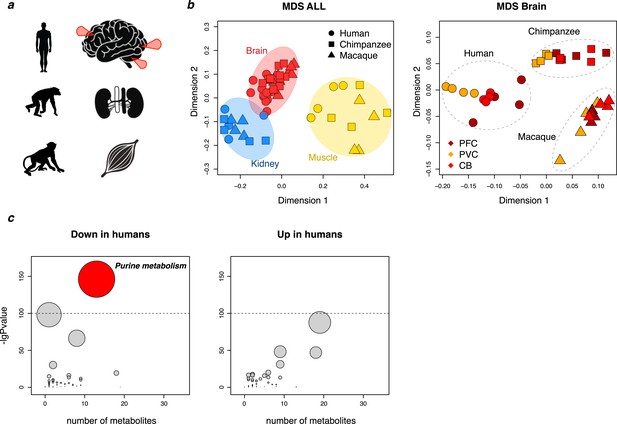
Primate metabolome analysis.
(a) Sketch depicting species and tissues used for metabolite measurements. Red arrows indicate sampled brain regions: the dorsolateral prefrontal cortex, primary visual cortex, and cerebellar cortex. (b) Multidimensional scaling (MDS) analysis of all samples (left) and brain samples (right) based on the intensities of all detected metabolic peaks. (c) An analysis of KEGG-based metabolic pathway showing for each pathway (circle) the number of detected metabolites (X axis) and the cumulative p-value of the difference (Y axis) between human and non-human primate (chimpanzee and macaque) intensity levels within the pathway in the five tissues. Left: an analysis based on metabolites with lower intensity in humans compared to non-human primates. The oxidative phosphorylation pathway is the second highest. Right: an analysis based on metabolites with higher intensity in humans. The size of the symbols is proportional to the -logarithm-transformed p-value. The dotted line indicates nominal p=10−10.
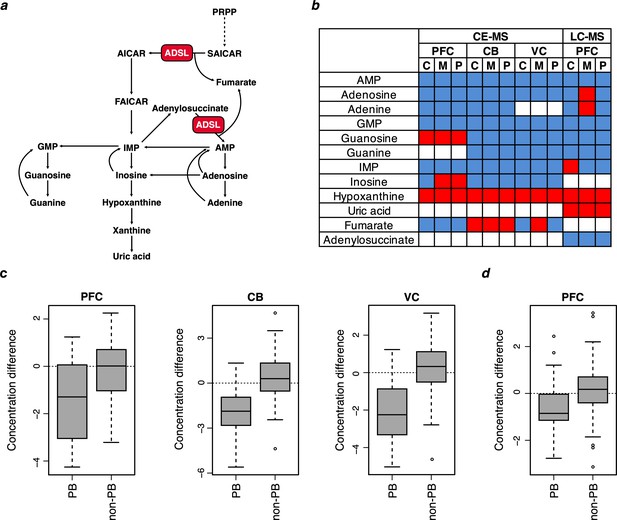
Purine metabolite levels in humans relative to chimpanzees and macaques.
(a) Pathway sketch illustrating the role of ADSL in purine biosynthesis. (b) Differences in metabolite levels between humans (n = 4) and chimpanzees (C) (n = 4), macaques (M) (n = 4) and the two non-human primates combined (P) in prefrontal cortex (PFC), cerebellum (CB), primary visual cortex (VC) analyzed by CE-MS. LC-MS measurements in PFC are from Kurochkin et al., 2019 and involved 40 humans, chimpanzees and macaques, respectively. Red and blue colors indicate higher and lower levels in humans, respectively. c and d. The distributions of metabolite differences between humans and non-human primates for purines biosynthesis (PB) (listed in panel b) and other metabolites (non-PB) detected by CE-MS (c) and LC-MS (d). The Y axis shows fold-change difference between humans and non-human primates. The metabolite concentration values are normalized and log-transformed. Wilcoxon tests for the differences between PB and non-PB distributions: p=0.049 (PFC), p=0.002 (CB), p=0.001 (VC), and p=0.019 (PFC LC-MC).

ADSL protein sequence.
Partial amino acid sequences of ADSL. Accession numbers and amino acid sequences are from NCBI. X-ray structural determination of ADSL (DOI: 10.2210/pdb4FFX/pdb) is from Ray et al., 2012. Arrow indicates position 429.
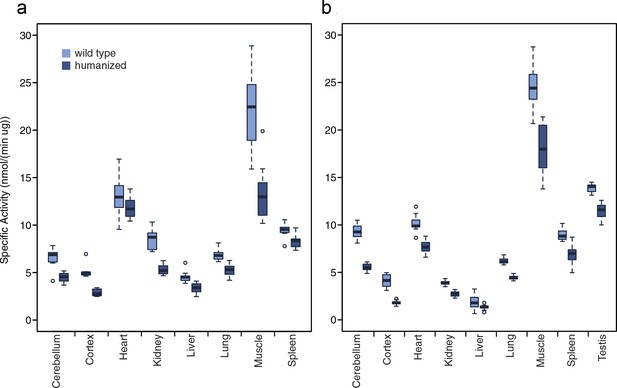
ADSL activity in tissues from humanized and wild type mice.
(a) One-week-old mice. (b) Twelve-week-old mice. Conversion of adenylosuccinate to AMP was monitored by measuring λ 282 nm absorbance over 20 min (see Materials and methods). Boxes represent upper and lower quartiles, lines extending from the boxes represent the ranges of the data, excluding outliers which are indicated by dots.
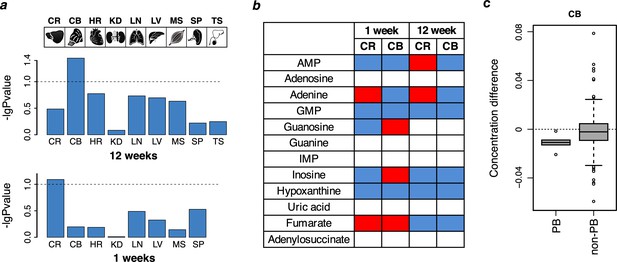
Purine biosynthesis in mice ‘humanized’ for ADSL.
(a) Data overview displaying -log10 transformed p-values of the metabolite differences between humanized and wild-type one-week (n = 10–12 per group) and 12-weeks-old (n = 9–12 per group) animals based on all detected metabolites (permutation test based on the Student’s test output). The tissues analyzed are: brain cortex (CR), cerebellum (CB), heart (HR), kidney (KD), lung (LN), liver (LV), muscle (MS), spline (SP), and testis (TS, 12 weeks only). Dotted lines indicate p=0.1. (b) The direction of purine metabolite differences in pairwise comparisons between humanized and wild type mice in the cortex (CR) and cerebellum (CB). Red and blue colors marks higher and lower levels in the humanized mice, respectively, white indicates non-detected metabolites. (c) The distributions of metabolite differences between 12-week-old humanized and wild-type mice for purines biosynthesis (PB) (listed in panel b) and the other metabolites (non-PB) detected using GC-MS in CB of 12-week-old mice (Wilcoxon test, p=0.04).
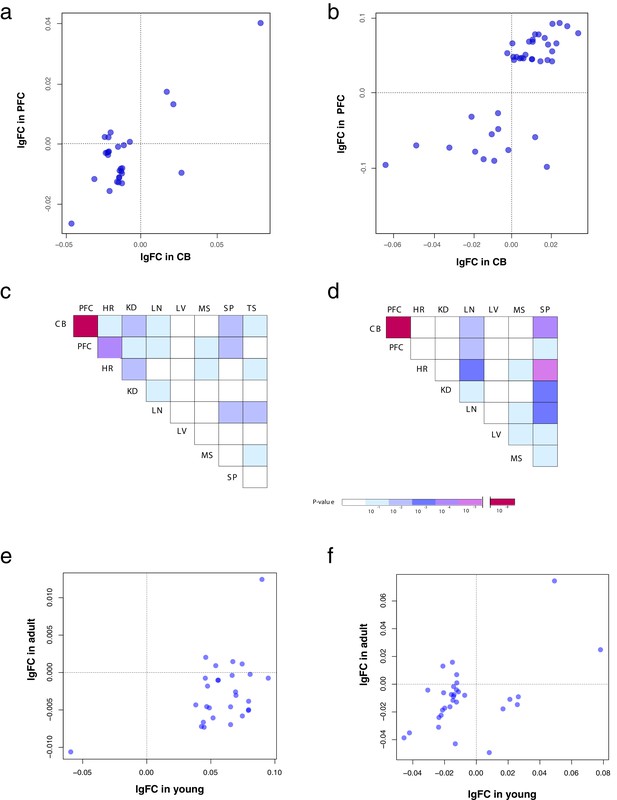
Correlations of metabolite differences in mouse tissues.
(a) Correlation between CB and PFC of metabolite differences (given as log fold change) between wild-type mice and mice carrying the V429A and R428Q substitutions in the ADSL protein in 12-week-old mice. (b) The same correlations in 1-week-old mice. (c, d) Correlations of metabolite intensity differences between all pairs of tissues in 12-week-old (c) and 1-week-old (d) mice. (e) Correlation of metabolite differences between 1-week- and 12 week-old mice in cerebral cortex. (f) Correlation of in metabolite differences between 1-week-old and 12-week-old mice in cerebellum.
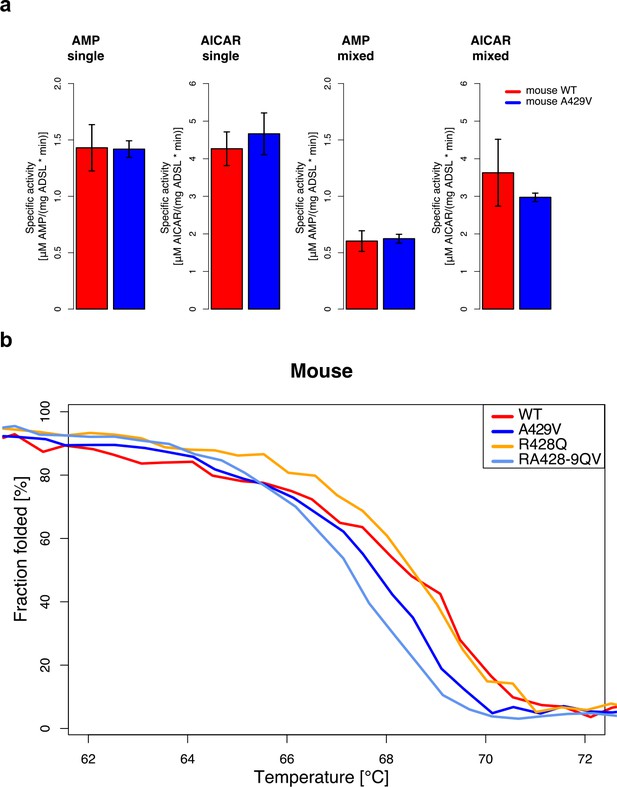
Characterization of mouse ADSL forms.
(a) Enzyme kinetics tested in substrate excess for the products (AICAR and AMP, see text above the plots) with one substrate (single) or with both substrates (mixed) in the reaction mix. Specific activities of wild type and humanized A429V versions do not differ (t-test, p>0.05). Error bars represent standard error of the mean from three experiments using two batches of proteins. (b) Protein melting measured by CD at 222 nm for WT, A429V, R428Q, and RA428-9QV ADSL versions. Lines represent the averages over four experiments. The A429V (t-test, p=0.10) and RA428-9QV versions are less stable than the other proteins (t-test, p=0.03, at denaturation midpoint (dm). dm(WT)=68.3 +/- 0.2°C, dm(A429V)=67.9 +/- 0.2°C, dm(R428Q)=68.6 +/- 0.0°C, and dm(RA428-9QV)=67.3 +/- 0.3°C).
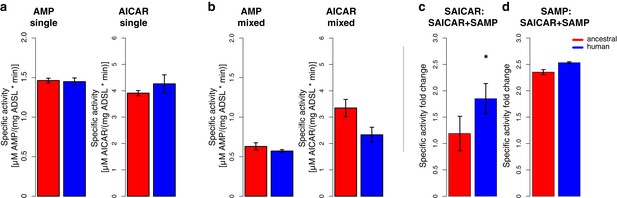
Specific activity and cooperativity of human and ancestral ADSL versions.
Specific activity of SAMP to AMP and SAICAR to AICAR catalysis for the Neandertal-like and modern human forms of ADSL when incubated (a) with the required substrate separately (‘single’) (t-test, p>0.05); (b) and with both substrates (SAICAR and SAMP) together (‘mixed’) (t-test, p>0.05). (c, d) The ratios of single substrate to mixed substrate specific activities for the ancestral and human versions of the enzyme. The human version shows a higher reduction in specific activity for SAICAR to AICAR catalysis in the presence of both substrates than the ancestral version (t-test, p=0.01) (c) which is not seen for SAMP to AMP conversion (d) (t-test, p=0.49). Error bars represent standard errors among three experiments.
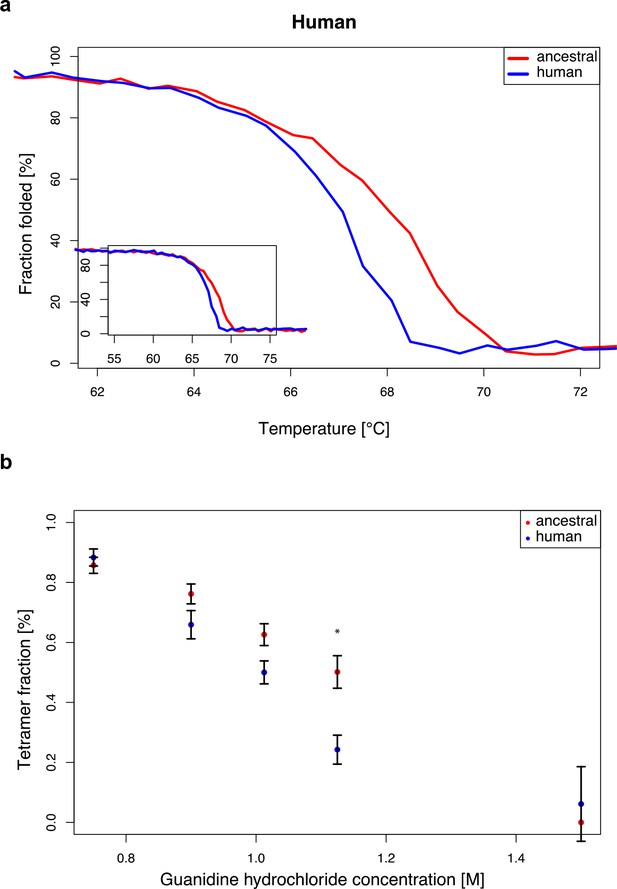
Stability of modern human and Neandertal-like ADSL.
(a) Thermal denaturation measured by CD at 222 nm for 55–75°C (plot insert) and enlarged for 62–72°C (bigger panel) showing lower stability of the modern human ADSL at the denaturation midpoint (t-test, p=0.001. dm(ancestral)=68.0 +/- 0.2°C, dm(human)=67.0 +/- 0.1°C). Data represents the average of five experiments. (b) Chemical denaturation measured by native gel electrophoresis (p=0.024 for 1.125M Gdn-Hcl concentration, t-test with Bonferroni correction). Plot represents average amount of tetrameric protein over five experiments. Error bars represent standard errors.
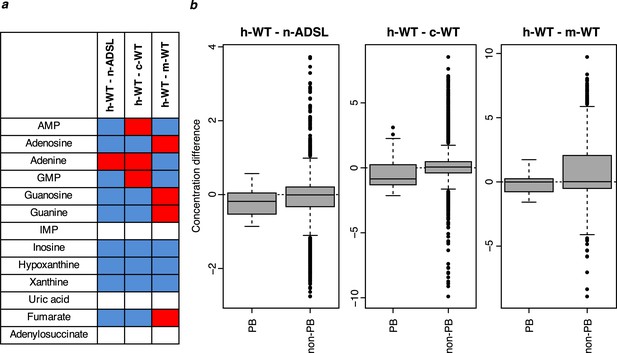
Purine biosynthesis in human cells carrying the ADSL V429A substitution as well as in human, chimpanzee, and macaque wild type cells.
(a) The direction of differences in metabolite levels in pairwise comparisons between wild type human cells (h-WT, 19 cultures of three cell lines) and human cells carrying the ADSL V429A substitution (n-ADSL, 10 cultures of three cell lines), chimpanzee cells (c-WT, nine cultures from three cell lines) and macaque cells (m-WT, three cultures from one cell line). Red and blue colors mark higher and lower metabolite levels in human wild-type cells, respectively, white indicates non-detected metabolites. (b) Distributions of differences in metabolite levels between h-WT cells and n-ADSL, c-WT, and m-WT cells, respectively, for purines biosynthesis (PB) metabolites (listed in panel a) and other metabolites (non-PB) Wilcoxon test, p=0.06 (h-ADSL), p=0.01 (c-WT), and p=0.04 (m-WT).
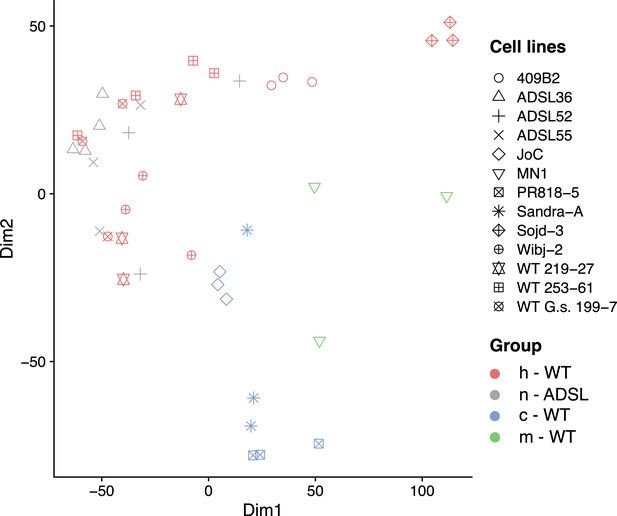
Principle component analysis of cell line metabolite composition.
The analysis is based on 8849 detected metabolites. Symbols indicate cell lines; colors indicate the group of origin. Identical symbols represent independently grown and harvested cell line clones or clone pools. The human wild-type cell line Sojd-3 was excluded from further analysis.
Additional files
-
Supplementary file 1
The MS signal intensities of metabolites detected by CE-MS in five tissues of four humans, four chimpanzees, and four rhesus macaques, reflecting their relative concentrations.
- https://cdn.elifesciences.org/articles/58741/elife-58741-supp1-v2.xlsx
-
Supplementary file 2
Metabolites showing significantly higher intensities in the human cerebellum compared to chimpanzees and rhesus macaques (t-test, adjusted p<0.05).
The table includes adjusted p-values and fold-change values for human-chimpanzee and human-macaque comparisons.
- https://cdn.elifesciences.org/articles/58741/elife-58741-supp2-v2.xlsx
-
Supplementary file 3
Pathway enrichment in metabolites showing higher levels in human tissues compared to chimpanzees and rhesus macaques.
The table includes pathway names, adjusted and nominal p-values, number of differential metabolites, and number of associated genes. Top pathways (corrected enrichment p-value<0.05) are shaded in green. Tables include multiple worksheets – one for each tissue.
- https://cdn.elifesciences.org/articles/58741/elife-58741-supp3-v2.xlsx
-
Supplementary file 4
Metabolites showing significantly lower intensities in the human prefrontal cortex compared to chimpanzees and rhesus macaques (t-test, adjusted p<0.05).
The table includes adjusted p-values and fold-change values for human-chimpanzee and human-macaque comparisons.
- https://cdn.elifesciences.org/articles/58741/elife-58741-supp4-v2.xlsx
-
Supplementary file 5
Pathway enrichment in metabolites showing lower levels in human tissues compared to chimpanzees and rhesus macaques.
The table includes names, adjusted and nominal p-values, number of differential metabolites, and number of associated genes. Top pathways (corrected enrichment p-value<0.05) are shaded in green. Tables include multiple worksheets – one for each tissue.
- https://cdn.elifesciences.org/articles/58741/elife-58741-supp5-v2.xlsx
-
Supplementary file 6
Mouse sample information.
Table includes sample information for two age groups of wild type and humanized mice: one week and 12 weeks of age. Eight tissues were sampled for both age groups. Testes were additionally sampled for 12-weeks-old mice. Outlier samples are shaded in red. Table includes multiple worksheets – one for each age group.
- https://cdn.elifesciences.org/articles/58741/elife-58741-supp6-v2.xlsx
-
Supplementary file 7
Normalized intensities of metabolites detected using GC-MS in eight tissues of 1-week-old humanized and wild-type mice.
The table includes multiple worksheets – one for each tissue.
- https://cdn.elifesciences.org/articles/58741/elife-58741-supp7-v2.xlsx
-
Supplementary file 8
Normalized intensities of metabolites detected using GC-MS in eight tissues of 12-weeks-old humanized and wild-type mice.
The table includes multiple worksheets – one for each tissue.
- https://cdn.elifesciences.org/articles/58741/elife-58741-supp8-v2.xlsx
-
Supplementary file 9
Metabolites showing significant intensity differences between humanized and wild-type mice.
Table lists 45 metabolites showing significant intensity differences in the prefrontal cortex (PFC) at one week of age and 36 showing significant intensity differences in the in cerebellum (CB) at 12 weeks of age. Table includes multiple worksheets – one for each tissue.
- https://cdn.elifesciences.org/articles/58741/elife-58741-supp9-v2.xlsx
-
Supplementary file 10
Normalized intensities of metabolites detected using LC-MS in cell lines.
The table includes multiple worksheets: cell line metadata, intensities of all detected metabolite peaks, peak annotation according to KEGG database.
- https://cdn.elifesciences.org/articles/58741/elife-58741-supp10-v2.xlsx
-
Transparent reporting form
- https://cdn.elifesciences.org/articles/58741/elife-58741-transrepform-v2.pdf