Scanned optogenetic control of mammalian somatosensory input to map input-specific behavioral outputs
Figures
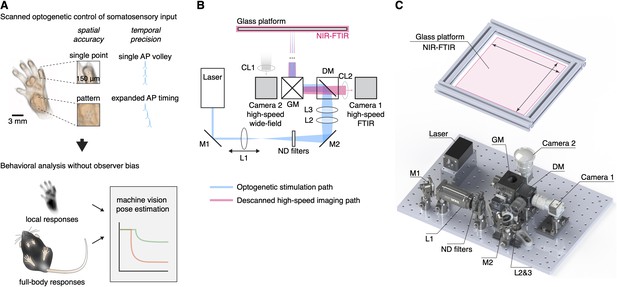
Remote and precise somatosensory input and analysis of behavior.
(A) Afferent neurons expressing ChR2 are controlled remotely in freely behaving mice by projecting laser light with sub-millimeter precision to the skin. This enables precise non-contact stimulation with microscale patterns, lines, and points using scanned transdermal optogenetics. Time-locked triggering of single-action potential volleys is achieved through high temporal control of the laser. Behavioral responses can be automatically recorded and analyzed using a combination of computational methods. (B) Schematic of the stimulation laser (in blue) and infrared imaging (in red) paths. Mirrors (M1 and M2) direct the laser beam through a set of lenses (L1–L3), which allow the beam to be focused manually to pre-calibrated spot sizes. A dichroic mirror (DM) guides the laser beam into a pair of galvanometer mirrors, which are remotely controlled to enable precise targeting of the beam onto the glass platform. Near-infrared frustrated total internal reflection (NIR-FTIR) signal from the glass platform is descanned through the galvanometers and imaged using a high-speed infrared camera. A second wide-field camera is used to concomitantly record a below view of the entire glass platform. (C) Rendering of the assembled components. A Solidworks assembly is available at https://github.com/browne-lab/throwinglight.
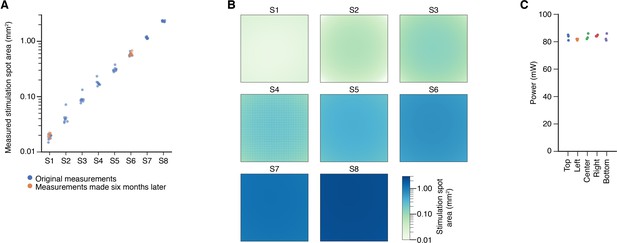
Technical calibration of the optical system.
(A) Average spot areas calculated from triplicate measures taken at nine distinct coordinates of the experimental glass platform. The stability of spot size area over time was demonstrated by re-sampling area measurements for S1 and S6 6 months after extensive use of the system (orange). (B) Uniformity of laser spot area across the surface of the experimental glass platform. Heatmaps of average areas for spot sizes S1 to S8 as measured in triplicates at nine distinct coordinates covering the entire glass platform and fitted with a two-dimensional polynomial equation. (C) Uniformity of laser power across the glass platform was demonstrated by measuring laser power in triplicates at five distinct locations using spot size S1 and 100 mW laser output.
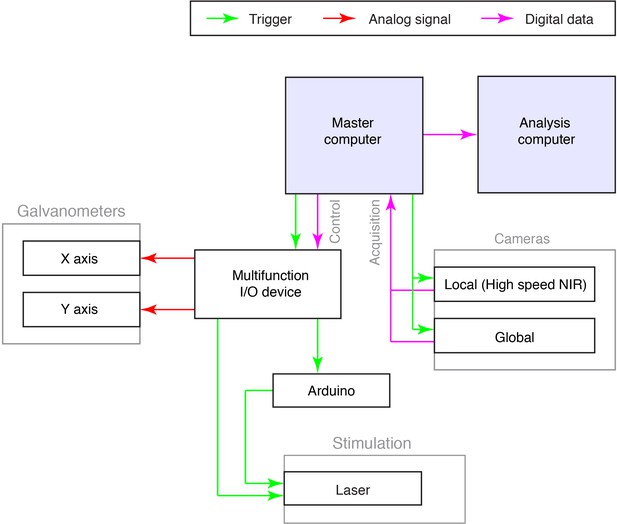
Hardware and software information flow used in the optical system.
Schematic illustrating the information flow between the system’s operating software and the different hardware components. A master computer allows user input to be transformed into digital signals, which are fed into a multifunction I/O device to coordinate the triggering of the laser, cameras, and analog control of the galvanometers. The same computer is used to record high-speed paw and full-body behaviors acquired through two separate cameras. Automated analysis is performed offline.
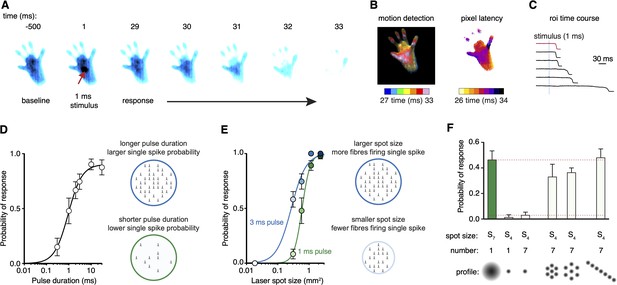
Scanned optogenetic stimuli reveal relationships with local behaviors.
(A) Millisecond-timescale changes in hind paw near-infrared frustrated total internal reflection (NIR-FTIR) signal in response to a single 1 ms laser pulse (laser spot size S6 = 0.577 mm2) recorded at 1000 frames/s. (B) Motion energy analysis (left) and response latencies calculated for each pixel (right) for the same trial as in (A). (C) Example traces of the NIR-FTIR signal time course as measured within a circular region of interest centered on the stimulation site. Six traces from two animals are depicted (1 ms pulse, spot size S6 = 0.577 mm2). The red trace corresponds to the example trial illustrated in (A) and (B). (D) Paw response probability increases as a function of laser pulse duration when stimulation size is constant (spot size S6 = 0.577 mm2; 37–42 trials for each pulse duration from eight mice, mean probability ± SEM). Light pulses 10 ms or less with the same intensity and wavelength have been shown to generate just a single-action potential in each nociceptor activated in the TRPV1Cre::ChR2 line (Browne et al., 2017). Note that a 30 ms might generate more than one action potential but the response already plateaus at 10 ms duration, suggesting one action potential per nociceptor shapes the response. (E) Paw response probability increases as a function of laser stimulation spot size when pulse duration is constant. Data are 34–45 trials for each spot size per pulse duration from 7 to 8 mice, shown as mean probability ± SEM. The dataset for (D) and (E) is provided in Figure 2—source data 1. (F) Stimulation patterning shows that the absolute size, rather than the geometric shape, of the nociceptive stimulus determines the withdrawal probability (Friedman’s non-parametric test for within subject repeated measures S(5) = 22.35, p=0.0004). Paw response probabilities in response to a single large laser spot (S7 = 1.15 mm2), a single small spot (S4 = 0.176 mm2; p=0.018 compared to S7 and p=0.013 compared to the line pattern), a 10 ms train of seven small 1 ms spots targeting the same site (p=0.039, compared to S7 and p=0.030 compared to the line pattern) or spatially translated to produce different patterns. Note that the cumulative area of the seven small spots approximates the area of the large spot, and no statistically significant difference was detected between any of their response probabilities. Data shown as mean probability ± SEM are from n = 6 mice, with each 6–10 trials per pattern. The dataset for (F) is provided in Figure 2—source data 2.
-
Figure 2—source data 1
Time courses of paw movement recorded at 1000 frames/s with stimuli that vary in duration and size.
Stimuli (40 mW/mm2) were delivered at 0 ms. Data are from TRPV1Cre::ChR2 mice and littermate controls.
- https://cdn.elifesciences.org/articles/62026/elife-62026-fig2-data1-v2.csv
-
Figure 2—source data 2
Time courses of paw movement recorded at 500 frames/s with single point and patterned stimuli.
Stimuli (1 ms, 40 mW/mm2) were delivered at 0 ms. Data are from TRPV1Cre::ChR2 mice and littermate controls.
- https://cdn.elifesciences.org/articles/62026/elife-62026-fig2-data2-v2.csv
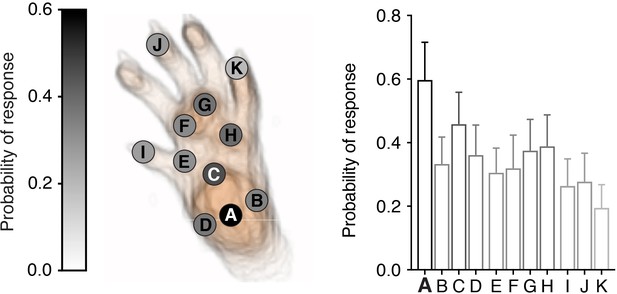
Microscale mapping of sensitivity to noxious optogenetic stimulation.
Paw response probabilities at 11 discrete 0.0185 mm2 stimulation locations across the hind paw glabrous skin using single-pulse stimulations (3 ms) in n = 8 mice. Response probabilities were determined manually.

Littermate controls do not respond to optogenetic stimulation.
(A) Examples of the near-infrared frustrated total internal reflection hind paw signal before, during, and after laser stimulation (arrow). (B) Examples of bottom-view camera recordings before, during, and after laser stimulation. (C) Raster plots of hind paw dynamics in response to a single 30 ms pulse (spot size S8 = 2.307 mm2) in TRPV1Cre::ChR2 mice (32 trials from seven mice) and littermate controls (16 trials from four mice). Where applicable, the paw response latency is indicated in red.
Pain-related hind paw withdrawals.
Millisecond-timescale changes in hind paw near-infrared frustrated total internal reflection signal in response to a single 1 ms laser pulse (laser spot size S5 = 0.577 mm2) recorded at 1000 frames/s. Six individual trials from two different TRPV1Cre::ChR2 mice are shown.
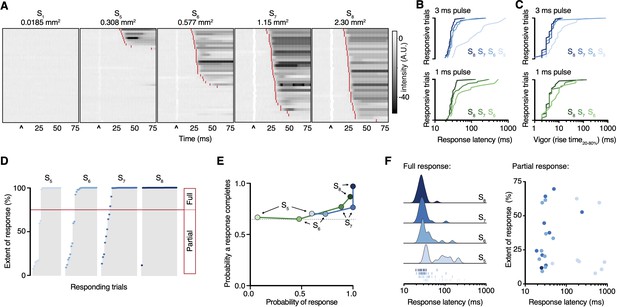
Paw response latency and magnitude are influenced by the sparse recruitment of nociceptors.
(A) Raster plots of hind paw responses for five different 3 ms laser stimulation sizes, sorted by response latency. The paw response latency is indicated in red. (B) Paw response latencies to trials with single 3 ms (blue, top) and 1 ms (green, bottom) stimulations at different spot sizes, sorted by latency. (C) Response vigor (hind paw rise time, 20–80%) to single 3 ms (blue, top) or 1 ms (green, bottom) pulses with a range of stimulation spot sizes. Rise times to a 3 ms pulse were 4 ± 1 ms, 4 ± 1 ms, 4 ± 1 ms, and 9 ± 5 ms for spot sizes S8, S7, S6, and S5, respectively, and to a 1 ms pulse were 4 ± 1 ms, 5 ± 2 ms, and 6 ± 3 ms for spot sizes S8, S7, and S6, respectively. (D) Extent of responses (%NIR-FTIR signal decrease). The threshold for a full response and partial response is 75% of baseline signal (red line). (E) The probability of responses to reach completion (full response) as a function of the probability of response for four stimulation spot sizes and two pulse durations (green 1 ms; blue 3 ms). (F) Response latency distributions for trials that reach completion (full response) shown with Gaussian kernel density estimation of data (left). Rug plot inset representing individual response latencies for each color-coded spot size. No correlation was observed between response latency and extent for partial responses when stimulation duration was 3 ms. Data from 7 to 8 mice with 39–44 trials per spot size for 1 ms pulse duration and 34–44 per spot size for 3 ms pulse duration. The dataset is provided in Figure 2—source data 1. NIR-FTIR: near-infrared frustrated total internal reflection.
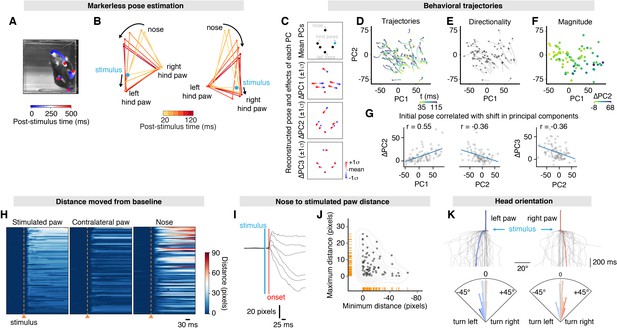
Mapping whole-body behavioral repertoires to precise nociceptive input.
(A) Example spatiotemporal structure of a noxious stimulus response superimposed on the baseline image taken immediately before stimulus. The color indicates the timing of nose and hind paw trajectories. In this example, the left hind paw of the mouse was stimulated, which is the right hind paw as viewed in the image. For ease, we refer to the stimulation side as viewed in the image, rather than the side with respect to the mouse. (B) Example graphical representation showing the sequence of postural adjustment following nociceptive stimulus in two trials. Left: the left (as viewed) hind paw was stimulated. Right: the right (as viewed) hind paw was stimulated. (C) Principal component analysis of the x and y values for six body parts – nose, left hind paw digits, left hind paw heel, right hind paw digits, right hind paw heel, and tail base – across all 80 trials. Coordinates were egocentrically aligned by the baseline pose, setting the tail base as origin and the stimulated paw on the right. This allowed the reconstruction of these locations using the first three principal components (PCs). Using the mean values of PC1, PC2, and PC3 with the stimulated hind paw indicated in blue (top); the mean values of PC2 and PC3, while varying PC1 either side of its mean by one standard deviation (middle-top); the mean values of PC1 and PC3, while varying PC2 (middle-bottom); and the mean values of PC1 and PC2, varying PC3 (bottom). (D) Behavioral trajectories of the 80 trials in PC space, showing 35–115 ms after stimulation. Only the first two PCs are shown for clarity. (E) PC vectors based on (D) show that trajectories are largely in the same direction. (F) The response magnitude (shown by colors that represent shift in PC2) varies as a function the initial pose, reduced to the first two PCs. (G) The initial PC values correlate with the shift in PC2 (left three plots). The initial PC3 value also correlates with the shift in PC3 (right). Least-squares linear fits are shown in blue and r values are Pearson’s correlation coefficients. (H) Raster plots of the distances that each tracked body part moves relative to baseline in 80 trials from 10 mice. All raster plots are sorted by maximum distances achieved by the stimulated paw within 300 ms of the stimulation. (I) Six representative traces showing the Euclidean distance between the stimulated paw and nose. (J) This expansion and shortening of Euclidean distance between the stimulated paw and the nose are shown up to 300 ms post-stimulus for all 80 trials by plotting the maximum distances as a function of the minimum distance. Corresponding rug plots (orange ticks) and a kernel density estimate (gray lines) are shown. (K) Traces showing the angle of the nose normalized to mean baseline angle between the nose and tail base. The tail base reflects the origin in these calculations. 80 trials are shown, with stimulation on the left hind paw and right hind paw (top). Average traces are shown in blue and red for left and right hind paw stimulations, respectively. Polar histograms for mean nose yaw during 300 ms post-stimulus, corresponding to the traces directly above (below). The dataset is provided in Figure 4—source data 2.
-
Figure 4—source data 1
Whole-body motion energy recorded at 40 frames/s with different size stimuli.
Stimuli (3 ms, 40 mW/mm2) were delivered at 0 ms. Data are from TRPV1Cre::ChR2 mice.
- https://cdn.elifesciences.org/articles/62026/elife-62026-fig4-data1-v2.csv
-
Figure 4—source data 2
Time courses for coordinates of six tracked body parts recorded at 400 frames/s.
Stimuli (10 ms, 40 mW/mm2, laser spot size S8 = 2.307 mm2) were delivered at 0 ms and body parts tracked with DeepLabCut. Data are from TRPV1Cre::ChR2 mice.
- https://cdn.elifesciences.org/articles/62026/elife-62026-fig4-data2-v2.csv
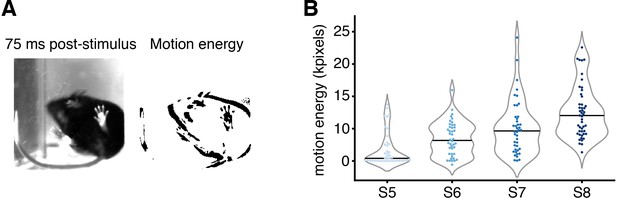
Motion energy analysis of behavior evoked by precisely controlled nociceptive input size.
(A ) Left: example image from the below-view camera, recording whole-body behavior at 40 frames/s, 75 ms after stimulus delivery (3 ms pulse, spot size S6 = 0.577 mm2). (Right) Motion energy calculated 75 ms after the stimulus. (B) Motion energy increases with larger spot sizes when pulse duration is kept constant at 3 ms. Violin plots with 41–47 trials per spot size from eight mice. Individual trials are shown, along with the associated median in black. The dataset is provided in Figure 4—source data 1.
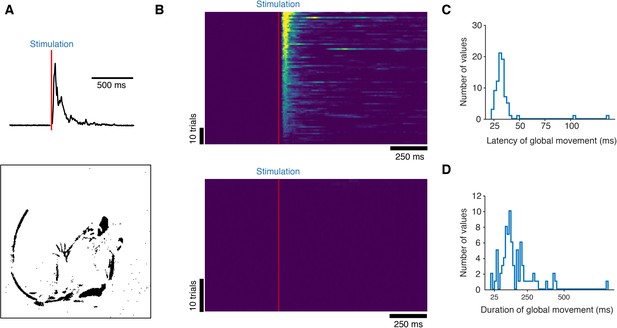
Motion energy analysis of high-speed recordings.
(A) Example motion energy trace acquired from 400 fps videos (top). The stimulus time is shown in red. Example image of animal motion detected by subtraction of neighboring frames (bottom). Pixels that change intensity are shown in black. (B) Raster plots of the motion energy time course from 10 TRPV1Cre::ChR2 mice from two litters (top; 80 trials from 10 mice) and control littermate mice from the same two litters (bottom; 40 trials from 5 mice). Trials are sorted according to their maximum peak response. The red vertical line represents stimulus. (C) Histogram of latencies for stimulus-evoked full-body movements. Latencies were detected at time points when motion energy pixel counts exceeded 10 times standard deviation of the mean baseline signal. (D) Histogram of the duration of stimulus-evoked full-body movements. Termination of movement was detected when motion energy pixel counts returned below 10 times standard deviation of baseline signal.
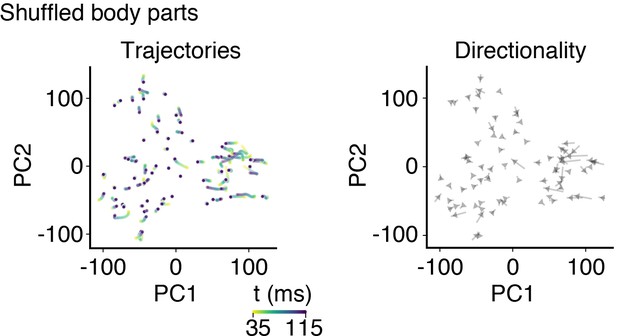
Principal component analysis of shuffled behavioral data.
Body part labels were shuffled on each trial and dimensionality reduction carried out identically as for non-shuffled data in Figure 4. The first principal component (PC1) explained 22.2% of variance, the second (PC2) 16.4%, and the third (PC3) 13.5%. Left: shuffled data show trajectories that were static compared to non-shuffled data. Right: trajectories were not uniformly directional.
Markerless tracking of behavior in response to nociceptive stimulation.
Markerless tracking of postural adjustments in a TRPV1Cre::ChR2 mouse, in response to a 10 ms optogenetic noxious stimulation (laser spot size S8 = 2.307 mm2). Body parts are labeled with multicolor points and the hind paws and nose connected with magenta lines. The time shown is relative to the stimulus onset.
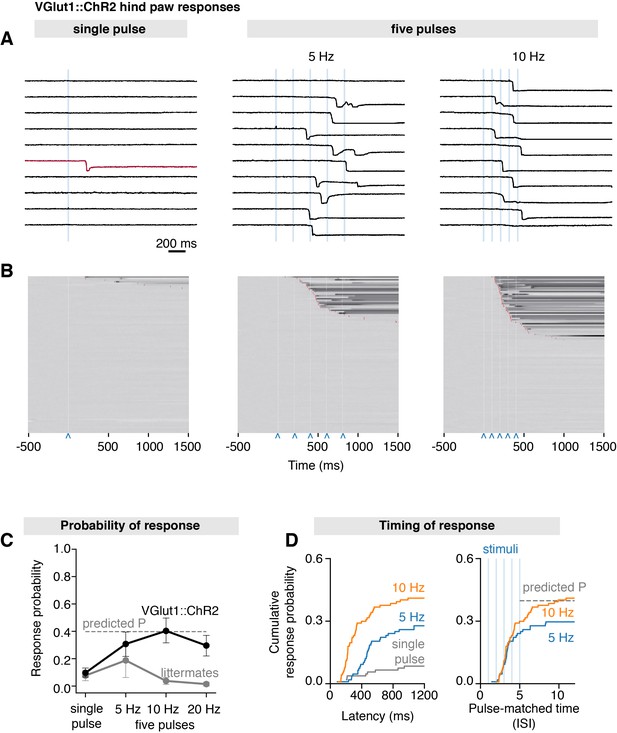
Scanned transdermal optogenetic activation of Aβ-LTMRs triggers slow-onset responses.
(A) Example traces of the near-infrared frustrated total internal reflection signal time course for three different stimulation protocols in Vglut1Cre::ChR2 mice: single pulse, five pulses at 5 Hz, and five pulses at 10 Hz (pulse duration 3 ms, spot size S7 = 1.155 mm2). (B) Corresponding raster plots of hind paw responses sorted by latency. The paw response latency is indicated in red (99–103 trials/protocol from n = 11–12 mice) and the 3 ms laser stimuli shown with blue carets. (C) Paw response probability peaks at 10 Hz stimulation frequency in Vglut1Cre::ChR2 mice (pulse duration 3 ms, spot size S7 = 1.155 mm2; 99–103 trials/protocol from n = 11–12 mice, mean probability ± SEM). (D) Left panel: paw response latencies in trials with a single 3 ms stimulation or with trains of five 3 ms stimuli at 5 Hz or at 10 Hz. Right panel: paw response latencies normalized to the interstimulus interval. The estimated probability in (C) and (D) (dashed gray lines) was calculated using P(X ≥ 1) = 1–(1–p)n, where p is the probability of a response on a single pulse (0.096) and n is the number of pulses (5). The dataset is provided in Figure 5—source data 1.
-
Figure 5—source data 1
Time courses of paw movement recorded at 1000 frames/s with stimuli that vary in frequency.
Stimuli (3 ms, laser spot size S7 = 1.181 mm2, 40 mW/mm2) were delivered at 0 ms. Data are from Vglut1Cre::ChR2 mice and littermate controls.
- https://cdn.elifesciences.org/articles/62026/elife-62026-fig5-data1-v2.csv
-
Figure 5—source data 2
Whole-body motion energy recorded at 400 frames/s with stimuli that vary in frequency.
Stimuli (3 ms, laser spot size S7 = 1.181 mm2, 40 mW/mm2) were delivered at 0 ms. Data are from Vglut1Cre::ChR2 mice.
- https://cdn.elifesciences.org/articles/62026/elife-62026-fig5-data2-v2.csv
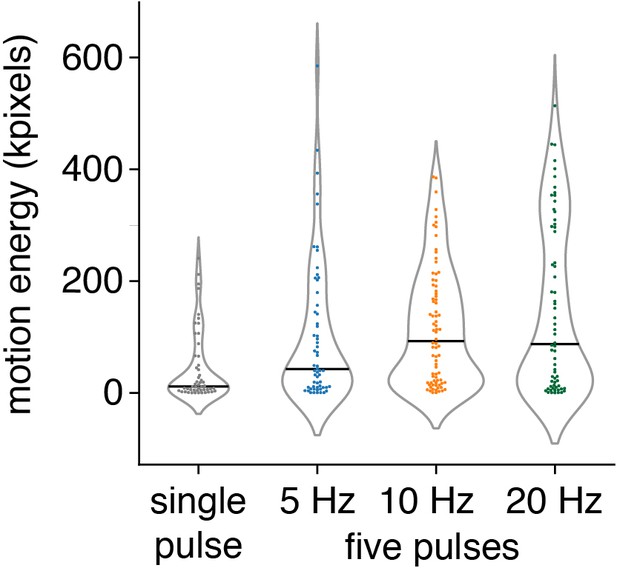
Motion energy analysis of full-body behavior-evoked Aβ-LTMRs.
Motion energy is not affected by stimulation frequency single 3 ms pulse or five pulses of 3 ms at 5 Hz, 10 Hz, or 20 Hz; spot size S7 = 1.155 mm2. Violin plots with 51–80 trials per protocol from 11 to 12 mice. Individual trials are shown, along with the associated median in black. The dataset is provided in Figure 5—source data 2.
Tables
Reagent type (species) or resource | Designation | Source or reference | Identifiers | Additional information |
---|---|---|---|---|
Genetic reagent (Mus musculus) | R26-CAG-LSL-hChR2(H134R)-tdTomato (Ai27D) | Jackson Laboratory | Stock #: 012567RRID: IMSR_JAX:012567 | PMID:22446880 |
Genetic reagent (M. musculus) | Trpv1-IRES-Cre (TRPV1Cre) | Jackson Laboratory | Stock #: 017769RRID: IMSR_JAX:017769 | PMID:21752988 |
Genetic reagent (M. musculus) | Slc17a7-IRES2-Cre-D(Vglut1Cre) | Jackson Laboratory | Stock #: 023527RRID: IMSR_JAX:023527 | PMID:21752988 |
Software, algorithm | RStudio | RStudio http://www.rstudio.com/ | RRID:SCR_000432 | Version 1.2.5019 |
Software, algorithm | Python | Python http://www.python.org/ | RRID:SCR_008394 | Version3.6.8 |
Software, algorithm | Fiji | Fiji http://fiji.sc | RRID:SCR_002285 | Version2.0.0 |
Software, algorithm | Prism 7 | GraphPad Prism http://www.graphpad.com/ | RRID:SCR_002798 | Version 7 |
Software, algorithm | Seaborn | Seaborn http://www.seaborn.pydata.org | RRID:SCR_018132 | |
Software, algorithm | Adobe Illustrator | Adobe http://www.adobe.com | RRID:SCR_010279 | Version 24.0 |
Additional files
-
Supplementary file 1
List of components for the assembly of the optical system.
List of parts used in system. A Solidworks assembly, the optical system control and acquisition software, and behavioral analysis toolkit are available at https://github.com/browne-lab/throwinglight (Schorscher-Petcu and Browne, 2020).
- https://cdn.elifesciences.org/articles/62026/elife-62026-supp1-v2.docx
-
Transparent reporting form
- https://cdn.elifesciences.org/articles/62026/elife-62026-transrepform1-v2.pdf