Brain endothelial cell TRPA1 channels initiate neurovascular coupling
Figures
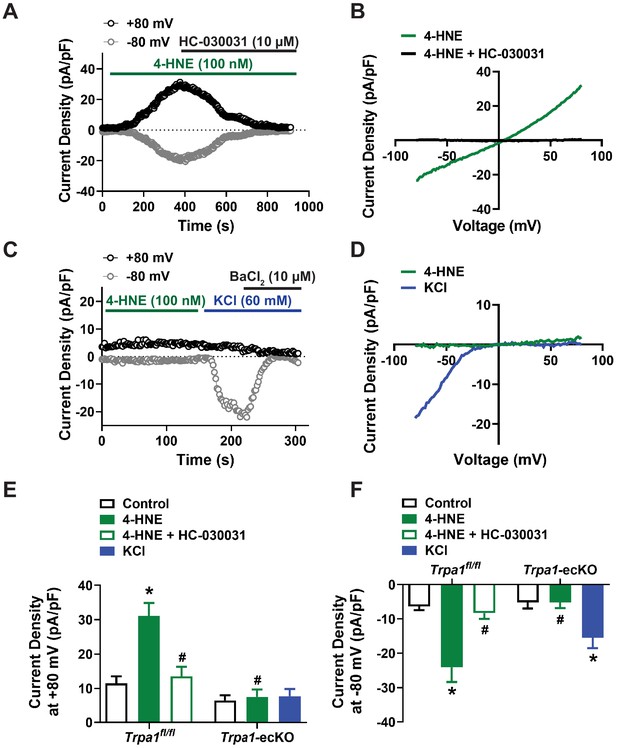
TRPA1 channels are functionally expressed in native brain capillary endothelial cells.
(A and B) Representative current versus time trace (A) and I-V relationship (B) from a whole-cell patch-clamp electrophysiology experiment demonstrating that the TRPA1 activator 4-HNE (100 nM) elicited a current in a native capillary endothelial cell isolated from a control Trpa1fl/fl mouse that was blocked by the selective TRPA1 antagonist HC-030031 (10 µM). (C and D) Representative current versus time trace (C) and I-V relationship (D) demonstrating that 4-HNE was unable to elicit a current in a native capillary endothelial cell from a Trpa1-ecKO mouse. Cell viability was confirmed by evoking Kir currents with raised extracellular KCl (60 mM) that were sensitive to BaCl2 (10 µM). (E and F) Summary data showing that the TRPA1 current produced by 4-HNE at +80 mV (E) and −80 mV (F) was blocked by HC-030031 and was absent in cells from Trpa1-ecKO mice (Trpa1fl/fl, n = 12 cells from five animals; Trpa1-ecKO, n = 8 cells from three animals; *p<0.05, one-way ANOVA).
-
Figure 1—source data 1
Individual data points and analysis summaries for datasets shown in Figure 1.
- https://cdn.elifesciences.org/articles/63040/elife-63040-fig1-data1-v2.xlsx
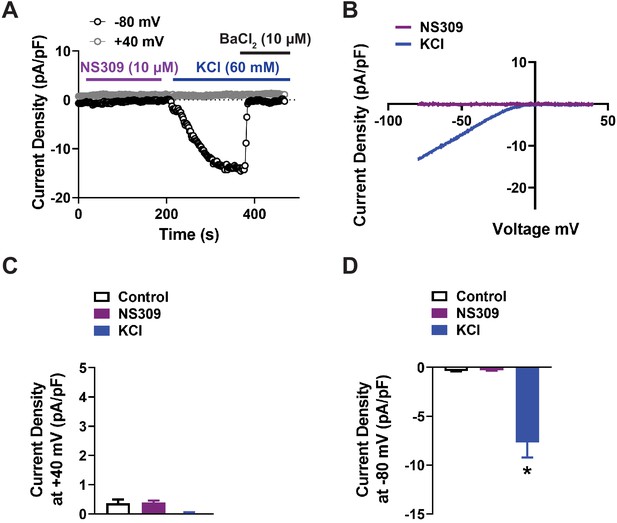
Lack of functional IK and SK channels in native capillary endothelial cells.
(A and B) Representative current-time trace (A) and I-V relationship (B) from a whole-cell patch-clamp electrophysiology experiment demonstrating that the IK and SK channel activator NS309 (10 µM) did not produce a current in isolated native capillary endothelial cells from wild-type mice. Kir currents were detected by raising extracellular KCl (60 mM) and were confirmed by assessing their sensitivity to BaCl2 (10 µM). (C and D) Summary data showing the current produced by NS309 (10 µM) and KCl (60 mM) at +40 mV (C) and −80 mV (D) (n = 6 cells from three animals; *p<0.05, one-way ANOVA).
-
Figure 1—figure supplement 1—source data 1
Individual data points and analysis summaries for datasets shown in Figure 1—figure supplement 1.
- https://cdn.elifesciences.org/articles/63040/elife-63040-fig1-figsupp1-data1-v2.xlsx
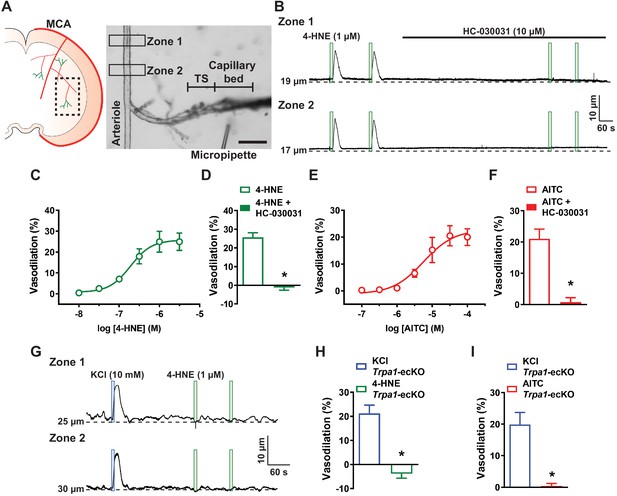
Capillary TRPA1 channels initiate conducted vasodilation in the cerebral microcirculation.
(A) Experimental illustration. Microvascular preparations were obtained from the subcortical region that is supplied by the middle cerebral artery (MCA). Representative image of an ex vivo microvascular preparation consisting of an intact parenchymal arteriole with attached capillaries. The post-arteriole transitional segment (TS) and capillaries are located at the distal end of the offshoot arteriole branch. Scale bar = 100 µm. Drugs were directly applied onto capillary extremities with a micropipette. (B) Representative traces showing that application of 4-HNE (1 µM; green box) directly onto capillary extremities produced a reproducible increase in lumen diameter in two different areas (Zone 1 and Zone 2) on the upstream arteriole segment in microvascular preparations isolated from wild-type mice. The dilation produced by 4-HNE was blocked the selective TRPA1 antagonist HC-030031 (10 µM). (C) Concentration-response curve produced by locally applying 4-HNE to capillary extremities over a concentration range of 10 nM to 30 µM (n = 6 preparations from five animals). An EC50 of 190 nM was calculated from the plot of a non-linear regression curve. (D) Summary data showing that dilation to 4-HNE (1 µM) was blocked by HC-030031 (10 µM) (n = 9 preparations from six animals; *p<0.05, paired t-test). (E) Concentration-response curve produced by locally applying the TRPA1 agonist AITC to capillary extremities over a concentration range of 100 nM to 100 µM. An EC50 of 6 µM was calculated from the plot of a non-linear regression curve (n = 6 preparations from five animals). (F) Summary data showing that dilation to AITC (30 µM) was blocked by HC-030031 (10 µM) (n = 7 preparations from five animals; *p<0.05, paired t-test). (G) Representative traces showing that application 4-HNE (1 µM; green box) onto capillary extremities was unable to dilate the upstream arteriole in microvascular preparations from Trpa1-ecKO mice, whereas application of KCl (10 mM; blue box) effectively dilated the upstream arteriole in these preparations. (H and I) Summary data showing that neither 4-HNE (1 µM) (n = 7 preparations from five animals; *p<0.05) (H) nor AITC (30 µM) (n = 7 preparations from four animals; *p<0.05, paired t-test) (I) evoked dilation of upstream arterioles in preparations from Trpa1-ecKO mice.
-
Figure 2—source data 1
Individual data points and analysis summaries for datasets shown in Figure 2.
- https://cdn.elifesciences.org/articles/63040/elife-63040-fig2-data1-v2.xlsx
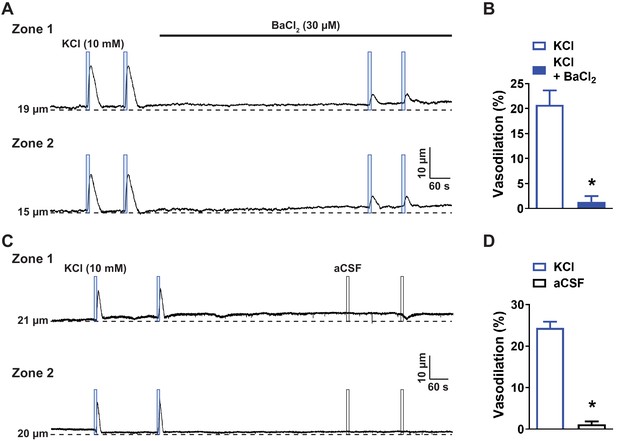
KCl-induced dilation of upstream arterioles is blocked by BaCl2.
(A) Representative traces (A) and summary data (B) showing that application of a solution containing elevated KCl (10 mM; blue box) directly onto capillary extremities induced a dilation of the upstream arteriole that was blocked by BaCl2 (30 µM) in ex vivo microvascular preparations from wild-type mice (n = 6 preparations from four animals; *p<0.05, paired t-test). (C and D) Representative trace (C) and summary data (D) showing that application of aCSF (black box) onto the capillary bed did not affect the lumen diameter of upstream arterioles (n = 6 preparations from four animals; *p<0.05, paired t-test).
-
Figure 2—figure supplement 1—source data 1
Individual data points and analysis summaries for datasets shown in Figure 2—figure supplement 1.
- https://cdn.elifesciences.org/articles/63040/elife-63040-fig2-figsupp1-data1-v2.xlsx
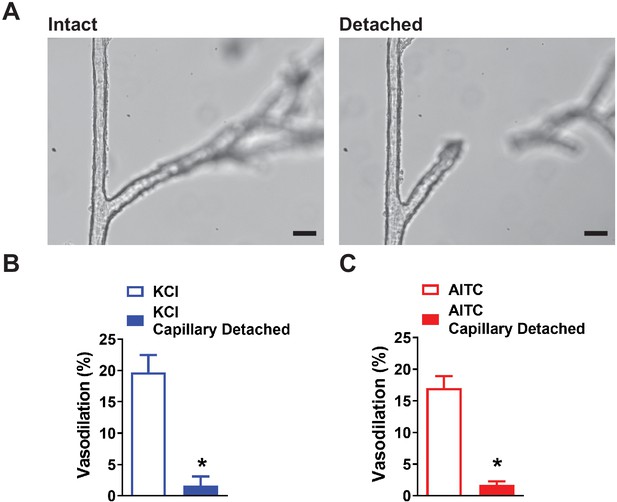
Severing the connection between capillaries and the arteriole segment of an ex vivo microvascular preparation.
(A) Representative images showing an intact microvascular preparation (left) and the same preparation after severing the connection between the arteriole and capillary (right). Scale bar = 50 µm. (B and C) Summary data demonstrating the loss of propagative dilation to elevated KCl (10 mM) (n = 7 preparations from five animals; *p<0.05, paired t-test) (B) and AITC (30 µM) (n = 7 preparations from five animals; *p<0.05, paired t-test) (C) after the connection is lost.
-
Figure 2—figure supplement 2—source data 1
Individual data points and analysis summaries for datasets shown in Figure 2—figure supplement 2.
- https://cdn.elifesciences.org/articles/63040/elife-63040-fig2-figsupp2-data1-v2.xlsx
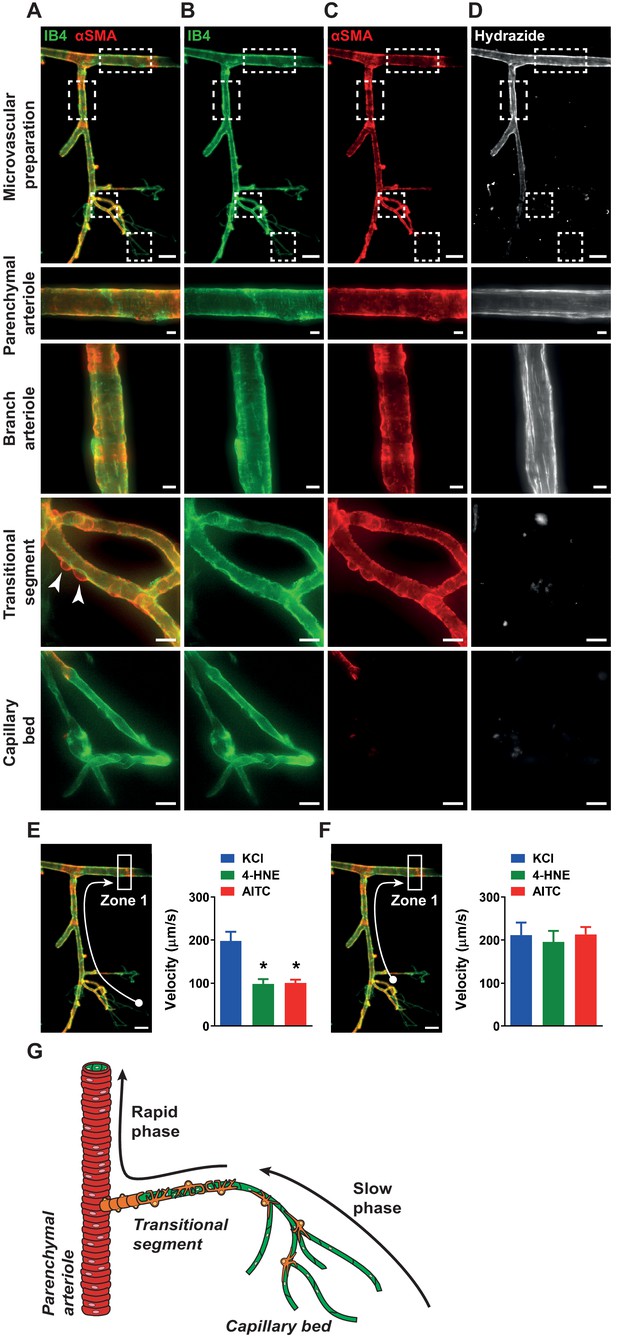
Biphasic velocity of conductive vasodilation following activation of capillary TRPA1 channels.
(A to D) Representative fluorescence image of an ex vivo microvascular preparation obtained from a wild-type mouse. Microvascular preparations were treated with Alexa Fluor 488 conjugated isolectin B4 (IB4) (green) to label endothelial cells, and Cy3 conjugated anti-α-smooth muscle actin antibody (αSMA) (red) to label mural cells, shown merged (A) or separately (B and C). The elastin layer that is only present in arteriole segments was stained using Alexa Fluor 633 conjugated hydrazide (white) (D). Scale bar = 50 µm. Magnified images of the parenchymal arteriole, offshoot branch arteriole, post-arteriole transitional segment and capillaries showing differential mural cell coverage (white arrow) and elastin expression in these vascular segments. Scale bars = 10 µm. (E) Left: Conduction velocity of the vasodilator signal was calculated from the time interval and distance traveled to a specific point in the upstream arteriole (Zone 1, white arrow) following application of drugs onto capillary extremities (white ball). Right: Responses to the TRPA1 channel activators AITC (30 µM) and 4-HNE (1 µM) were significantly slower than the response to activation of Kir channels with KCl (10 mM) (n = 6–15 preparations from four to seven animals; *p<0.05, one-way ANOVA). (F). Left: Velocity measurements were calculated from the post-arteriole transitional segment (adjacent to the capillary bed, white ball) to a point in the upstream arteriole (Zone 1, white arrow). Right: Velocity following AITC (30 µM) or 4-HNE (1 µM) treatment was comparable to that following application of KCl (60 mM) (n = 6 preparations from four animals; one-way ANOVA). (G) Proposed model showing that activation of TRPA1 channels on capillaries produces a signal that slowly propagates through the capillary network and a faster propagating signal in arterioles and the post-arteriole transitional region.
-
Figure 3—source data 1
Individual data points and analysis summaries for datasets shown in Figure 3.
- https://cdn.elifesciences.org/articles/63040/elife-63040-fig3-data1-v2.xlsx
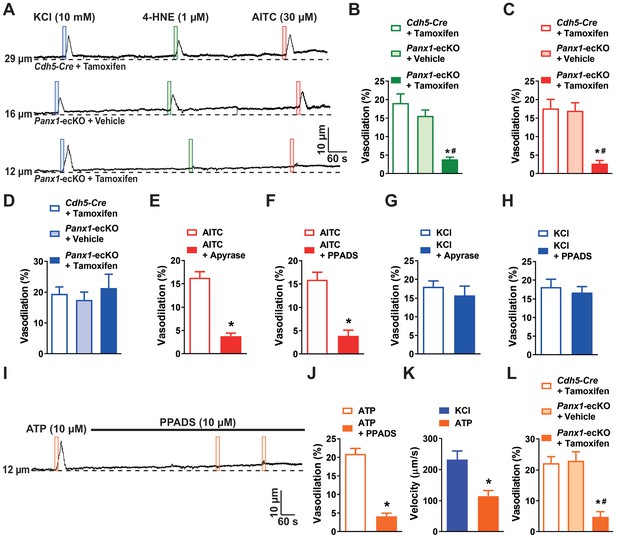
Activation of capillary TRPA1 channels produces a purinergic signal that travels through the capillary bed.
(A) Representative traces showing that application of AITC (30 µM; red box) or 4-HNE (1 µM; green box) onto capillary extremities did not dilate the upstream arteriole in microvascular preparations from Panx1-ecKO mice, whereas vasodilatory effects of elevated KCl (10 mM; blue box) were unchanged. (B and C) Summary data showing that neither 4-HNE (1 µM) (B) nor AITC (30 µM) (C) evoked dilation of upstream arterioles in preparations from Panx1-ecKO mice (n = 6 preparations from five animals; *p<0.05 vs. Cdh5-Cre injected with tamoxifen, #p<0.05 vs. Panx1-ecKO mice injected with vehicle (peanut oil), one-way ANOVA). (D) Summary data showing that the response to elevated KCl (10 nM) was unchanged in preparations from Panx1-ecKO mice (n = 6 preparations from five animals; one-way ANOVA). (E) Summary data showing that catabolism of extracellular purines with apyrase (1 U/mL) blunted the response to AITC (30 µM) in microvascular preparations from wild-type mice (n = 9 preparations from eight animals; *p<0.05, paired t-test). (F) Summary data showing that the pan-P2X inhibitor PPADS (10 µM) inhibited the response to AITC (30 µM) (n = 9 preparations from six animals; *p<0.05, paired t-test). (G and H). The response to elevated KCl (10 mM) was unaffected by treatment with apyrase (1 U/mL) (G) or PPADS (10 µM) (H) (n = 9 preparations from six to eight animals; paired t-test). (I) Representative trace showing that application of ATP (10 µM; orange box) onto capillary extremities dilated the upstream arteriole in microvascular preparations from wild-type mice, and that this response was blocked by the pan-P2X inhibitor PPADS (10 µM). (J) Summary data showing that PPADS (10 µM) inhibited the response ATP (10 µM) responses (n = 6 preparations from four animals; *p<0.05, paired t-test). (K) The velocity of the ATP (10 µM) response was significantly slower compared with that to activation of Kir channels with KCl (60 mM) (n = 6 preparations from four animals; *p<0.05, paired t-test). (L) Summary data showing that ATP (10 µM) did not evoke dilation of upstream arterioles in preparations from Panx1-ecKO mice (n = 6 preparations from three to four animals; *p<0.05 vs. Cdh5-Cre injected with tamoxifen, #p<0.05 vs. Panx1-ecKO mice injected with vehicle (peanut oil), one-way ANOVA).
-
Figure 4—source data 1
Individual data points and analysis summaries for datasets shown in Figure 4.
- https://cdn.elifesciences.org/articles/63040/elife-63040-fig4-data1-v2.xlsx
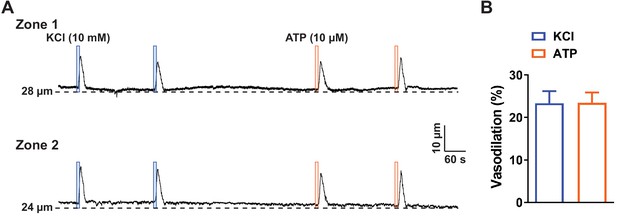
ATP-induced dilation persisted in preparations from Trpa1-ecKO mice.
(A and B) Representative traces (A) and summary data (B) showing that application of elevated KCl (10 mM; blue box) and ATP (10 µM; orange box) directly onto capillary extremities induced a dilation of the upstream in preparations from Trpa1-ecKO mice (n = 6 preparations from four animals; paired t-test).
-
Figure 4—figure supplement 1—source data 1
Individual data points and analysis summaries for datasets shown in Figure 4—figure supplement 1.
- https://cdn.elifesciences.org/articles/63040/elife-63040-fig4-figsupp1-data1-v2.xlsx
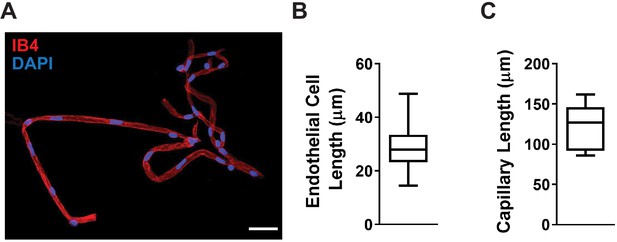
Average length of a cerebral capillary endothelial cell.
(A) Representative image of an isolated cerebral capillary network. Endothelial cells and nuclei were stained with Alexa Fluor 568 isolectin B4 (IB4) (red) and DAPI (blue), respectively. Scale bar = 25 µm. (B) Summary data showing the average length of capillary endothelial cells, calculated by measuring the inter-nuclear distance (determined from 173 endothelial cells from four animals). (C) Summary data showing the average length of the capillary segment in isolated ex vivo microvascular preparations (n = 9 preparations from six animals). Data are shown as a box and whisker plot.
-
Figure 4—figure supplement 2—source data 1
Individual data points and analysis summaries for datasets shown in Figure 4—figure supplement 2.
- https://cdn.elifesciences.org/articles/63040/elife-63040-fig4-figsupp2-data1-v2.xlsx
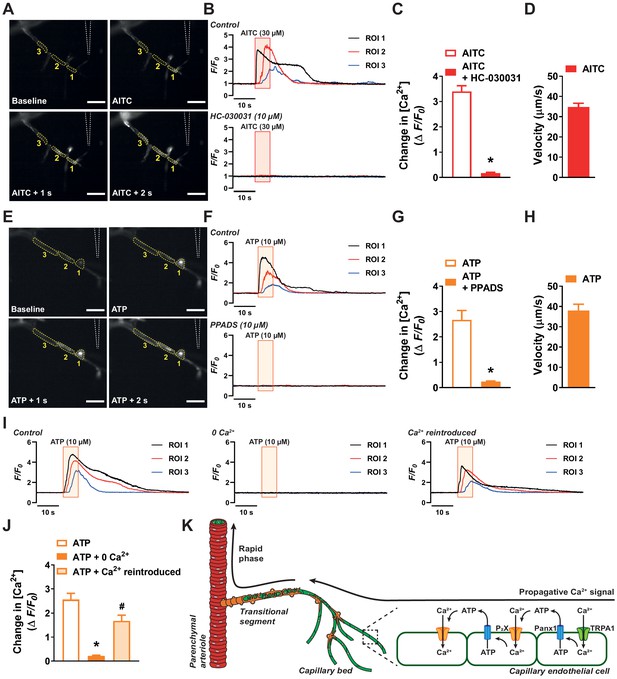
Activation of capillary TRPA1 channels and purinergic receptors produces a Ca2+ response that travels through the capillary bed.
(A) Representative time course images demonstrating the fractional increase in fluorescence (F/F0) of the Ca2+ signal in a capillary segment in microvascular preparations from transgenic mice expressing a genetically encoded Ca2+ indicator, GCaMP8, exclusively in the endothelium (Cdh5-GCaMP8). The tip of the micropipette (outlined) was placed adjacent to distal extremities of capillaries. Focal application of AITC (30 µM) evoked a propagative Ca2+ signal that was observed in adjacent region of interests. Scale bar = 50 µm. (B) Representative traces showing the fractional increase in florescence following application of AITC (30 µM; red box) that was blocked by the selective TRPA1 antagonist HC-030031 (10 µM). (C) Summary data showing that HC-030031 (10 µM) inhibited the response to AITC (30 µM) (n = 6 preparations from five animals; *p<0.05, paired t-test). (D) The velocity of the response through capillary segments evoked by activation of TRPA1 channels (n = 6 preparations from five animals). (E) Representative time course images demonstrating the fractional increase in fluorescence of the Ca2+ signal produced following focal application of ATP (10 µM) to distal capillaries. Propagation of the Ca2+ signal was observed in the adjacent region of interests following ATP (10 µM) application. Scale bar = 50 µm. (F) Representative traces showing the fractional increase in florescence following application of ATP (10 µM; orange box) that was blocked by the pan-P2X inhibitor PPADS (10 µM). (G) Summary data showing that PPADS (10 µM) inhibited the response to ATP (10 µM) (n = 7 preparations from seven animals; *p<0.05, paired t-test). (H) The velocity of the response through capillary segments evoked by activation of purinergic receptors (n = 13 preparations from 12 animals). (I) Representative traces showing the fractional increase in florescence following application of ATP (10 µM; orange box) was abolished when the preparation was superfused in Ca2+-free aCSF. The response was restored following the reintroduction of extracellular Ca2+. (J) Summary data showing that bathing the preparation in Ca2+-free aCSF solution attenuated the response to ATP (10 µM), but was restored once Ca2+ (2 mM) was reintroduced (n = 6 preparations from five animals; *p<0.05 vs. control ATP response, #p<0.05 vs. preparations bathed in 0 Ca2+, one-way ANOVA). (K) Illustration of the proposed model for signal propagation through the capillary bed. Increases in intracellular [Ca2+] caused by TRPA1 channel-mediated Ca2+ influx induce ATP release through Panx1 channels, which in turn activates purinergic P2X receptors on the adjacent endothelial cell.
-
Figure 5—source data 1
Individual data points and analysis summaries for datasets shown in Figure 5.
- https://cdn.elifesciences.org/articles/63040/elife-63040-fig5-data1-v2.xlsx
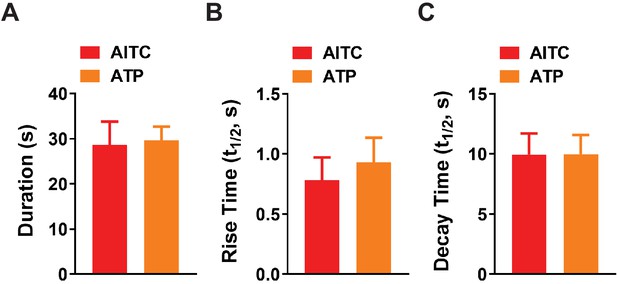
Kinetics of the Ca2+ response in capillaries following activation of TRPA1 channels purinergic receptors.
(A to C) Duration (A), rise time [half-time (t1/2, s)] (B) and decay time (t1/2, s) (C) of the Ca2+ response following focal application of AITC (30 µM) and ATP (10 µM) to distal capillaries in microvascular preparations from Cdh5-GCaMP8 (AITC, n = 6 preparations from five animals; ATP, n = 13 preparations from 12 animals; unpaired t-test).
-
Figure 5—figure supplement 1—source data 1
Individual data points and analysis summaries for datasets shown in Figure 5—figure supplement 1.
- https://cdn.elifesciences.org/articles/63040/elife-63040-fig5-figsupp1-data1-v2.xlsx
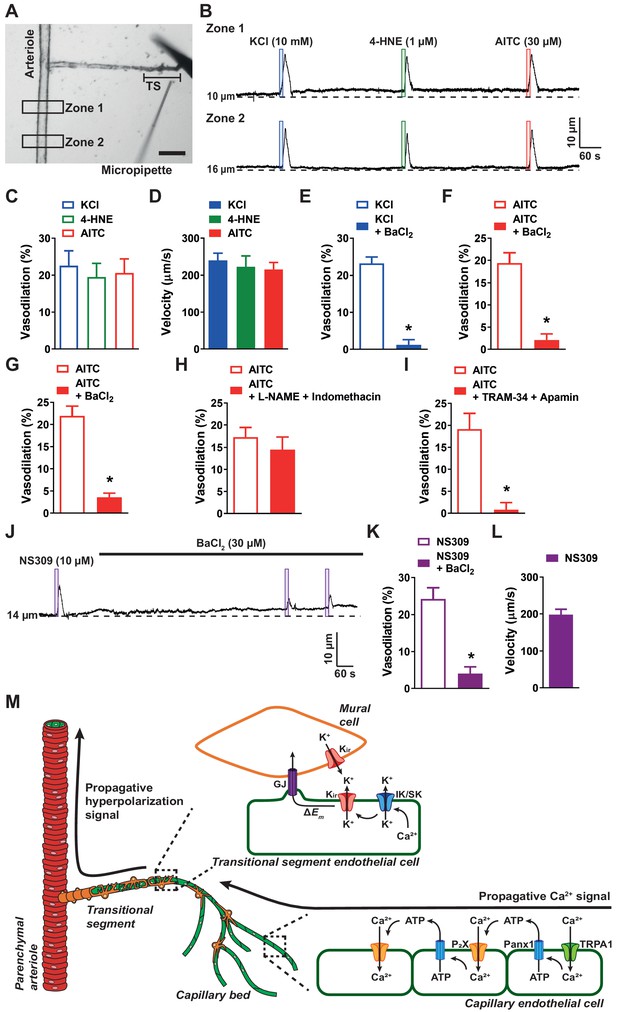
Rapid propagation of TRPA1-induced vasodilation is dependent on Kir, IK, and SK channels.
(A) Representative image of a cerebral microvascular preparation in which the capillary bed was removed while leaving the post-arteriole transitional segment (TS) intact. The transitional segment is located at the distal end of the offshoot arteriole branch. Scale bar = 100 µm. A picospritzing cannula was used to directly apply drugs to the post-arteriole transitional segment. (B) Representative traces showing that application of elevated KCl (10 mM; blue box), AITC (30 µM; red box), or 4-HNE (1 µM; green box) onto the post-arteriole transitional segment increased the lumen diameter of the upstream arteriole in microvascular preparations from wild-type mice. (C) Summary data showing dilation produced by elevated KCl (10 mM), AITC (30 µM) and 4-HNE (1 µM) (n = 6–8 preparations from four to five animals; one-way ANOVA). (D) Velocity was calculated from the post-arteriole transitional segment to a point in the upstream arteriole following localized drug application onto the post-arteriole transitional segment. Velocity measurements were comparable for elevated KCl (10 mM), 4-HNE (1 µM), and AITC (30 µM) (n = 6–8 preparations from four to five animals; one-way ANOVA). (E and F) Summary data showing that BaCl2 (30 µM) significantly reduced the response to elevated KCl (10 mM) (E) and AITC (30 µM) (F) when applied to the post-arteriole transitional segment (n = 6 preparations from four animals; *p<0.05, paired t-test). (G) Summary data showing that BaCl2 (30 µM) significantly attenuated the response to AITC (30 µM) when directly applied onto capillary extremities (n = 6 preparations from four animals; *p<0.05, paired t-test). (H) Summary data showing that combined inhibition of nitric oxide synthase and cyclooxygenase with L-NAME (100 µM) and indomethacin (10 µM), respectively, did not affect the response to AITC (30 µM) when directly onto capillary extremities (n = 7 preparations from four animals; paired t-test). (I) Summary data showing that combined IK and SK channel inhibition with TRAM-34 (1 µM) and apamin (300 nM), respectively, significantly attenuated the response to AITC (30 µM) when directly applied onto capillary extremities (n = 7 preparations from five animals; *p<0.05, paired t-test). (J and K) Representative trace (J) and summary data (K) showing that application of the IK and SK channel activator NS309 (10 µM; purple box) directly onto the post-arteriole transitional segment dilated the upstream arteriole, and that this response was attenuated by inhibition of Kir channels with BaCl2 (30 µM) (n = 6 preparations from four animals; *p<0.05, paired t-test). (L) The velocity of the response produced by application of the IK and SK channel activator NS309 (10 µM) onto the post-arteriole transitional segment was similar to that produced by activation of Kir channels or TRPA1 channels (n = 6 preparations from four animals). (M) Illustration of the proposed signaling model depicting events following activation of capillary endothelial TRPA1 channels. Once the Ca2+ signal from the capillary bed arrives at the post-arteriole transitional segment, IK and SK channels are activated and facilitate K+ efflux. This in turn activates Kir channels, which propagate the signal retrogradely to cause dilation of the upstream arteriole.
-
Figure 6—source data 1
Individual data points and analysis summaries for datasets shown in Figure 6.
- https://cdn.elifesciences.org/articles/63040/elife-63040-fig6-data1-v2.xlsx
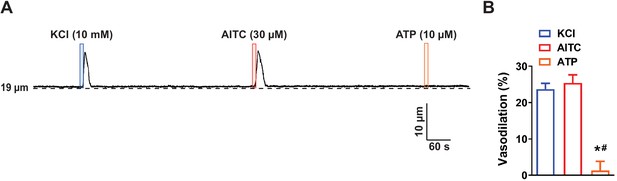
ATP does not evoke dilation of upstream arterioles when applied to the post-arteriole transitional segment.
(A) Representative trace showing that application of elevated KCl (10 mM; blue box) or AITC (30 µM; red box) onto the post-arteriole transitional segment increased the lumen diameter of the upstream arteriole, but application of ATP (10 µM; orange box) to this segment did not. (B) Summary data showing that application of ATP (10 µM) onto the post-arteriole transitional segment did not dilate upstream arterioles (n = 6 preparations from three animals; *p<0.05 vs. KCl, #p<0.05 vs. AITC, one-way ANOVA).
-
Figure 6—figure supplement 1—source data 1
Individual data points and analysis summaries for datasets shown in Figure 6—figure supplement 1.
- https://cdn.elifesciences.org/articles/63040/elife-63040-fig6-figsupp1-data1-v2.xlsx
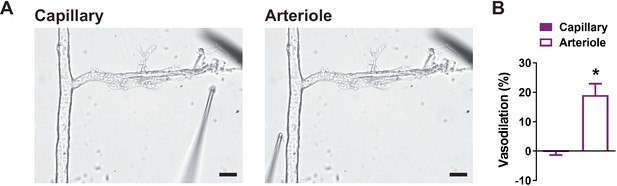
Application of NS309 to capillary extremities has no effect on the upstream arteriole.
(A) Representative images of an intact microvascular preparation showing the drug-administering cannula positioned adjacent to capillary extremities (left) and arteriole segment (right). Scale bar = 50 µm. (B) Summary data showing that application of the IK and SK channel activator NS309 (10 µM) did not induce relaxation of the upstream arteriole when applied to capillaries, but did cause dilation when applied directly to the arteriole (n = 6 preparations from three animals; *p<0.05, paired t-test).
-
Figure 6—figure supplement 2—source data 1
Individual data points and analysis summaries for datasets shown in Figure 6—figure supplement 2.
- https://cdn.elifesciences.org/articles/63040/elife-63040-fig6-figsupp2-data1-v2.xlsx
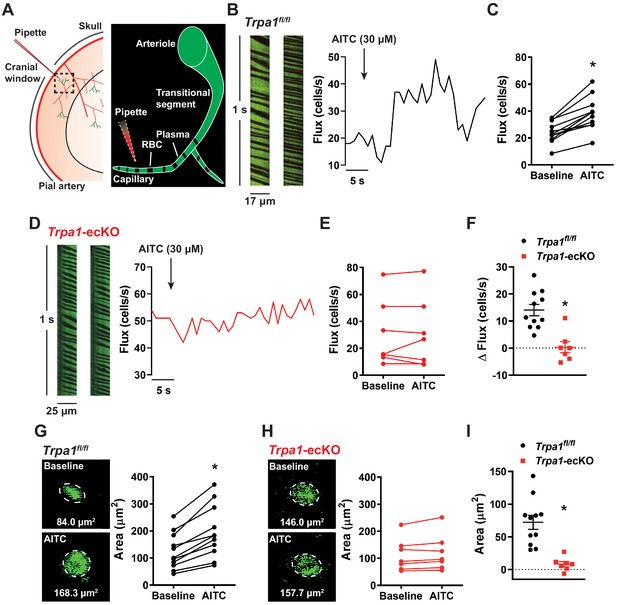
In vivo stimulation of capillary TRPA1 channels increases RBC flux within the capillary bed.
(A) Experimental illustration. Mice were injected with FITC-conjugated dextran to allow visualization of the cortical vasculature through a cranial window using in vivo two-photon laser-scanning microscopy. RBCs appear as black shadows against green fluorescent plasma. A pipette containing AITC and TRITC-dextran (for pipette visualization) was positioned next to a capillary. (B) RBC flux through a single capillary was examined by analyzing distance-time plots of capillary line scans. TRPA1 channels on capillary endothelial cells were locally stimulated by picospritzing AITC (30 µM) directly onto a single capillary. Representative line-scan plots of a capillary (left) and representative time-flux trace (right) demonstrating changes in RBC flux before and after application of AITC (30 µM) in control Trpa1fl/fl mice. (C) Summary data showing that AITC (30 µM) increased RBC flux in the stimulated capillary of Trpa1fl/fl mice (n = 11 animals; *p<0.05, paired t-test). (D) Representative line-scan plots of a capillary (left) and representative time-flux trace (right) demonstrating that application of AITC (30 µM) had no effect in Trpa1-ecKO mice. (E) Summary data showing that AITC (30 µM) had no effect in Trpa1-ecKO mice (n = 7 animals, paired t-test). (F) Change in RBC flux in control Trpa1fl/fl versus Trpa1-ecKO mice (n = 7–11 animals; *p<0.05, unpaired t-test). (G and H) Representative images and summary data showing change in cross-sectional area of the upstream arteriole following application of AITC (30 µM) onto a capillary of control Trpa1fl/fl (G) and Trpa1-ecKO (H) mice (n = 7–11 animals; *p<0.05, paired t-test). (I) Change in arteriole cross-sectional area was less in Trpa1-ecKO mice compared to control Trpa1fl/fl (n = 7–11 animals; *p<0.05, unpaired t-test).
-
Figure 7—source data 1
Individual data points and analysis summaries for datasets shown in Figure 7.
- https://cdn.elifesciences.org/articles/63040/elife-63040-fig7-data1-v2.xlsx
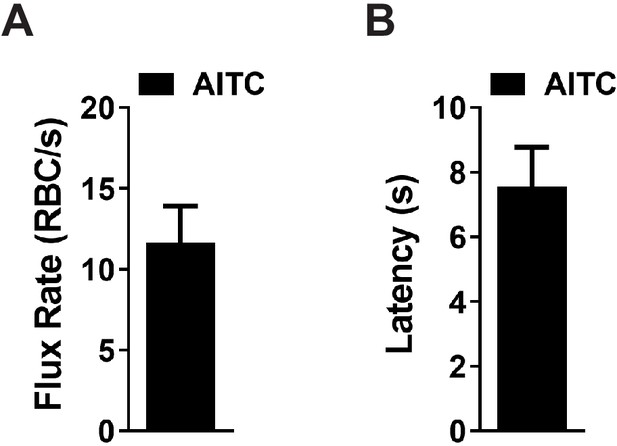
Kinetics of the RBC flux increase following in vivo stimulation of capillary TRPA1 channels.
(A and B) Summary data showing the rate of RBC flux increase (A) and latency of the response following local application of AITC (30 µM) directly onto a single capillary of Trpa1fl/fl mice (n = 11 animals).
-
Figure 7—figure supplement 1—source data 1
Individual data points and analysis summaries for datasets shown in Figure 7—figure supplement 1.
- https://cdn.elifesciences.org/articles/63040/elife-63040-fig7-figsupp1-data1-v2.xlsx
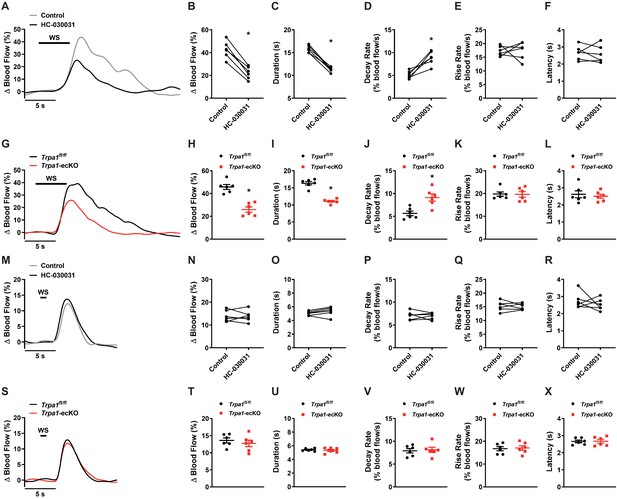
Functional hyperemia is dependent on brain capillary TRPA1 channels.
(A and B) Representative traces (A) and summary data (B) showing the hyperemic response in the somatosensory cortex following contralateral whisker stimulation (WS) for 5 s, measured using laser-Doppler flowmetry in wild-type mice. Treatment with HC-030031 (100 mg/kg, i.p. for 30 min) reduced the hyperemic response (n = 6 animals; *p<0.05, paired t-test). (C–F) Duration (C), decay rate (D), rise rate (E), and latency (F) of the hyperemic response was also determined, and a reduction in duration and increase in decay rate was observed in animals treated with HC-030031 (100 mg/kg, i.p. for 30 min) (n = 6 animals; *p<0.05, paired t-test). (G and H) Representative traces (G) and summary data (H) showing the hyperemic response is blunted in Trpa1-ecKO mice compared with Trpa1fl/fl controls following contralateral whisker stimulation for 5 s (n = 6 animals; *p<0.05, unpaired t-test). (I to L) Duration (I), decay rate (J), rise rate (K), and latency (L) of the hyperemic response was also determined, and duration was diminished and decay rate was increased in Trpa1-ecKO compared with Trpa1fl/fl mice (n = 6 animals; *p<0.05, unpaired t-test). (M and N) Representative traces (M) and summary data (N) showing the hyperemic response was unchanged following a 1 s whisker stimulation in animals treated with HC-030031 (100 mg/kg, i.p. for 30 min) (n = 6 animals; paired t-test). (O–R) Duration (O), decay rate (P), rise rate (Q), and latency (R) were unaffected by HC-030031 treatment (100 mg/kg, i.p. for 30 min) (n = 6 animals; paired t-test). (S and T) Representative traces (S) and summary data (T) showing the hyperemic response did not differ between Trpa1fl/fl and Trpa1-ecKO mice following a 1 s whisker stimulation (n = 6 animals; unpaired t-test). (U–X) Duration (U), decay rate (V), rise rate (W), and latency (X) also did not differ between Trpa1fl/fl and Trpa1-ecKO mice (n = 6 animals; unpaired t-test).
-
Figure 8—source data 1
Individual data points and analysis summaries for datasets shown in Figure 8.
- https://cdn.elifesciences.org/articles/63040/elife-63040-fig8-data1-v2.xlsx
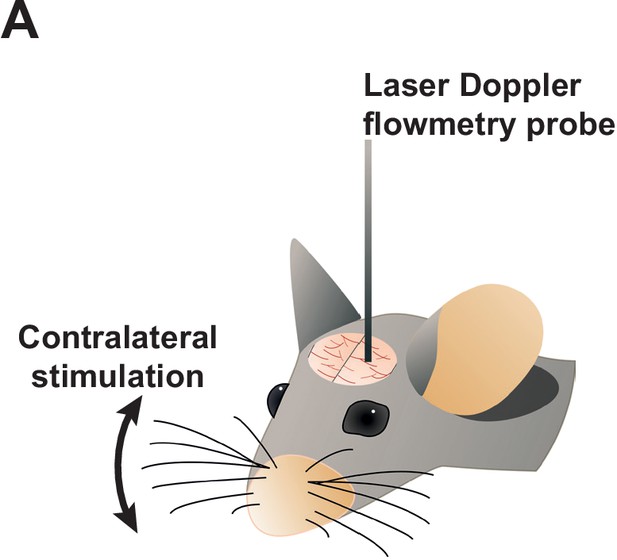
Experimental illustration of how functional hyperemia was measured in vivo.
(A) Functional hyperemia was assessed in the somatosensory cortex through a thinned skull. Relative changes in blood flow in response to contralateral whisker stimulation were recorded using laser-Doppler flowmetry.
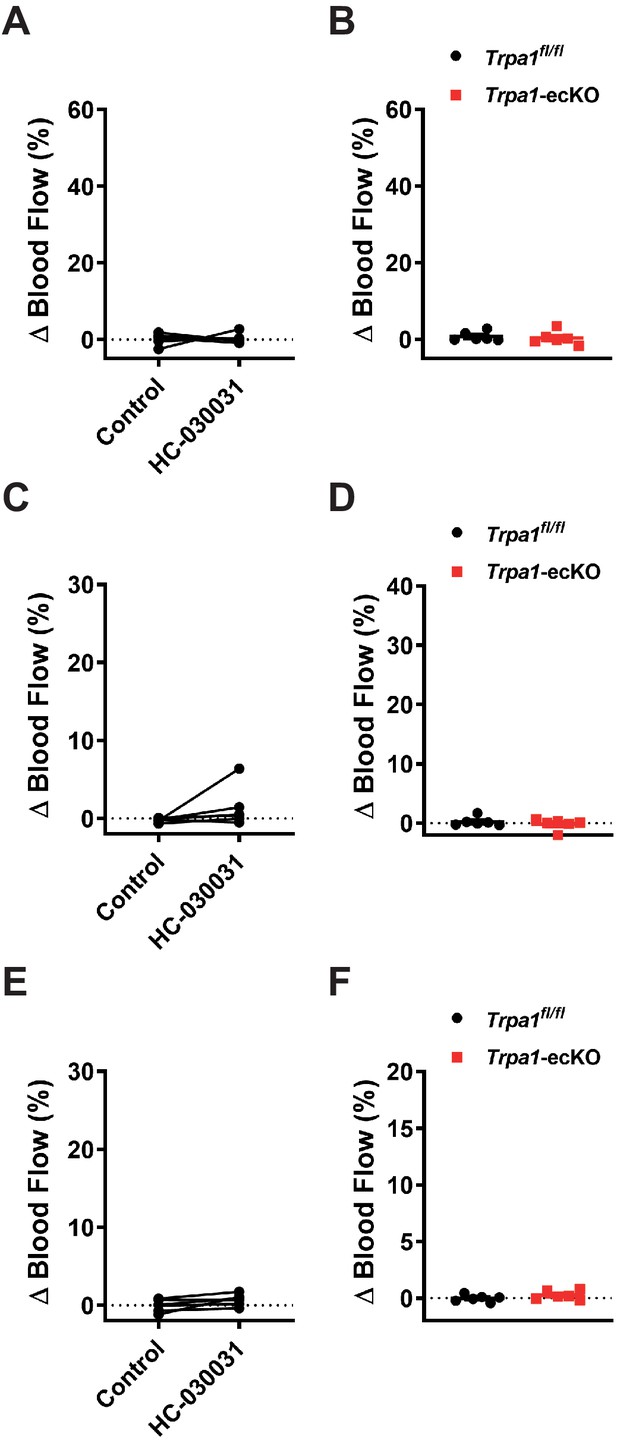
Lack of a hyperemic response following ipsilateral whisker stimulation.
(A and B) Summary data showing the lack of a hyperemic response in the somatosensory cortex following 5 s ipsilateral whisker stimulation, measured using laser-Doppler flowmetry in mice prior to and following treatment with HC-030031 (100 mg/kg, i.p. for 30 min) (A) (n = 6 animals; paired t-test) and Trpa1-ecKO mice and control Trpa1fl/fl mice (B) (n = 6 animals; unpaired t-test). (C and D) Summary data showing the lack of a hyperemic response following 2 s ipsilateral whisker stimulation in mice prior to and following treatment with HC-030031 (100 mg/kg, i.p. for 30 min) (C) (n = 6 animals; paired t-test) and Trpa1-ecKO mice and control Trpa1fl/fl mice (D) (n = 6 animals; unpaired t-test). (E and F) Summary data showing the lack of a hyperemic response following 1 s ipsilateral whisker stimulation in mice prior to and following treatment with HC-030031 (100 mg/kg, i.p. for 30 min) (E) (n = 6 animals; paired t-test) and Trpa1-ecKO mice and control Trpa1fl/fl mice (F) (n = 6 animals; unpaired t-test).
-
Figure 8—figure supplement 2—source data 1
Individual data points and analysis summaries for datasets shown in Figure 8—figure supplement 2.
- https://cdn.elifesciences.org/articles/63040/elife-63040-fig8-figsupp2-data1-v2.xlsx
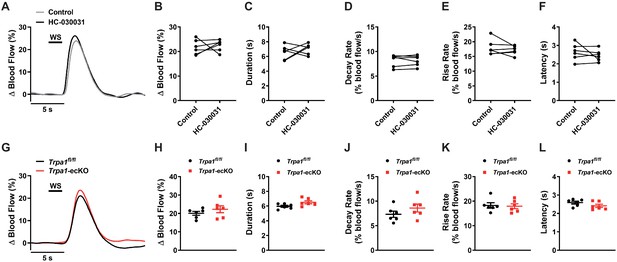
Functional hyperemic response following 2 s whisker stimulation.
(A and B) Representative traces (A) and summary data (B) showing the hyperemic response was unchanged following a 2 s whisker stimulation in animals treated with HC-030031 (100 mg/kg, i.p. for 30 min) (n = 6 animals; paired t-test). (C to F) Duration (C), decay rate (D), rise rate (E), and latency (F) were unaffected by HC-030031 treatment (100 mg/kg, i.p. for 30 min) (n = 6 animals; paired t-test). (G and H) Representative traces (G) and summary data (H) showing the hyperemic response did not differ between control Trpa1fl/fl and Trpa1-ecKO mice following a 2 s whisker stimulation (n = 6 animals per group; unpaired t-test). (I to L) Duration (I), decay rate (J), rise rate (K), and latency (L) did not differ between control Trpa1fl/fl and Trpa1-ecKO mice (n = 6 animals per group; unpaired t-test).
-
Figure 8—figure supplement 3—source data 1
Individual data points and analysis summaries for datasets shown in Figure 8—figure supplement 3.
- https://cdn.elifesciences.org/articles/63040/elife-63040-fig8-figsupp3-data1-v2.xlsx
Videos
Localized application of Evans Blue dye onto capillary extremities of a microvascular preparation.
Representative time-series images of a microvascular preparation demonstrating that application of Evans Blue dye (1% w/v) is localized to the region of the capillary tree and does not spread to the upstream parenchymal arteriole. Evans Blue was applied after 10 s. Scale bar = 100 µm.
Localized application of AITC onto capillary extremities of a microvascular preparation dilates the upstream arteriole.
Representative time-series images of a microvascular preparation demonstrating that localized application of AITC (30 µM) onto capillary extremities dilates the upstream arteriole. AITC was applied after 10 s. Scale bar = 50 µm.
Focal application of AITC onto capillary extremities induces an increase in intracellular [Ca2+].
Representative time-series images of a microvascular preparation demonstrating that localized application of AITC (30 µM) onto distal capillary extremities produces a propagative Ca2+ signal. AITC was applied after 10 s. Scale bar = 50 µm.
Increase in intracellular [Ca2+] initiated by AITC is blocked by the selective TRPA1 antagonist HC-030031.
Representative time-series images of a microvascular preparation demonstrating that the propagative Ca2+ signal produced by AITC (30 µM) is blocked by superfusing the preparation with HC-030031 (10 µM). AITC was applied after 10 s. Scale bar = 50 µm.
Focal application of ATP onto capillary extremities induces an increase in intracellular [Ca2+].
Representative time-series images of a microvascular preparation demonstrating that localized application of ATP (10 µM) onto distal capillary extremities produces a propagative Ca2+ signal. ATP was applied after 10 s. Scale bar = 50 µm.
Increase in intracellular [Ca2+] initiated by AITC is blocked by the pan-P2X inhibitor PPADS.
Representative time-series images of a microvascular preparation demonstrating that the propagative Ca2+ signal produced by ATP (10 µM) is blocked by superfusing the preparation with PPADS (10 µM). ATP was applied after 10 s. Scale bar = 50 µm.
ATP-induced Ca2+ signal under normal conditions.
Representative time-series images of a microvascular preparation demonstrating that localized application of ATP (10 µM) onto distal capillary extremities produces a propagative Ca2+ signal in preparations superfused with Ca2+-containing aCSF. ATP was applied after 10 s. Scale bar = 50 µm.
ATP-induced Ca2+ signal is abolished in extracellular Ca2+-free conditions.
Representative time-series images of a microvascular preparation demonstrating that localized application of ATP (10 µM) onto distal capillary extremities failed to induced a propagative Ca2+ signal in preparations superfused with Ca2+-free aCSF. ATP was applied after 10 s. Scale bar = 50 µm.
ATP-induced Ca2+ signal is restored following reintroduction of extracellular Ca2+.
Representative time-series images of a microvascular preparation demonstrating that the propagative Ca2+ signal following localized application of ATP (10 µM) onto distal capillary extremities returned once extracellular Ca2+ (2 mM) was reintroduced to the preparation. ATP was applied after 10 s. Scale bar = 50 µm.
Localized application of Evans Blue dye onto the post-arteriole transitional segment of a microvascular preparation.
Representative time-series images of a modified microvascular preparation in which the capillary tree was removed. Application of Evans Blue dye (1% w/v) onto the post-arteriole transitional segment was localized to this region and did not spread to the upstream parenchymal arteriole. Evans Blue was applied after 10 s. Scale bar = 100 µm.
Tables
Reagent type (species) or resource | Designation | Source or reference | Identifiers | Additional information |
---|---|---|---|---|
Genetic reagent (M. musculus) | C57BL/6J | Jackson Laboratory | Stock #: 000664 RRID:IMSR_JAX:000664 | |
Genetic reagent (M. musculus) | Panx1-ecKO | Dr. Brant Isakson PMID:26242575 | ||
Genetic reagent (M. musculus) | TekCre | Jackson Laboratory | Stock #: 008863 RRID:IMSR_JAX:008863 | |
Genetic reagent (M. musculus) | Trpa1fl/fl | Jackson Laboratory | Stock #: 008654 RRID:IMSR_JAX:008654 | |
Genetic reagent (M. musculus) | Cdh5-GCaMP8 | CHROMus(https://chromus.vet.cornell.edu/cdh5gcamp8/) PMID:23240011 | ||
Antibody | Cy3 conjugated anti-α-smooth muscle actin (Mouse monoclonal) | Sigma-Aldrich, Inc | Cat. #: C6198 RRID:AB_476856 | (1:200, 1.0–1.5 mg/ml) |
Other | Alexa Fluor 488 conjugated isolectin B4 | ThermoFisher Scientific | Cat. #: I21411 | (1:200, 1.0 mg/ml) |
Other | Alexa Fluor 568 conjugated isolectin B4 | ThermoFisher Scientific | Cat. #: I21412 | (1:200, 1.0 mg/ml) |
Other | Alexa Fluor 633 conjugated hydrazide | ThermoFisher Scientific | Cat. #: A30634 | (1:1000, 1.0 mg/ml) |
Other | DAPI Fluoroshied mounting medium | Abcam plc. | Cat. #: ab104139 | |
Other | Fluorescein isothiocyanate (FITC)-dextran | Sigma-Aldrich, Inc | Cat. #: FD150S | |
Other | Tetramethylrhodamine isothiocyanate (TRITC)-dextran | Sigma-Aldrich, Inc | Cat. #: T1287 | |
Peptide, recombinant protein | Apamin | Tocris Bioscience | Cat. #: 1652 | |
Peptide, recombinant protein | Apyrase | Sigma-Aldrich, Inc | Cat. #: A6535 | |
Peptide, recombinant protein | Collagenase type I | Worthington Biochemical Corporation | Cat. #: LS004194 | |
Peptide, recombinant protein | Elastase | Worthington Biochemical Corporation | Cat. #: LS002292 | |
Peptide, recombinant protein | Neutral protease | Worthington Biochemical Corporation | Cat. #: LS02104 | |
Chemical compound, drug | 4-hydroxynonenal (4-HNE) | Cayman Chemical | Cat. #: 32100 | |
Chemical compound, drug | Adenosine 5-triphosphate (ATP) disodium salt | Sigma-Aldrich, Inc | Cat. #: A2383 | |
Chemical compound, drug | Allyl isothiocyanate (AITC) | Sigma-Aldrich, Inc | Cat. #: 377430 | |
Chemical compound, drug | HC-030031 | Tocris Bioscience | Cat. #: 2896 | |
Chemical compound, drug | Indomethacin | Sigma-Aldrich, Inc | Cat. #: I7378 | |
Chemical compound, drug | NS309 | Tocris Bioscience | Cat. #: 3895 | |
Chemical compound, drug | Nω-Nitro-L-arginine methyl ester (L-NAME) hydrochloride | Sigma-Aldrich, Inc | Cat. #: N5751 | |
Chemical compound, drug | PPADS tetrasodium salt | Tocris Bioscience | Cat. #: 0625 | |
Chemical compound, drug | Tamoxifen | Sigma-Aldrich, Inc | Cat. #: T5648 | |
Software, algorithm | pClamp software | Molecular Devices, LLC. (http://www.moleculardevices.com/products/software/pclamp.html) | RRID:SCR_011323 | Version 10.2 |
Software, algorithm | FluoView FV1000 FV10-ASW software | Olympus (https://www.olympus-lifescience.com/en/support/downloads/) | RRID:SCR_014215 | Version 4.02 |
Software, algorithm | GraphPad Prism software | GraphPad Software, Inc (https://www.graphpad.com/) | RRID:SCR_002798 | Version 8.2 |
Software, algorithm | ImageJ software | National Institutes of Health (https://imagej.nih.gov/ij/) | RRID:SCR_003070 | Version 1.52 n |
Software, algorithm | IonWizard software | IonOptix, LLC. (https://www.ionoptix.com/products/software/ionwizard-core-and-analysis/) | Version 6.4.1.73 | |
Software, algorithm | VisiView software | Visitron Systems GmbH (https://www.visitron.de/products/visiviewr-software.html) | Version 4.5.0.7 | |
Software, algorithm | μManager software | University of California, San Francisco (https://micro-manager.org/) | RRID:SCR_000415 | Version 1.4.22 |