Post-translational flavinylation is associated with diverse extracytosolic redox functionalities throughout bacterial life
Figures
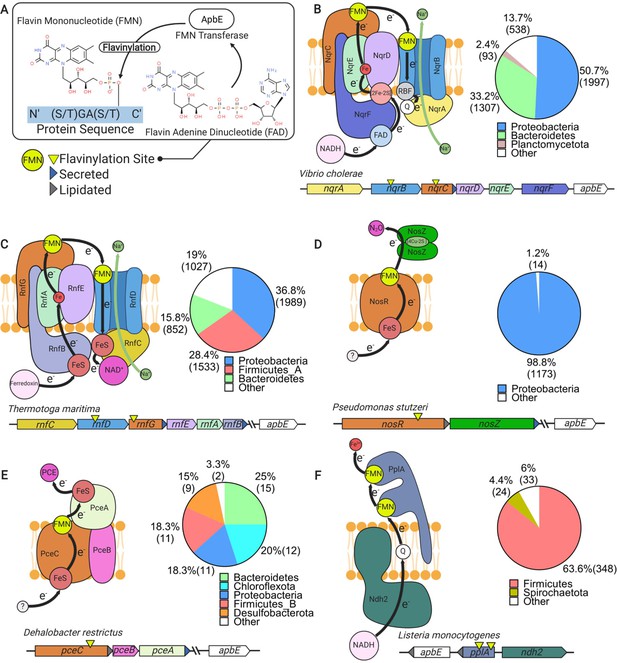
Characterized extracytosolic electron transfer systems with an ApbE-flavinylated subunit.
(A) The post-translational flavinylation reaction catalyzed by the ApbE enzyme. (B–F) Models of previously characterized electron transfer systems that include an ApbE-flavinylated subunit. Gene clusters that encode each system are shown, with arrowheads indicating gene orientation and additional features shown in the key. ‘Secreted’ and ‘lipidated’ refer to the presence of computationally predicted signal peptides and lipidation sites, respectively. Black arrows trace proposed electron transfer pathways. Depicted systems and primary references for the model are: (B) NADH:quinone oxidoreductase (NQR) (Steuber et al., 2014), (C) Rhodobacter nitrogen fixation (RNF) (Kuhns et al., 2020), (D) nitrous oxide reduction (Zhang et al., 2017), (E) organohalide reduction (Buttet et al., 2018), and (F) extracellular electron transfer (Light et al., 2018). Accompanying pie charts show the number of genomes within each phylum that encode the electron transfer system.
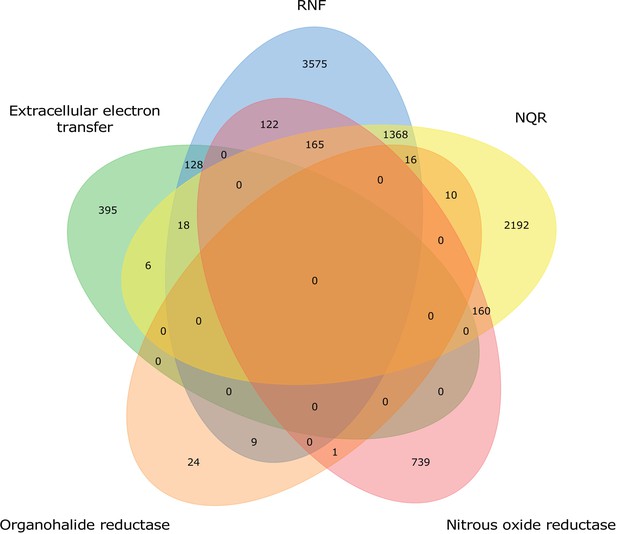
Distribution of characterized extracytosolic electron transfer systems within prokaryotic genomes.
Venn diagram showing the number of characterized systems within the 8928 genomes that have at least one of the previously characterized extracytosolic electron transfer systems.
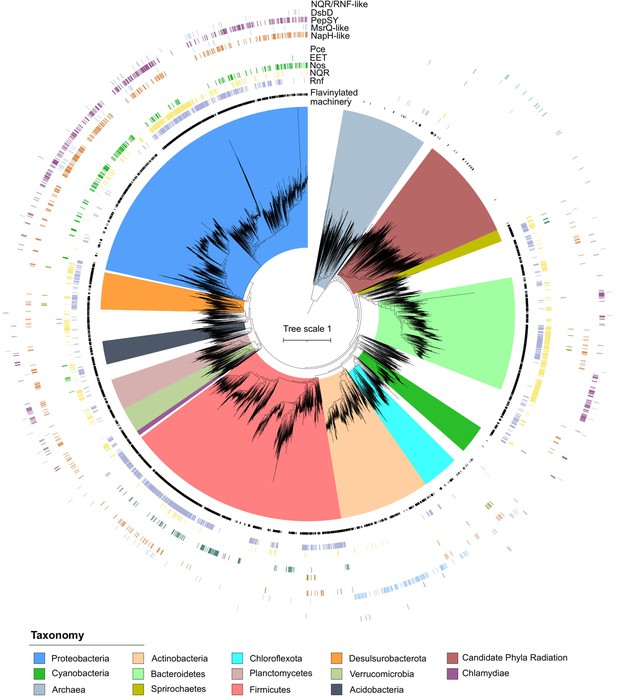
Phylogenetic distribution of extracytosolic protein flavinylation in prokaryotes.
Phylogenetic reconstruction of the evolutionary history of 9152 genomes representing 97% of the diversity available at the genus level in the GTDB (9428 distinct genera) (Parks et al., 2020). The maximum likelihood tree was constructed based on a concatenated alignment of 14 ribosomal proteins under an LG + I + G4 model of evolution (2850 amino acid sites). The inner to outer rings display the presence in one genome of the genus of extracytosolic flavinylated proteins (i.e., genomes encoding a signal peptide-containing flavin mononucleotide [FMN]-binding or ApbE) (flavinylation machinery), the presence of a Rhodobacter nitrogen fixation (RNF) system, an NADH:quinone oxidoreductase (NQR) system, a Nos system, an EET system, and an organohalide reduction system (Pce), the presence of an NapH-like system, an MsrQ-like system, a PepSY system, a DsbD system, and an NQR/RNF-like system. The scale bar indicates the mean number of substitutions per site.
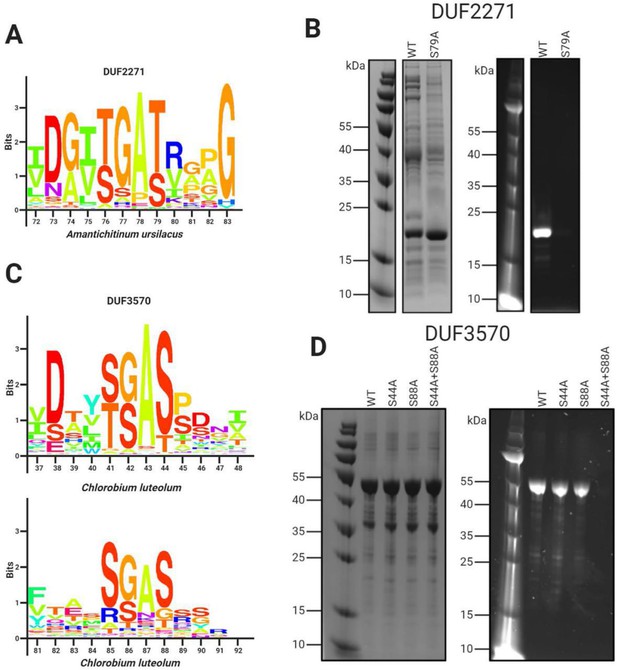
Domains of unknown function DUF2271 and DUF3570 are flavinylated by ApbE.
(A) Conserved sequence motif within 282 flavinylation-associated DUF2271 proteins. Letter size (bits) is proportional to amino acid frequency at each position in the sequence alignment. Amino acid numbering corresponds to the Amantichitinum ursilacus DUF2271 sequence. (B) SDS-PAGE of purified A. ursilacus DUF2271 variants coexpressed in Escherichia coli with their cognate ApbE. The gel is shown with coomassie stain (left) and under ultraviolet illumination (right). (C) Conserved sequence motif within 228 flavinylation-associated DUF3570 proteins. Letter size (bits) is proportional to amino acid frequency at each position in the sequence alignment. Amino acid numbering corresponds to the Chlorobium luteolum DUF3570 sequence. (D) SDS-PAGE of purified C. luteolum DUF3570 variants coexpressed in E. coli with their cognate ApbE. The gel is shown with coomassie stain (left) and under ultraviolet illumination (right).
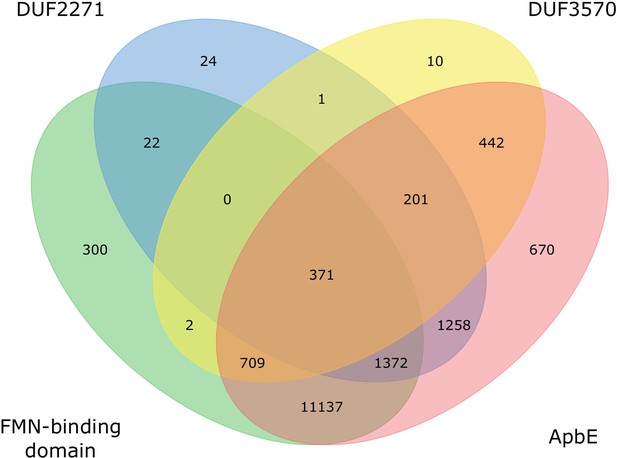
Presence of apbE and its putative substrates in prokaryotic genomes.
Venn diagram showing numbers of shared protein-coding genes between the genomes. Only genomes with a signal peptide predicted in at least one of the four genes are included.
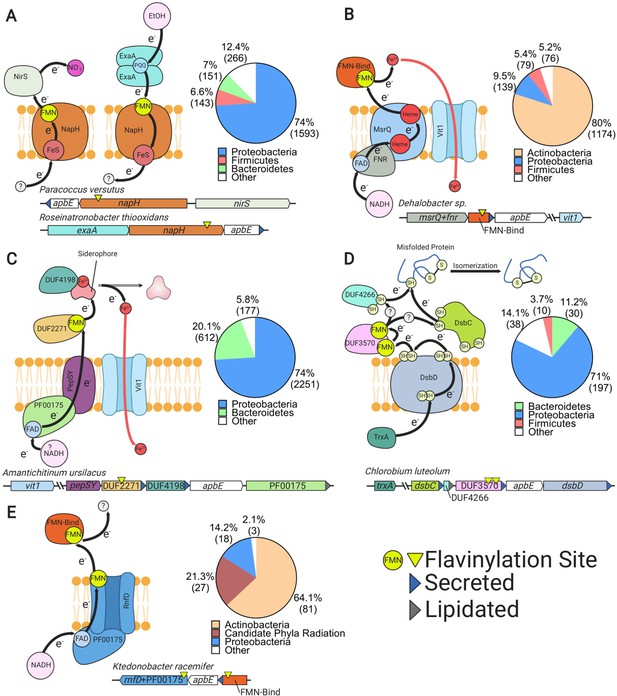
Uncharacterized extracytosolic electron transfer systems with an ApbE-flavinylated subunit.
Representative gene clusters and hypothesized models of the electron transfer systems they encode: (A) NapH-like (Moreno-Vivián et al., 1999), (B) MsrQ-like (Juillan-Binard et al., 2017), (C) PepSY (Yeats et al., 2004), (D) DsbD (Bushweller, 2020), and (E) RNF/NQR-like (Steuber et al., 2014). System names are based on sequence homology of a membrane protein encoded within the cluster to the referenced protein (see main text for additional context). Black arrows trace proposed electron transfer pathways. Accompanying pie charts show the number of genomes within each phylum that encode the electron transfer system. ‘Secreted’ and ‘lipidated’ refer to the presence of computationally predicted signal peptides and lipidation sites, respectively.
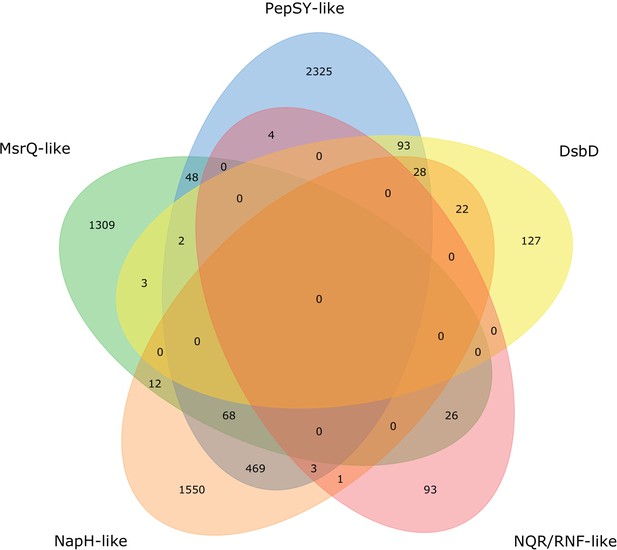
Distribution of uncharacterized extracytosolic electron transfer systems within prokaryotic genomes.
Venn diagram showing the number of uncharacterized systems (summarized in Figure 4) within the 6183 genomes that have at least one of the uncharacterized extracytosolic electron transfer systems.
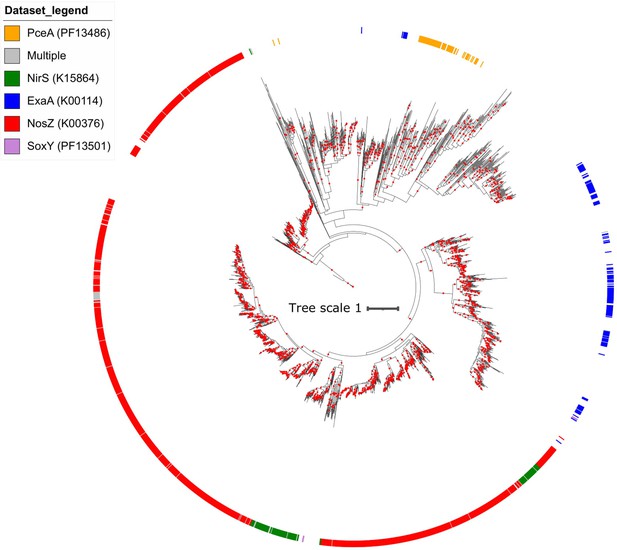
Maximum likelihood phylogeny of flavinylation-associated NapH-like proteins.
The tree was inferred using an LG + F + I + G4 model of evolution. Scale bar indicates the mean number of substitutions per site. Branches with bootstrap values ≥ 95 are indicated by red circles. The outer ring indicates the genes associated with the gene clusters.
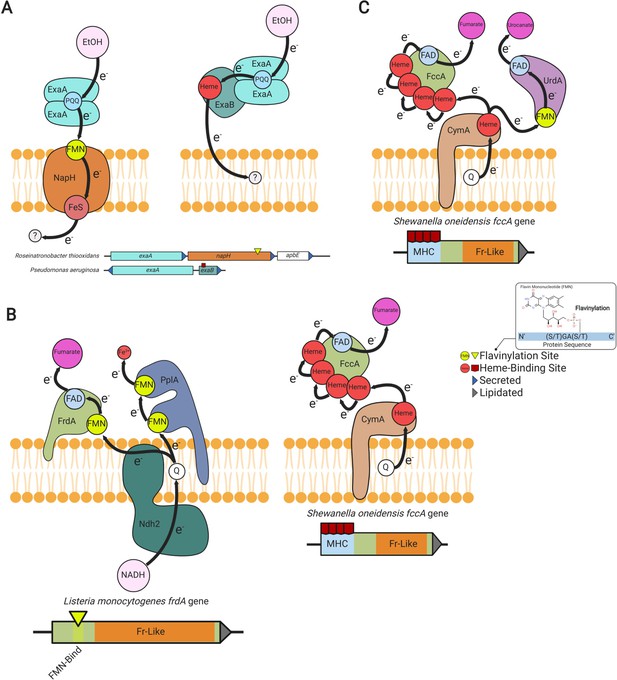
Modular flavinylation/cytochrome usage in extracytosolic electron transfer.
(A) Model of the characterized Pseudomonas aeruginosa (Görisch, 2003; Matsutani and Yakushi, 2018) and uncharacterized Rhiobacillus thiooxidans exaA alcohol oxidase gene clusters and the proposed electron transfer pathways they encode. (B) Domain structure of Listeria monocytogenes and Shewanella oneidensis fumarate reductases (FrdA and FccA, respectively) and a model of their characterized interactions with electron transfer pathways (Light et al., 2019; Kees et al., 2019). (C) Characterized electron transfer pathways for the S. oneidensis fumarate reductase-like enzymes, fumarate reductase (FccA), and urocanate reductase (UrdA) (Kees et al., 2019).
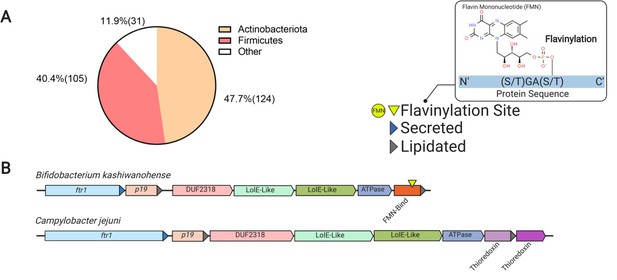
Flavinylation and thioredoxin-like proteins encoded in P19 gene clusters.
(A) Pie chart showing the number of genomes within each phylum with a P19-containing flavinylation-associated gene cluster. (B) Representative P19 gene clusters with thioredoxin-like or FMN-binding proteins. The Campylobacter jejuni cluster includes a mechanistically uncharacterized iron transporter and a thioredoxin-like protein (Liu et al., 2018b). P19 clusters in other genomes, such as Bifidobacterium kashiwanohense, lack the thioredoxin-like gene, but contain a gene with an FMN-binding domain.
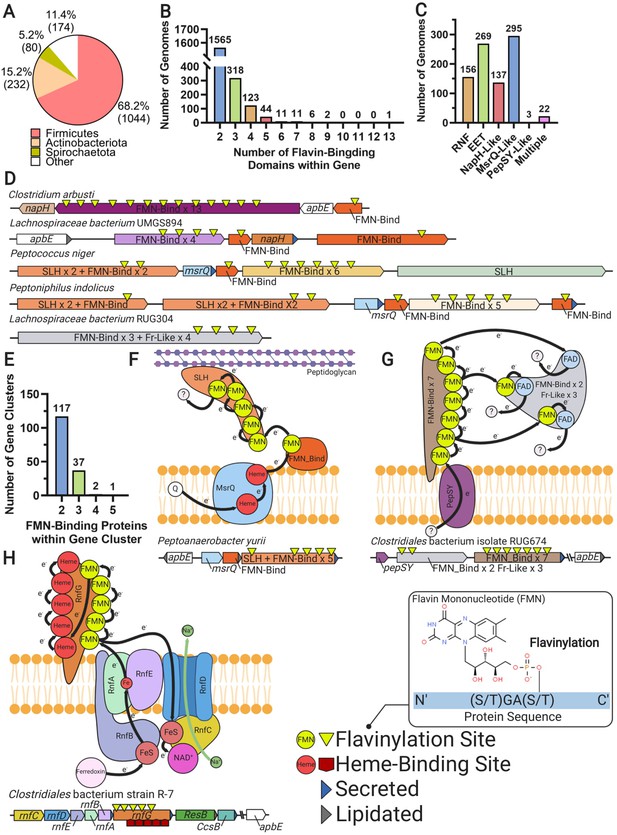
Multi-flavinylated proteins may possess novel electron transfer properties.
(A) Pie chart showing the number of genomes within each phylum that contain a multi-flavinylated (i.e., >1 FMN-binding domain) protein. (B) Histogram showing the number of FMN-binding domains within identified multi-flavinylated proteins. (C) Histogram showing the number of multi-flavinylated protein gene clusters that encode one of the transmembrane electron transfer systems described in Figures 1 and 4. (D) Examples of multi-flavinylated protein gene clusters. Fumarate reductase-like domains are abbreviated as Fr-like. (E) Histogram showing the number of proteins containing at least one FMN-binding domain within multi-flavinylated protein gene clusters. (F) Model of a possible electron transfer path encoded by a representative multi-flavinylated gene cluster that includes a protein with a cell wall-binding domain. (G) Model of a possible electron transfer path encoded by a representative multi-flavinylated gene cluster that includes multiple Fr-like domains. (H) Model of possible bifurcated electron transfer pathway encoded by an RNF complex with a multi-flavinylated/multi-heme cytochrome RnfG.
Additional files
-
Supplementary file 1
Annotations of the flavinylation-associated gene clusters discussed in the study.
Gene clusters of the 10 flavinylated systems discussed in this study (RNF, NQR, Nos, extracellular electron transfer, organohalide respiration, NapH-like, MsrQ-like, NQR/RNF-like, DsbD/DsbD-like, and PepSY-like), the P19 system, DUF2271, DUF3570, and multi-flavinylated proteins. The five genes downstream and upstream of each key gene (column K) were identified and annotated using signal peptide prediction (column G), transmembrane helix prediction (column H), PFAM (column I), and KEGG databases (column J). Genome accession and taxonomy are presented in columns A and B, respectively. Open reading frame (ORF) accessions are shown in column C. Columns D and E correspond to the scaffold accession and the ORF coordinates on the scaffold (start, end, and strand). Column F indicates the size of ORFs in amino acids.
- https://cdn.elifesciences.org/articles/66878/elife-66878-supp1-v2.xlsx
-
Supplementary file 2
Summary of prevalence of characterized and uncharacterized systems in prokaryotic genomes.
- https://cdn.elifesciences.org/articles/66878/elife-66878-supp2-v2.xlsx
-
Supplementary file 3
Fumarate reductase-like associations with flavin mononucleotide (FMN)-binding domains and cytochromes.
- https://cdn.elifesciences.org/articles/66878/elife-66878-supp3-v2.xlsx
-
Transparent reporting form
- https://cdn.elifesciences.org/articles/66878/elife-66878-transrepform-v2.docx