Scaling of subcellular actin structures with cell length through decelerated growth
Figures
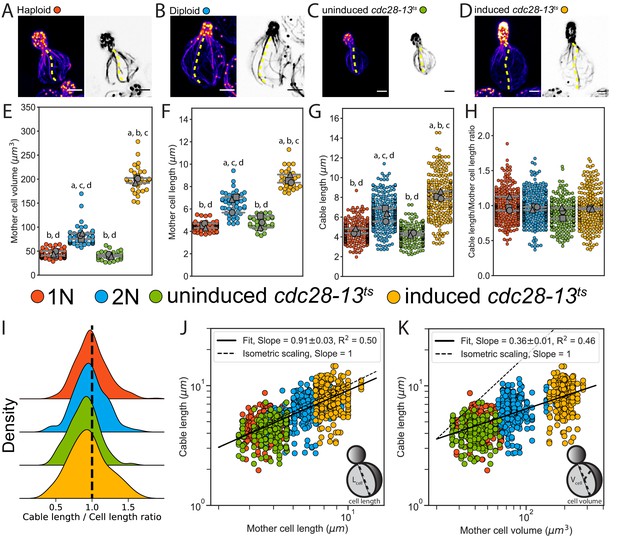
Actin cable length scales with cell length.
(A–D) Representative images of haploid (A), diploid (B), uninduced cdc28-13ts (C), and induced cdc28-13ts (D) cells fixed and stained with labeled-phalloidin. Lengths of single actin cables are indicated (dashed lines) in maximum intensity projections (left, color) and single Z planes (right, inverted). Scale bar, 2 µm. (E–F) Mother cell volume (E) and length (F) measured in three independent experiments (≥30 cells/strain). Each data point is from an individual cell. Larger symbols represent the mean from each experiment. (G–H) Cable length (G) and ratio of cable length/cell length (H) measured from the same cells as in E and F (≥200 cables/strain). Each data point represents an individual cable. Larger symbols represent the mean from each experiment. Error bars, 95% confidence intervals. Statistical significance determined by students t-test. Significant differences (p≤0.05) indicated for comparisons with haploid (‘a’), diploid (‘b’), uninduced cdc28-13ts (‘c’), and induced cdc28-13ts (‘d’). Complete statistical results in Figure 1—source data 1. (I) Probability density functions for ratios in H. (J–K) Cable lengths plotted against mother cell length (J) or volume (K) on double-logarithmic plots and fit using the power-law. Hypothetical isometric scaling (dashed line) is compared to experimentally measured scaling exponent (solid line).
-
Figure 1—source data 1
Complete results from statistical tests performed in this study.
The p-value is indicated for student’s t-test used to compare the data presented in Figure 1 and Figure 1—figure supplement 1.
- https://cdn.elifesciences.org/articles/68424/elife-68424-fig1-data1-v2.xlsx
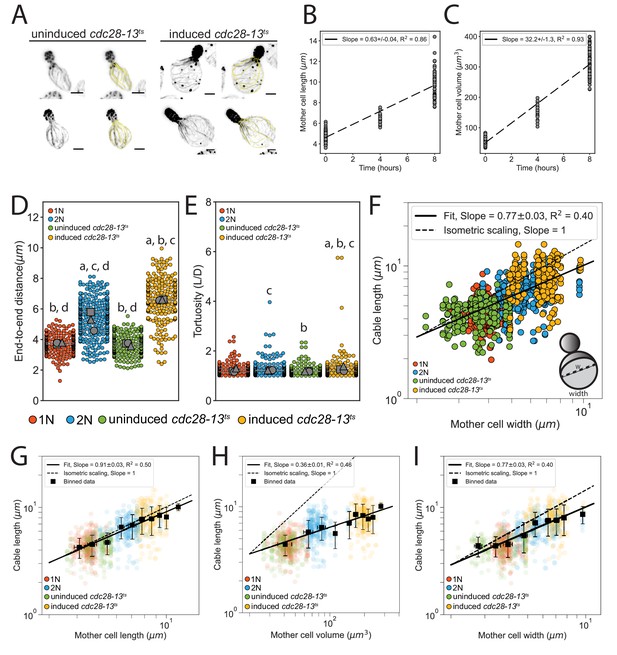
Changes to actin cable architecture in cells of different size.
(A) Representative images uninduced cdc28-13ts (left), and induced cdc28-13ts (right) cells fixed and stained with labeled-phalloidin. Lengths of all actin cables measured in each are indicated (yellow line). Scale bar, 2 µm. (B–C) Changes in mother cell length (B) and cell volume (C) of cdc28-13ts cells after growth at the restrictive temperature for the indicated times (≥20 cells/strain). Dashed line indicates results from linear regression. (C–D) End-to-end cable distance (C) and length/distance, or tortuosity (D) measured in three independent experiments (≥200 cables/strain). Each data point represents an individual cable. Larger symbols, mean from each experiment. Error bars, 95% confidence intervals. Statistical significance determined by students t-test. Significant differences (p≤0.05) indicated for comparisons with haploid (‘a’), diploid (‘b’), uninduced cdc28-13ts (‘c’), and induced cdc28-13ts (‘d’). Complete statistical results in Figure 1—source data 1. (E) Cable lengths plotted against mother width on a double-logarithmic plot, and fit using the power-law. This analysis indicated that scaling between cable length and cell width is hypoallometric (, in contrast with the observed isometric scaling between cell length and cable length (Figure 1J) Hypothetical isometric scaling (dashed line) is compared to experimentally measured scaling exponent (solid line). (F–H) Binned data (black squares, mean ± standard deviation) from Figure 1J and K and E.
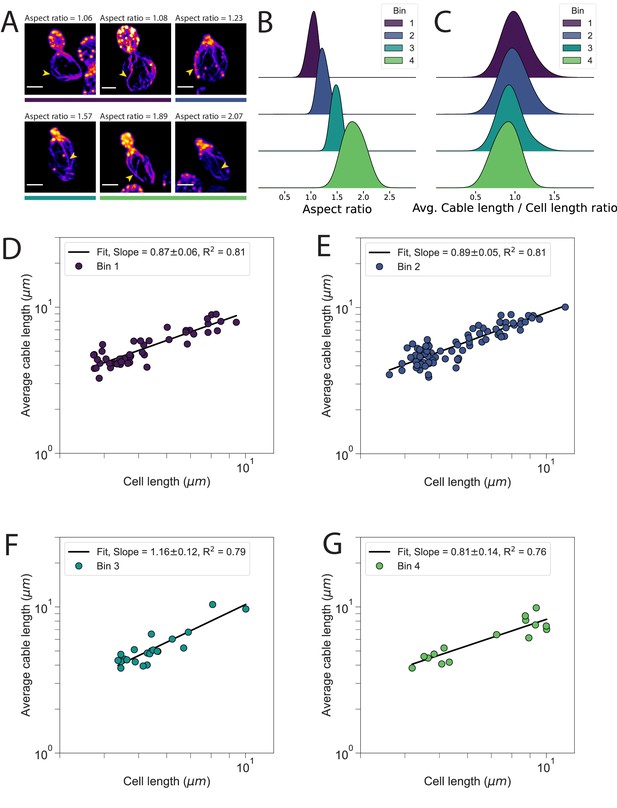
Changes to actin cable architecture in cells of different shape.
(A) Representative images of uninduced cdc28-13ts cells fixed and stained with labeled-phalloidin. The aspect ratio for each cell is indicated, and example actin cables that reach the rear of the mother cell are highlighted (yellow arrow). Colored bars beneath each image indicate the corresponding bin used for subsequent analyses. Scale bar, 2 µm. (B) Probability density functions for the aspect ratios of cells within each bin. (C) Probability density functions for the ratio of average cable length/cell length for each bin. Aspect ratio (B) and ratio of average cable length/cell length (C) measured from the same cells and cables as in Figure 1. (D–G) Average length of cables in a cell plotted against cell length, for each cell in each bin. Data are presented on double-logarithmic plots and fit using the power-law. Experimentally measured scaling exponent is indicated (solid line).
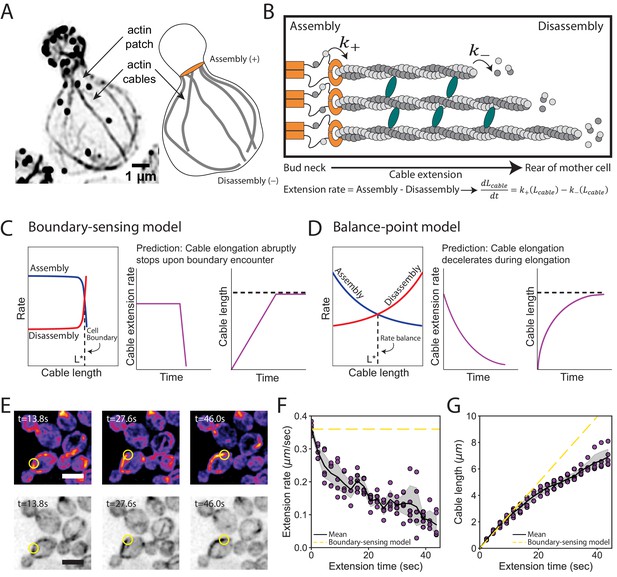
Models for control of actin cable length.
(A) Actin staining in haploid cell (left) and cable traces (right). (B) Relevant parameters and equation for cable extension, where assembly () and disassembly () rates change as a function of cable length. Cables are polymerized by formins (orange) from actin monomers (gray), bundled by crosslinkers (blue), and disassembled by factors not shown. Cable extension rate is the difference in assembly and disassembly rates. (C–D) Two models for cable length control. Additional information in Figure 2—figure supplement 1. (E) Maximum intensity projection of haploid cells expressing cable marker (Abp140-GFPEnvy) shown in color (top panels) and inverted gray scale (bottom panels). Yellow circle highlights tip of elongating cable over time. Scale bar, 5 µm. (F–G) Extension rate (F) and length (G) measured in five independent experiments (n = 82 cables). Symbols at each time point represents mean for individual experiment. Solid lines and shading, mean and 95% confidence interval for all five experiments. Dashed yellow lines, predictions of boundary-sensing model in C.
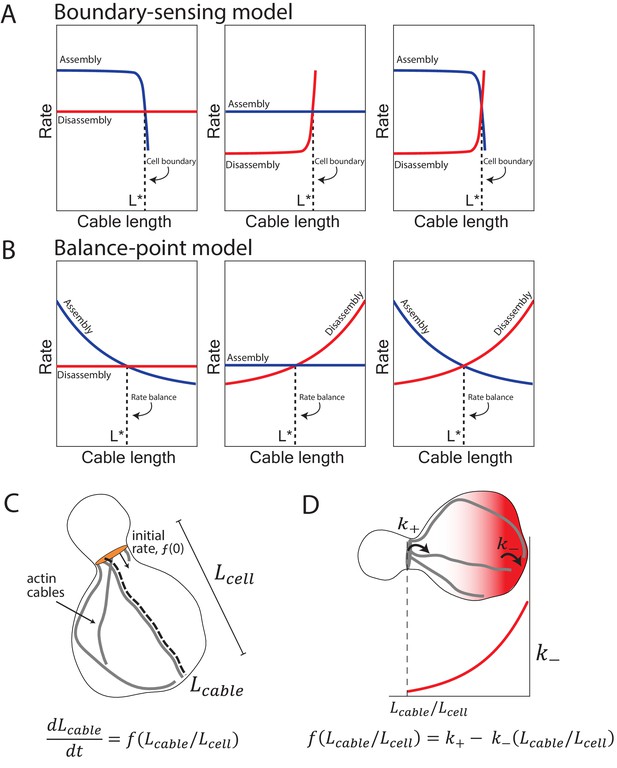
Boundary-sensing and balance-point models of length regulation.
(A) The boundary-sensing model proposes that cable length scales with mother cell length due to the growing tip of the cable physically encountering the rear of the mother cell (cell boundary). In this model, cable assembly and disassembly rates are length-independent until the boundary is reached. As the cable is growing (extending), the assembly rate must be greater than the disassembly rate. When the cable encounters the boundary, there is an abrupt shift in rates to prevent further growth of the cable: the assembly rate rapidly decreases (left panel), the disassembly rate rapidly increases (middle panel), or both rates change (right panel). Based on these changes to the assembly and/or disassembly rates, the boundary-sensing model predicts that the rate of cable extension remains constant until the cable physically encounters the rear of the mother cell, and then it abruptly decreases (Figure 2C). This behavior results in a cable that grows linearly with time until it reaches the boundary (Figure 2C). (B) In contrast, in balance-point model, the assembly rate (left panel), the disassembly rate (middle panel), or both rates (right panel) are length-dependent, and the intersection of these two rates (rate balance) produces a steady-state cable length (dashed line). Therefore, the balance-point model predicts that the cable extension rate will decelerate as a cable grows longer (Figure 2D). In the balance-point model, changes in cable length are expected to be greater during initial time points in a cable’s growth, and gradually decline until the steady-state length is reached (Figure 2D, dashed line). (C) Cartoon of yeast cell with actin cables (gray) extending from formins (orange) at the bud neck. Relevant parameters and mathematical form for the derived balance-point model are indicated, where the change in cable length as a function of time is controlled by the feedback function, (additional details in Materials and methods). (D) Cartoon depiction of a mechanism where actin cable length is scaled with cell length by a constant rate of cable assembly (), and a variable rate of disassembly () controlled by a gradient of depolymerizing activity (red shading). The gradient is highest at the back of the mother cell, which leads to the disassembly rate (red line, lower plot) increasing as the cable lengthens. By this mechanism, the cable length will scale with cell length.
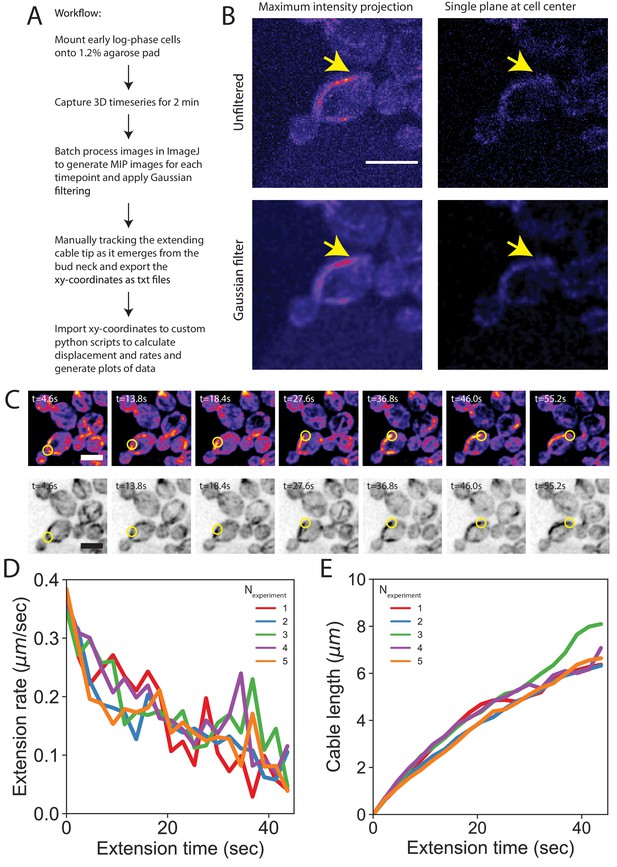
3D timeseries imaging workflow.
(A) Yeast cells grown to early log-phase were mounted onto 1.2% agarose pads made with synthetic complete media and imaged on a spinning disk confocal microscope. 3D stacks were acquired at 0.3 μm intervals for approximately half of the cell height with no time delay for 2 min (approximately 0.30–0.43 frames per second). Images were processed using custom ImageJ macros to generate maximum intensity projections for each stack and apply a Gaussian blur (sigma = 1) to facilitate manual tracking of cable tips from the point where they emerged from the bud neck until they ceased extending. Individual trajectories were analyzed using custom Python scripts to compute the distance of cable tip extension between each frame, the rate of cable extension between each frame, and the total extension distance starting from initial growth at the bud neck. (B) Tracking the entire lifetime of a single cable was made possible by capturing 3D timeseries images in conjunction with our image analysis pipeline. Representative images of a single time point of a cell expressing the cable marker Abp140-GFPEnvy (integrated) show that 2D timeseries images do not have sufficient signal-to-noise ratio for long term cable tracking (upper right panel). Application of a Gaussian blur to the timeseries images helps enable reliable tracking of cables (bottom panels). Scale bar, 5 µm. (C) Maximum intensity projection of haploid cells expressing a cable marker (Abp140-GFPEnvy) shown in color (top panels) and inverted gray scale (bottom panels). Yellow circle highlights tip of an elongating cable over time. Scale bar, 5 µm. (D–E) Mean cable extension rates (D) and mean cable lengths (E) for the five independent experiments shown in Figure 2F and G (n = 82 cables). Line color indicates the mean of each replicate.
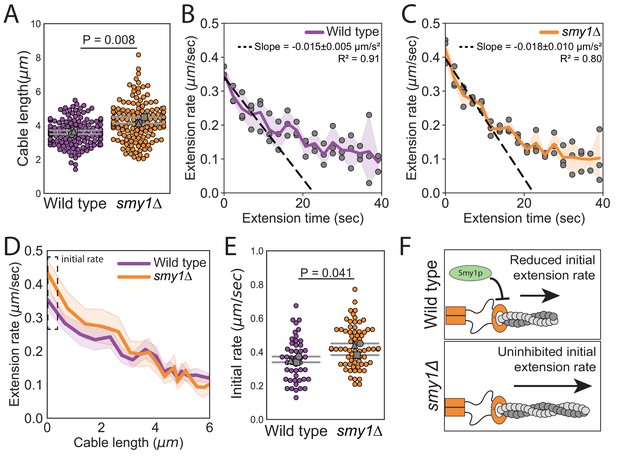
Smy1 controls initial cable extension rate.
All data are from three independent experiments. (A) Cable lengths (≥130 cables/strain). Each data point represents an individual cable. Larger symbols, mean from each experiment. Error bars, 95% confidence intervals. Statistical significance determined by students t-test. (B–C) Cable extension rates for wildtype (B) and smy1∆ (C) yeast (≥47 cables/strain). Symbols, mean from each experiment. Solid lines and shading, mean and 95% confidence interval for all experiments. Deceleration rates were derived from the slopes (±95% CI) of the dashed lines, which were determined by linear regression using the first ~10 s of extension. (D) Average extension rate as a function of cable length. Solid lines and shading, mean and 95% confidence interval for all experiments. Dashed box highlights region of no overlap in confidence intervals. (E) Initial cable extension rate for each strain. Small symbols, individual cables. Larger symbols, mean from each experiment. Error bars, 95% confidence intervals. Statistical significance determined by students t-test. (F) Cartoon comparing cable extension in wildtype and smy1∆ cells.
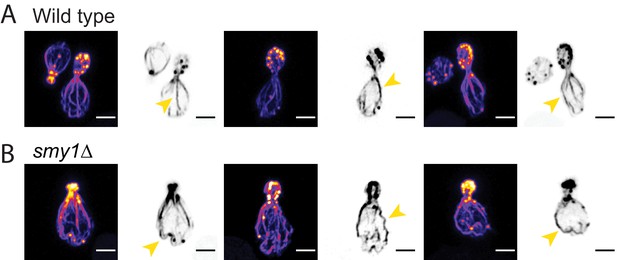
Altered actin cable length and architecture in smy1Δ cells.
(A–B) Representative images of fixed haploid wild-type (A) and smy1Δ (B) cells stained with fluorescent phalloidin to label F-actin. Actin cables in wildtype cells grow to reach the back of the mother cell and have a relatively straight appearance (yellow arrows in A), whereas cables in smy1Δ are abnormally long and have a ‘wavy’ appearance (yellow arrows in B), in agreement with previous studies (Eskin et al., 2016; Chesarone-Cataldo et al., 2011). Scale bar, 2 µm.
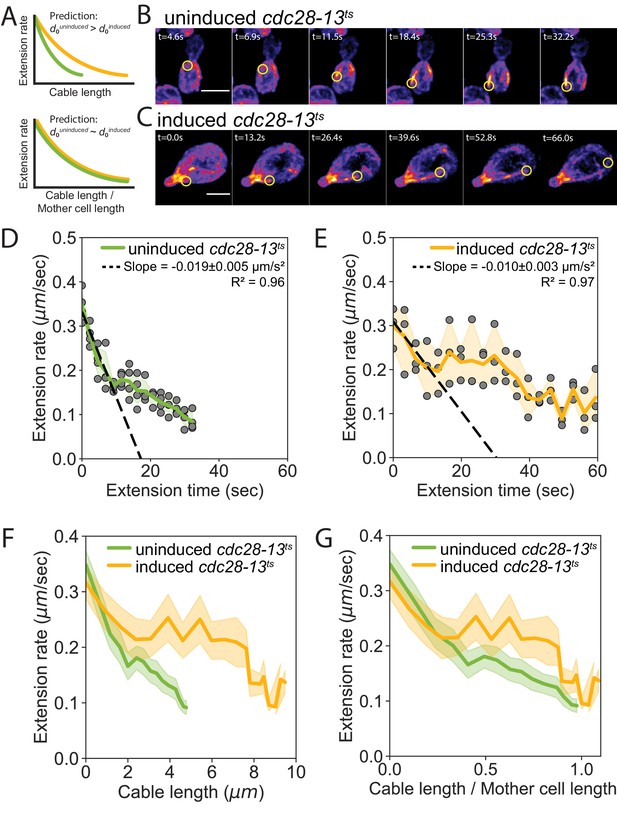
Cell length-dependent deceleration of actin cable growth.
(A) Predictions of balance-point model comparing how cable deceleration changes as a function of cable length (top graph) in shorter (green curve) and longer (yellow curve) cells. This difference in the deceleration profiles is eliminated when cable length is normalized to cell length (bottom graph). (B–C) Maximum intensity projections of uninduced (B) and induced cdc28-13ts (C) cells expressing cable marker (Abp140-GFPEnvy). Yellow circle highlights tip of elongating cable over time. Scale bar, 5 µm. (D–E) Cable extension rates for uninduced (D) and induced cdc28-13ts (E) cells, from at least three independent experiments (≥57 cables/strain). Symbols and shading, mean and 95% confidence intervals for all experiments. Deceleration rates were derived from the slopes (±95% CI) of the dashed lines, which were determined by linear regression using the first ~10 s of extension. (F–G) Average extension rates in uninduced and induced cdc28-13ts cells (data from experiments in D and E) plotted as a function of cable length (F), or the ratio of cable length/cell length (G). Solid lines and shading, mean and 95% confidence interval for all experiments.
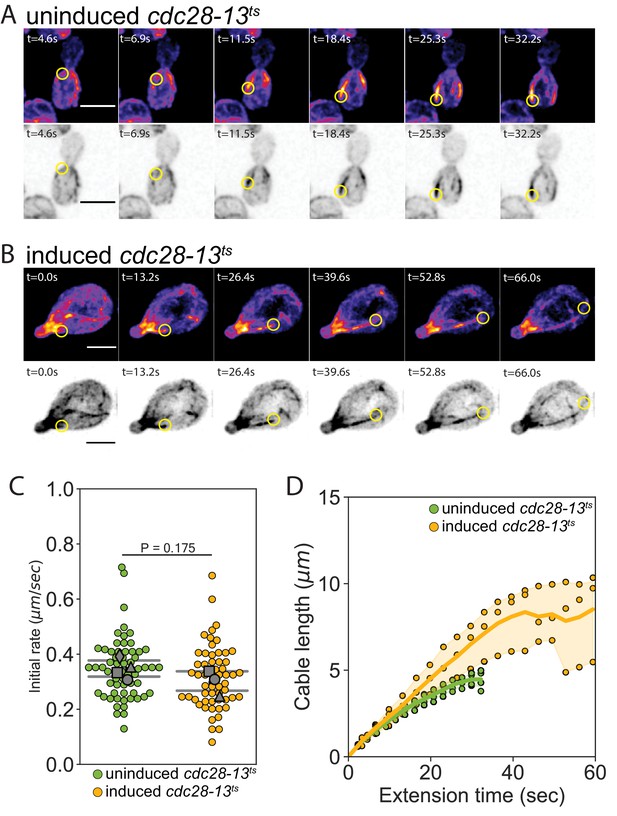
Cable extension dynamics are cell-length dependent.
(A–B) Maximum intensity projections of uninduced (A) and induced cdc28-13ts (B) cells expressing cable marker (Abp140-GFPEnvy) shown in color (top panels) and inverted gray scale (bottom panels). Yellow circle highlights tip of elongating cable over time. Scale bar, 5 µm. (C) Initial cable extension rate for uninduced (green) and induced cdc28-13ts (yellow) cells. Data are from live-cell imaging experiments as in Figure 4. Small symbols, individual cables. Larger symbols, mean from at least three independent experiments (≥57 cables/strain). Error bars, 95% confidence intervals. Statistical significance determined by students t-test. (D) Analysis of data from C, showing changes in actin cable length (D) plotted versus extension time. Symbols, mean from each experiment. Solid lines and shading, mean and 95% confidence interval for all experiments.
Videos
Maximum intensity projection of haploid cells expressing a cable marker (Abp140-GFPEnvy) shown in color.
Yellow circle highlights tip of an elongating cable over time. Video is played at 7 frames per second and time (seconds) is indicated in the top left corner. Scale bar, 5 µm.
Maximum intensity projections of uninduced cdc28-13ts cell expressing cable marker (Abp140-GFPEnvy) shown in color (top panels).
Yellow circle highlights tip of elongating cable over time. Video is played at 7 frames per second and time (s) is indicated in the top left corner. Scale bar, 5 µm.
Maximum intensity projections of induced cdc28-13ts cell expressing cable marker (Abp140-GFPEnvy) shown in color (top panels).
Yellow circle highlights tip of elongating cable over time. Video is played at 7 frames per second and time (s) is indicated in the top left corner. Scale bar, 5 µm.
Maximum intensity projections of uninduced cdc28-13ts cells expressing cable marker (Abp140-GFPEnvy) shown in color (top panels).
Yellow circle highlights tip of elongating cable over time. Video is played at 7 frames per second and time (s) is indicated in the top left corner. Scale bar, 5 µm.
Tables
Reagent type (species) or resource | Designation | Source or reference | Identifiers | Additional information |
---|---|---|---|---|
Strain, strain background (S. cerevisiae) | See: Supplementary file 1 | This paper | NCBITaxon:4932 | Strains maintained in the Goode lab |
Chemical compound, drug | Alexa Fluor 488- phalloidin | Life Technologies | A12379 | |
Chemical compound, drug | Alexa Fluor 568-phalloidin | Life Technologies | A12380 | |
Recombinant DNA reagent | pFA6a-link-GFPEnvy-SpHis5 | PMID:25612242 | RRID:Addgene_60782 | |
Recombinant DNA reagent | pFA6a-TRP1 | PMID:9717241 | RRID:Addgene_41603 |
Additional files
-
Supplementary file 1
Yeast strains used in this study.
The genotype, source, and related data are indicated for each strain used in this study.
- https://cdn.elifesciences.org/articles/68424/elife-68424-supp1-v2.xlsx
-
Transparent reporting form
- https://cdn.elifesciences.org/articles/68424/elife-68424-transrepform-v2.pdf