Corollary discharge promotes a sustained motor state in a neural circuit for navigation
Figures
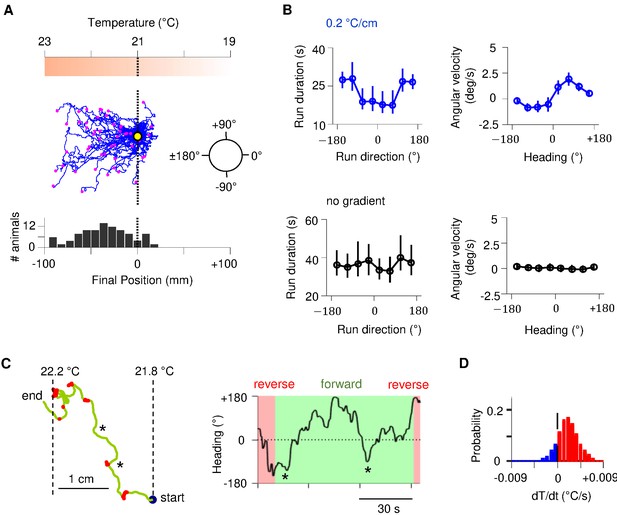
Sustained forward motor state despite temperature fluctuations during positive thermotaxis.
(A) Example trajectories of wild-type C. elegans cultivated at 25°C migrating up a linear temperature gradient over 20 min. Top: schematics of the thermal gradient. Middle: trajectories of 49 animals during positive thermotaxis. The starting points of all trajectories are aligned (yellow dot) and the end points are marked by magenta dots. Bottom: a histogram of the final location of animals. (B) Left column: duration of forward runs as a function of their overall direction (vector pointing from the starting point to the end point of the run). Right column: instantaneous velocity during forward runs as a function of the instantaneous heading angle. Top row: data from animals exposed to spatial thermal gradients (top, N = 140). Bottom row: data from animals under constant temperature surfaces (bottom, N = 73). (C) Thermotaxis trajectory of a single animal during thermotaxis with alternating periods of forward movement and reversals (left), and the instantaneous heading angle over time during one extended period of forward movement within the trajectory (right). Asterisks denote periods where the heading direction pointed down the thermal gradient. (D) Histogram of temporal changes in temperature () experienced by animals during forward runs that ended up pointing up the temperature gradient. Data from N = 140 wild-type animals exposed to linear thermal gradient and N = 73 wild-type animals exposed to constant temperature of 21°C. Error bars are standard errors of the mean (s.e.m.).
-
Figure 1—source data 1
Thermotaxis assay data.
- https://cdn.elifesciences.org/articles/68848/elife-68848-fig1-data1-v3.xlsx
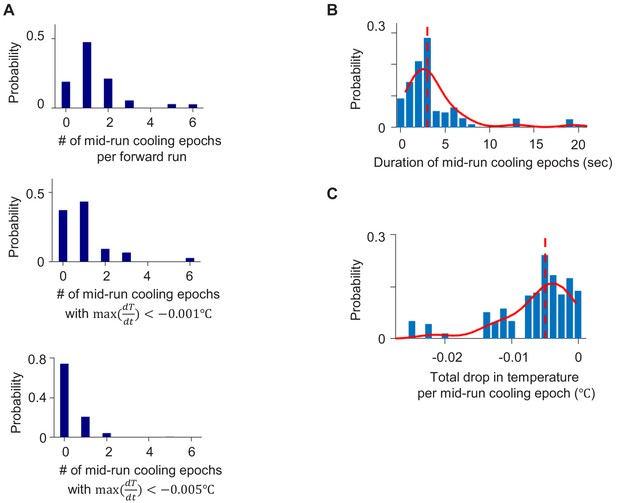
Distribution of thermal fluctuations experienced by C. elegans animals during positive chemotaxis.
(A) (Top) Distribution of the number of cooling epochs experienced during forward runs. Cooling epochs that occurred at the beginning or the end of forward runs were excluded. Same analysis carried out for cooling epochs where instantaneous temperature change was lower than −0.001°C (middle) or −0.005° (bottom) throughout the epoch. (B) Distribution of the duration of mid-run cooling epochs. (C) Distribution of the total drop in temperature experienced during mid-run cooling epochs. Data from N = 140 wild-type animals exposed to linear thermal gradients shown in Figure 1A.
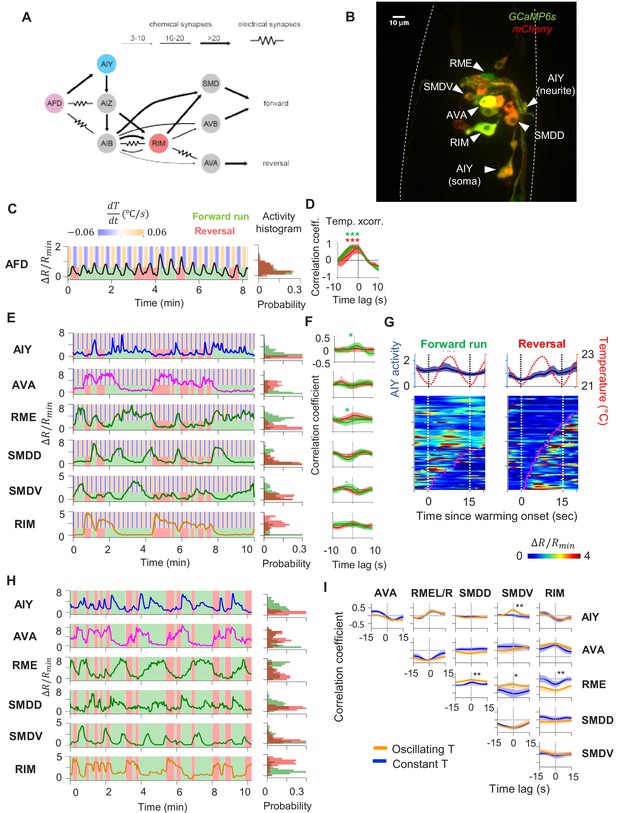
Characterization of circuit-level neural activity in the behaving animal with fluctuating or constant temperatures.
(A) Anatomical connections among neurons implicated in positive thermotaxis, locomotory control, or both. Connectivity is inferred from both the original C. elegans connectome (White et al., 1986) and a recent reconstruction of the connectome across the developmental time course (Witvliet et al., 2020) (see http://www.nemanode.org) (B) Example maximum projection of a confocal z-stack taken from a transgenic animal expressing GCaMP6s and mCherry in neurons examined in this study. (C) Example ratiometric calcium activity trace (left panel) and histogram (right panel) of the thermosensory neuron AFD in response to oscillating temperature. (D) Average cross-correlation function between thermal stimuli and AFD activity during forward run (green) or reversal (red) states. N = 5 wild-type animals. (E) Simultaneously measured calcium activity of interneurons and motor neurons involved in thermosensory processing and/or motor control. The activity traces (left) and activity histograms (middle) are from the same sample dataset. (F) Average cross-correlation functions between the thermal stimuli and individual neurons shown in (E), conditioned on the animal in forward run (green) or reversal (red) states. N = 6 wild-type animals. Error bars are 95% CI of the mean. Wilcoxon rank-sum test was used to compare the peak mean cross-correlation values during forward runs (green) versus reversals (red). *p<0.05; **p<0.01; ***p<0.001; no asterisk p>0.05. (G) Thermal stimulus-triggered activity of the AIY interneuron during forward runs (left column) and reversals (right column) in wild-type animals (N = 5). Individual stimulus epochs from the same neuron under the given motor state were concatenated into heat maps, with the average calcium activity trace shown on top. (H) Simultaneous recording of the activity of interneurons and motor neurons under constant temperature. The histograms on the right are derived from the sample activity traces to the left. (I) Pairwise cross-correlation functions among neurons examined in (E) and (H) under oscillating (orange, N = 6) or constant temperature (blue, N = 7). Error bars are standard errors of the mean (s.e.m.). Wilcoxon rank-sum test was used to compare the peak mean cross-correlation values between data under oscillating temperature versus data under constant temperature. *p<0.05; **p<0.01; ***p<0.001; no asterisk p>0.05.
-
Figure 2—source data 1
wild type circuit activity under thermal stimulation.
- https://cdn.elifesciences.org/articles/68848/elife-68848-fig2-data1-v3.xlsx
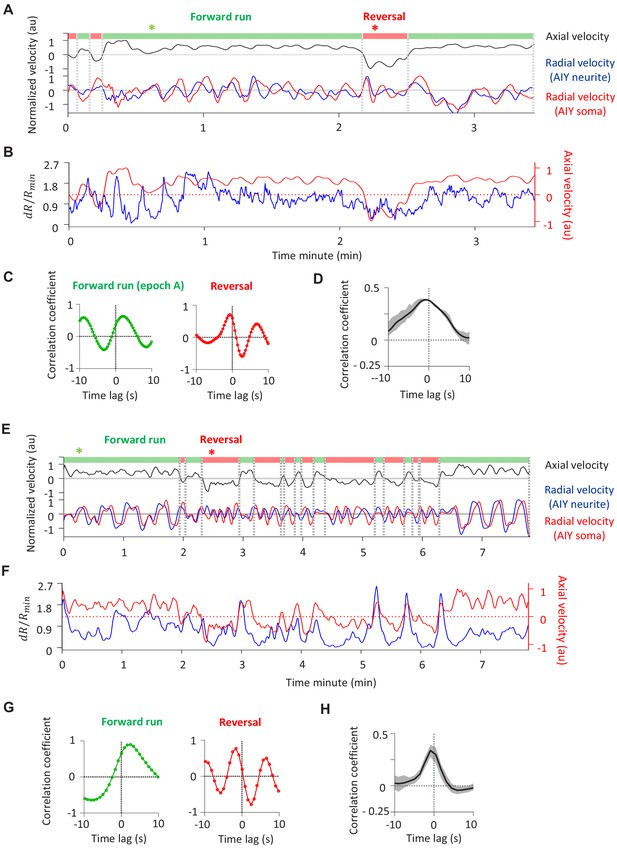
Behavior state annotation in free-moving and semi-constrained animals.
(A-C) Annotating forward runs and reversals in a free-moving animal. (A) Top: locomotory state can be read out either by directly measuring the axial velocity (i.e., velocity projected onto the animal’s body axis) of a landmark object (in this care, the AIY neurite, black trace) or by computing the cross-correlation of the radial velocities (i.e., component of the velocity orthogonal to the body axis) of two landmark objects located along the A-P axis (e.g., the AIY neurite [blue trace] and the more posterior AIY soma [red trace]), as illustrated in (C, D). (B) Calcium activity extracted from AIY neurite (blue trace) from the same recording as in (A), overlaid on its axial velocity (red trace, same as in A). (C) Cross-correlation functions corresponding to the forward run epoch (left panel) and the reversal epoch (right panel) marked with asterisks in (A). During the forward run, the measured velocity of the AIY neurite precedes that of the AIY soma; the opposite is true during reversal. (D) Average cross-correlation function between AIY calcium activity and the axial velocity of the AIY neurite, exhibiting positive correlation. Data are from N = 3 wild-type animals. (E–G) Annotating forward run and reversal states in a semi-constrained animal. (E) The axial velocity of the AIY neurite (black trace) and the radial velocities of the AIY neurite (blue) and the AIY soma (red). (F) Calcium activity extracted from AIY neurite (blue trace) from the same recording as in (A), overlaid on its axial velocity (red trace, same as in A). (G) Cross-correlation functions corresponding to forward run epoch (left panel) and reversal epoch (right panel) marked with asterisks in (E), with similar patterns as seen in (C). (H) Cross-correlation function between AIY calcium activity and the axial velocity of the AIY neurite, exhibiting positive correlation. Data are from N = 5 wild-type animals.
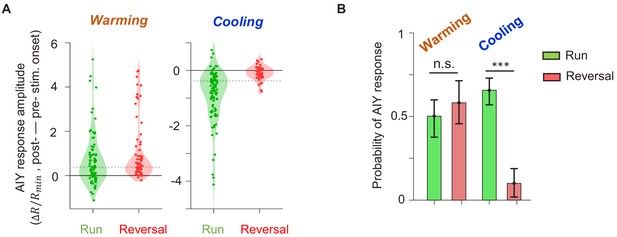
Thermal response of AIY under different locomotory states.
(A) Violin plots showing the distribution of changes in AIY activity in response to warming (left) or cooling (right) stimuli under forward run or reversal state in wild-type animals. Dotted black lines indicate the threshold values used to calculate the response probability in (B). (B) Probability that the magnitude of change in AIY activity upon warming or cooling is above the defined thresholds. See Materials and methods for details. For (A) and (B), data are from N = 5 wild-type animals.
Circuit-wide neural activity in semi-constrained wild-type animal exposed to oscillating temperature.
Left: calcium imaging of GCaMP6s (green) and wCherry (red) signals in head neurons involved in thermosensory processing or locomotory control. Neuron names are listed next to the neurite (AIY only) or soma (all other neurons) regions from which fluorescent signals are extracted. Video is sped up five times. Right: ratiometric calcium activity traces extracted from the video to the left. Background colors indicate the oscillating thermal stimuli (blue-orange) and the forward run (green) and reversal (red) states of the animal. Same dataset as in Figure 2E.
Circuit-wide neural activity in semi-constrained wild-type animal exposed to constant temperature.
Left: calcium imaging of GCaMP6s (green) and wCherry (red) signals in head neurons involved in thermosensory processing or locomotory control. Neuron names are listed next to the neurite (AIY only) or soma (all other neurons) regions from which fluorescent signals are extracted. Video is sped up five times. Right: ratiometric calcium activity traces extracted from the video to the left. Background colors indicate the forward run (green) and reversal (red) states of the animal. Same dataset as in Figure 2H.
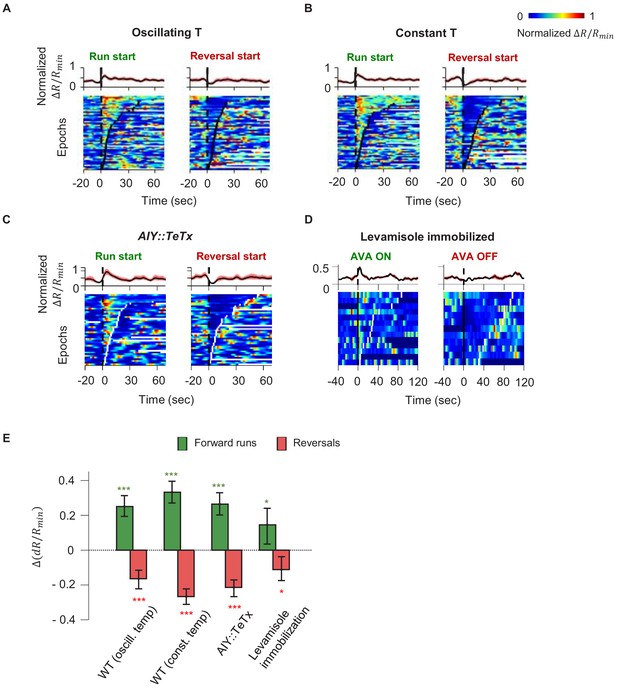
Motor-related activity in the AIY interneuron represents a corollary discharge signal.
(A, B) Calcium activity of the AIY interneuron aligned to the onset of forward runs (left column) or reversals (right column) in animals exposed to oscillating temperature (A, N = 6) or constant temperature (B, N = 5). Each row of the heat plots represents AIY calcium activity during a single behavioral epoch. The curve on top of each panel represents activity dynamics averaged across individual epochs. Broken lines indicate the onset and offset of each behavior epochs. (C) Calcium activity of the AIY interneuron aligned to the onset of forward runs or reversals in animals expressing tetanus toxin (TeTx) specifically in AIY (N = 4). (D) Calcium activity of AIY aligned to onset or offset of AVA activation in animals immobilized by the cholinergic agonist levamisole (N = 4). The ON and OFF states of AVA activity are defined by binarizing AVA activity using the Otsu method. See Materials and methods for details. (E) Change in AIY activity before versus. after the onset of forward runs (green) or reversals (red) for datasets shown in (A) and (B). Wilcoxon Signed rank test was used to test if the change in AIY activity has a median significantly different from zero, *p<0.05; **p<0.01; ***p<0.001; n.s., non-significant.
-
Figure 3—source data 1
AIY activity analysis - wild type.
- https://cdn.elifesciences.org/articles/68848/elife-68848-fig3-data1-v3.xlsx
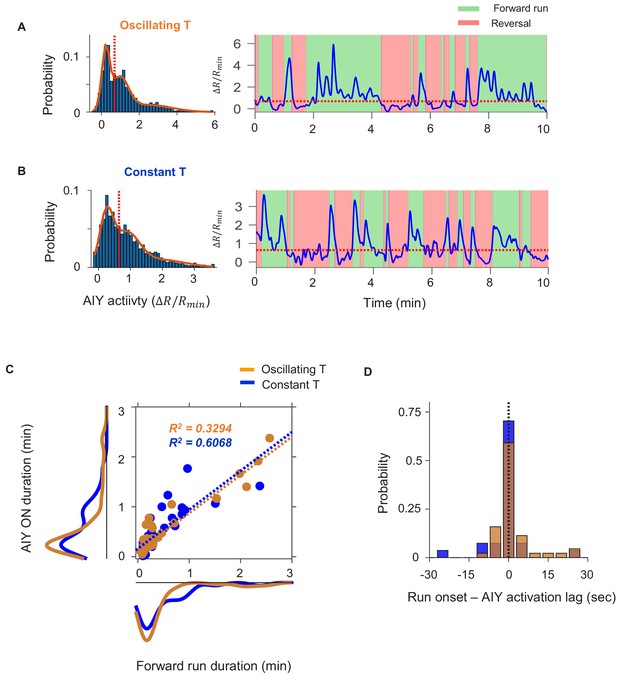
Quantification of persistent activity in AIY in wild-type animals.
(A, B) Definition of the AIY ON state. (A) Example histogram (left) and time-series trace (right) of AIY activity under oscillating temperature. Solid curve is derived by fitting the distribution with a Gaussian mixture model with three components. Dotted orange lines in both plots indicate the local minimum between the centers of the first two components of the Gaussian mixture model. Activity above the dotted orange line is classified as the ON state. (B) Same analyses as in (A) on a sample AIY activity trace measured under constant temperature. Activity above the dotted orange line is classified as the ON state. (C) The duration of the forward run state exhibits positive correlation with the duration of the AIY ON state under both oscillating and constant temperature. (D) Time lag between the onset of AIY ON states and the onset of forward runs for data collected under oscillating (orange) or constant (blue) temperatures. Positive values indicate that the AIY ON state followed the onset of the forward run.
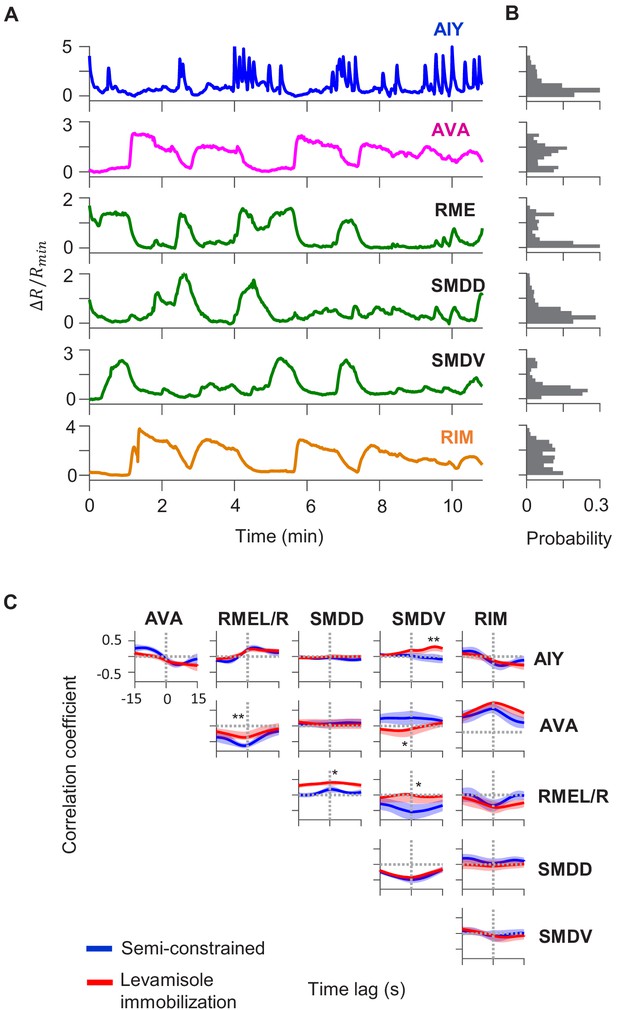
Circuit-level neural activity in immobilized animals.
(A) Simultaneously measured calcium activity in AIY and neurons of the motor circuit in an animal pharmacologically immobilized by levamisole. (B) Cross-correlation functions between the activity of AIY and that of simultaneously measured interneurons and motor neurons. (C) Average pairwise cross-correlation functions among neurons in wild-type animals under semi-constrained (blue) and pharmacologically immobilized (red) preparations. Data are from N = 4 wild-type animals. Error bars are standard errors of the mean (s.e.m.). Wilcoxon rank-sum test was used to compare the peak mean cross-correlation values between data under oscillating temperature versus data under constant temperature. *p<0.05; **p<0.01; ***p<0.001; no asterisk p>0.05.
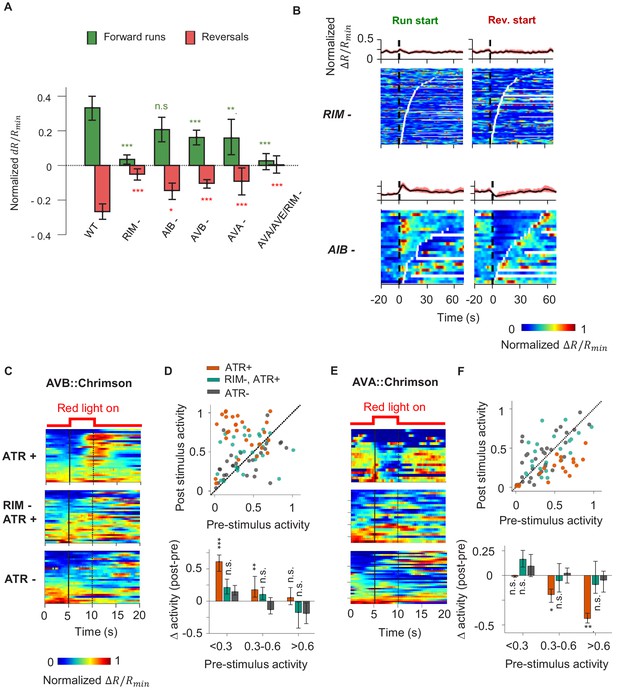
A corollary discharge (CD) pathway that requires the RIM interneuron couples AIY activity with the motor state.
(A) Change in AIY activity before versus. after the onset of forward runs (green) or reversals (red) in animals where candidate neurons for relaying the CD signal have been ablated. Data are from RIM-ablated animals (N = 9); AIB-ablated animals (N = 3); AVB-ablated animals (N = 4); AVA-ablated animals (N = 4); and AVA/AVE/RIM (nmr-1::miniSOG)-ablated animals (N = 4). Error bars are 95% CI. Wilcoxon rank-sum test was used to test if the distributions of AIY activity before and after the onset of motor states have the same median: *p<0.05; **p<0.01; ***p<0.001; no asterisk p>0.05. (B) AIY activity aligned to the onset of forward runs (left column) or reversals (right column) in the animals where neurons RIM (upper panels, N = 9) or AIB (lower panels, N = 3) have been genetically ablated. (C) AIY activity in response to optogenetic stimulation of the AVB premotor interneurons in wild-type animals grown on all-trans retinal (ATR) (top, N = 5), RIM-ablated animals grown on ATR (N = 6), and wild-type animals grown without ATR (N = 3). (D) Top: AIY activity 2.5 s before (pre-stimulus) versus 2.5 s at the end of the AVB opto-stimulation (post-stimulus) under experimental conditions shown in (D). Bottom: average change in AIY activity pre-and post-stimulation under experimental conditions shown (D). Trials are sorted into three groups based on pre-stimulation AIY activity. Same datasets as in (C). (E) AIY activity in response to optogenetic stimulation of the AVA premotor interneurons in wild-type animals grown on ATR (top, N = 4), RIM-ablated animals grown on ATR (middle, N = 5), and wild-type animals grown without ATR (bottom, N = 5). (F) Top: AIY activity 2.5 s before (pre-stimulus) versus 2.5 s at the end of the AVA opto-stimulation (post-stimulus) under experimental conditions shown in (E). Bottom: average change in AIY activity pre- and post-stimulation under experimental conditions shown in (E). Trials are sorted into three groups based on pre-stimulation AIY activity. Same datasets as in (E). For (E) and (F), error bars are 95% CI; Wilcoxon signed-rank test was used to test if the average post-stimulation change in AIY activity was significantly different from 0. *p<0.05; **p<0.01; ***p<0.001; no asterisk p>0.05.
-
Figure 4—source data 1
AIY activty analysis - mutant.
- https://cdn.elifesciences.org/articles/68848/elife-68848-fig4-data1-v3.xlsx
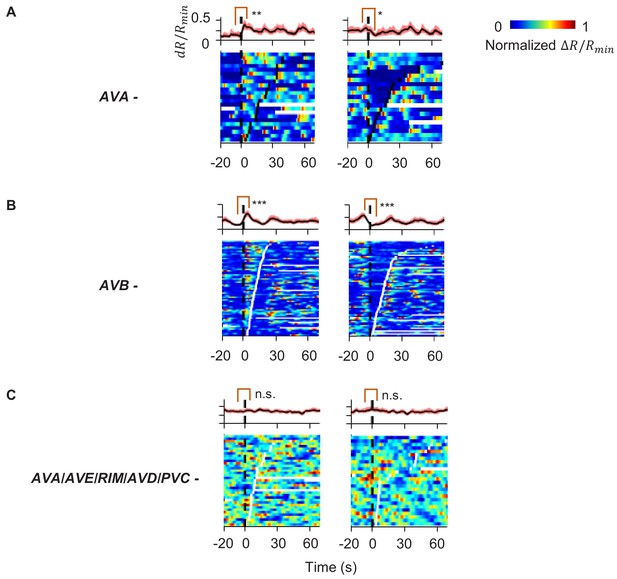
AIY activity aligned to behavioral states in animals where candidate neurons for relaying the corollary discharge signal have been ablated.
(A) AIY activity aligned to the onset of forward runs (left column) or reversals (right column) in the animals where the AVA interneurons have been genetically ablated (N = 4). (B) AIY activity aligned to the onset of forward runs (left column) or reversals (right column) in the animals where the AVB interneurons have been genetically ablated (N = 4). (C) AIY activity aligned to the onset of forward runs (left column) or reversals (right column) in the animals where multiple premotor interneurons (AVA, AVE, AVD, and PVC) and the RIM neurons have been genetically ablated (N = 9).
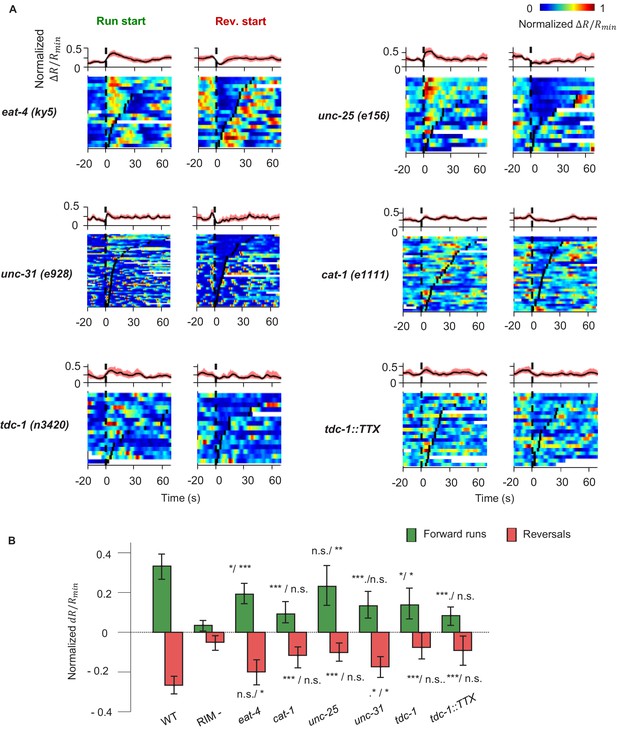
AIY activity aligned to motor states in mutant and transgenic animals defective in various modes of chemical transmission.
(A) AIY activity aligned to the onset of forward runs (left column) or reversals (right column) in VGLUT3/eat-4(ky5) mutants (N = 3); VMAT/cat-1(e1111) mutants (N = 3); GAD/unc-25(e156) mutants (N = 3); CAPS/unc-31(e69) mutants (N = 3); TDC/tdc-1(n3420) mutants (N = 4); transgenic animals expressing tetanus toxin (TeTx) specifically in the RIM and RIC neurons (N = 4). (B) Quantification of motor-related activity in AIY in mutants and transgenic animals presented in (A). Significance in comparison to wild-type and to RIM-ablated animals is presented side by side on top of each bar. Error bars are 95% CI. Wilcoxon rank-sum test, n.s., non-significant, *p<0.05; **p<0.01; ***p<0.001.
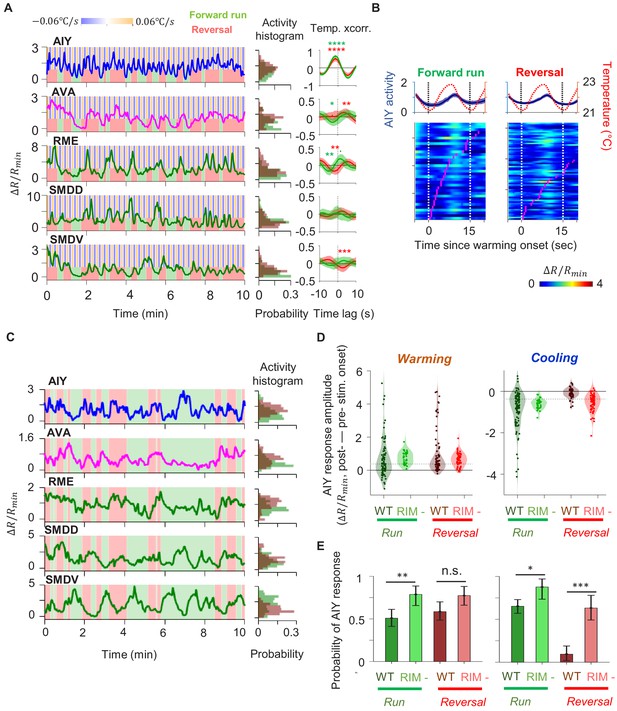
Characterization of circuit-level neural activity in behaving RIM-ablated animals under fluctuating or constant temperature.
(A) Simultaneously measured activity of AIY and neurons of the motor circuit in RIM-ablated animals under oscillating temperature. Middle panels show histograms of neuron activity during forward runs (green) or reversals (red) for the dataset to the left. Right panels show average cross-correlograms between neural activity and thermal stimuli during forward runs and reversals across RIM-ablated animals (N = 3). Error bars are 95% CI of the mean. Wilcoxon rank-sum test was used to compare the peak mean cross-correlation values during forward runs (green) versus reversals (red). *p<0.05; **p<0.01; ***p<0.001; no asterisk p>0.05. (B) Thermal stimulus-triggered activity of the AIY interneuron during forward runs (left column) and reversals (right column) in RIM-ablated animals (N = 3). Individual stimulus epochs from the same neuron under the given motor state were concatenated into heat maps, with the average activity trace shown on top. (C) Simultaneously measured activity of AIY and neurons of the motor circuit in RIM-ablated animals under constant temperature (left). Right panels show histograms of neuron activity during forward runs (green) or reversals (red). (D) Violin plots showing the distribution of changes in AIY activity in response to warming (left) or cooling (right) stimuli under forward run or reversal state in wild-type and RIM-ablated animals. Dotted black lines indicate threshold values used to calculate the response probability in (E). (E) Probability that the magnitude of change in AIY activity upon warming or cooling is above defined thresholds in wild-type or RIM-ablated animals. See Materials and methods for details. For (D) and (E), N = 5 wild-type animals and N = 3 RIM-ablated animals. Error bars are 95% CI of the mean. Wilcoxon rank-sum test *p<0.05; **p<0.01; ***p<0.001; n.s., non-significant.
-
Figure 5—source data 1
Circuit activity under thermoal stimulation in RIM ablated animals.
- https://cdn.elifesciences.org/articles/68848/elife-68848-fig5-data1-v3.xlsx
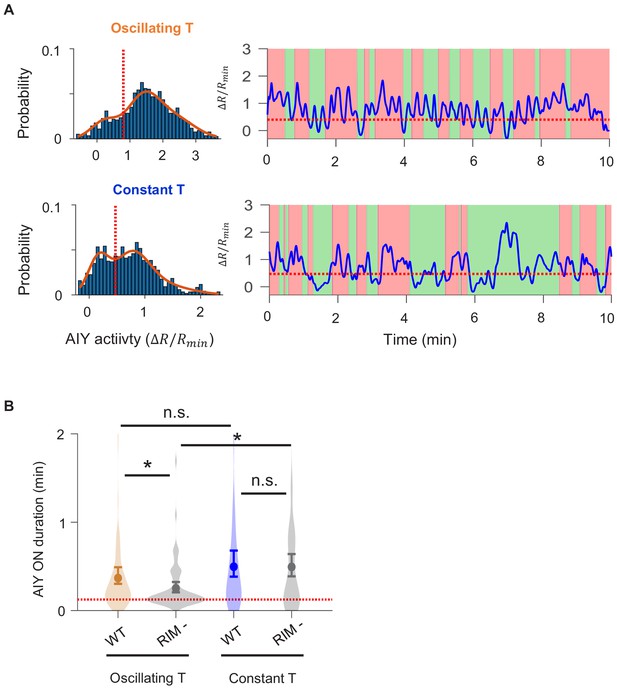
Analysis of AIY activity in RIM-ablated animals.
(A) Top: example histogram (left) and time-series trace (right) of AIY activity under oscillating temperature. Solid curve is derived by fitting the distribution with a Gaussian mixture model with three components. Dotted orange lines in both plots indicate the local minimum between the centers of the first two components of the Gaussian mixture model. Activity above the dotted orange line is classified as the ON state. See Materials and methods for details. Bottom: same analyses as in (A) on a sample AIY activity trace measured under constant temperature. Activity above the dotted orange line is classified as the ON state. (B) Violin plot showing the distributions of the duration of AIY ON states in wild-type versus RIM-ablated animals under oscillating and constant temperature. Data are from: N = 5 wild-type animals under oscillating temperature, N = 6 wild-type animals under constant temperature, N = 3 RIM-ablated animals under oscillating temperature, and N = 4 RIM-ablated animals under constant temperature. Wilcoxon rank-sum test *p<0.05; **p<0.01; ***p<0.001; n.s., non-significant.
Circuit-wide neural activity in semi-constrained RIM-ablated animal exposed to oscillating temperature.
Left: calcium imaging of GCaMP6s (green) and wCherry (red) signals in head neurons involved in thermosensory processing or locomotory control. Neuron names are listed next to the neurite (AIY only) or soma (all other neurons) regions from which fluorescent signals are extracted. Video is sped up five times. Right: ratiometric calcium activity traces extracted from the video to the left. Background colors indicate the oscillating thermal stimuli (blue-orange) and the forward run (green) and reversal (red) states of the animal. Same dataset as in Figure 5A.
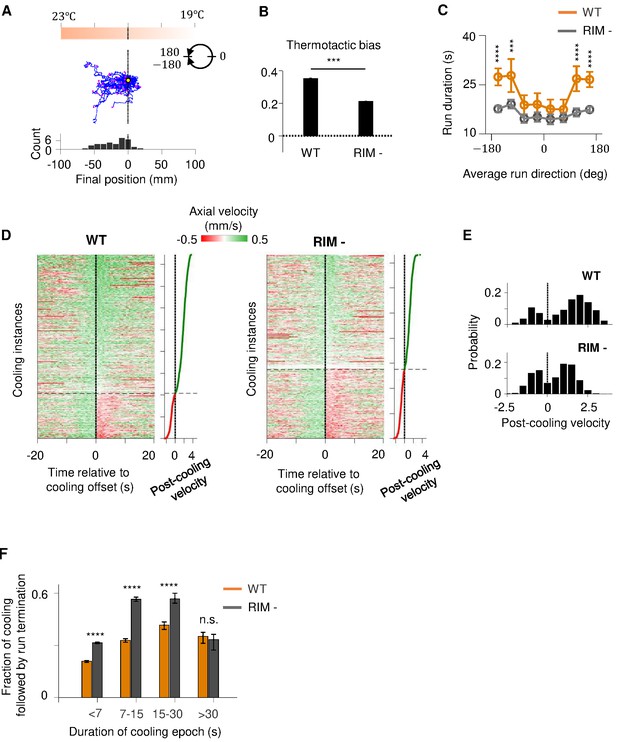
RIM ablation disrupts positive thermotaxis and leads to increased susceptibility to sensory fluctuations.
(A) Example trajectories of RIM-ablated animals (N = 39) cultivated at 25°C and exposed to the same thermal gradient as in Figure 1A. Top: schematic of the thermal gradient. Middle: trajectories of individual animals during positive thermotaxis. The starting points of all trajectories are aligned (yellow dot) and the end points are marked by magenta dots. Bottom: a histogram of the final location of the animals at the end of the 20 min period. (B) Average thermotactic bias of wild-type (N = 140) versus RIM-ablated animals (N = 102). (C) Forward run duration as a function of forward run direction in RIM-ablated animals (blue) compared to the wild type (gray). Error bars are standard errors of the mean (s.e.m.). Wilcoxon rank-sum test was used to compare the run duration of wild-type versus RIM-ablated animals. p<*0.05, **0.01, ***0.001, p>0.05 (non-significant) for panels without asterisk. (D) Velocity profiles of wild-type (left) and RIM-ablated (right) animals aligned to the end of cooling epochs that occurred during forward runs. Heat maps are generated by concatenating velocity profiles from individual cooling epochs along the y-axis and sorting by the average velocity within the first 2 s after the offset of cooling epochs (shown as line plot to the right). Black dotted lines divide instances in which forward runs continued past the offset of cooling epochs from instances where reversals ensued within the first 2 min of cooling offset. (E) Histograms of post-cooling velocities in wild-type (top) and RIM-ablated animals (bottom). Analysis applied to same dataset as in (D). (F) Fraction of cooling epochs that were followed by transition from forward runs to reversals as a function of the duration of cooling epochs (orange: wild type, gray: RIM ablated).
-
Figure 6—source data 1
Thermotaxis behavior in RIM ablated animals.
- https://cdn.elifesciences.org/articles/68848/elife-68848-fig6-data1-v3.xlsx
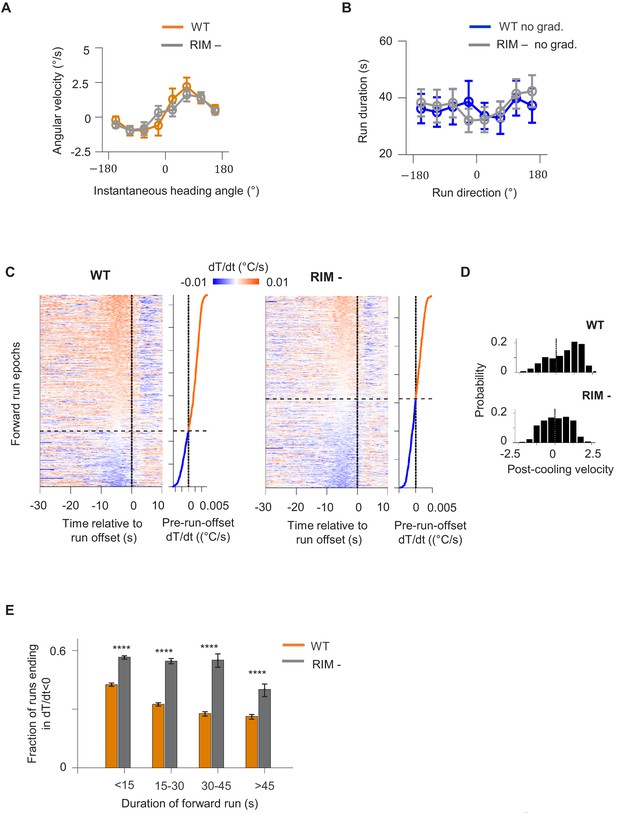
RIM ablation results in higher likelihood of forward runs ending after a period of cooling.
(A) Average angular velocity of wild-type (blue) and RIM-ablated (gray) animals as a function of their instantaneous heading angle. (B) Average forward run durations as a function of overall run direction in wild-type (blue) and RIM-ablated (gray) animals. Error bars are standard errors of the mean (s.e.m.). Wilcoxon rank-sum test was used to draw comparisons between wild-type and RIM-ablated animals. p<*0.05, **0.01, ***0.001, p>0.05 (non-significant) for panels without asterisk. (C) Instantaneous thermal variations experienced by wild-type (left) and RIM-ablated (right) animals aligned to the offset of forward runs. Heat maps are generated by concatenating time series along the y-axis and sorting by the average values within the last 2 s before the offset of forward runs (shown as line plot to the right). Black dotted lines divide instances in which forward runs ended after a period of warming from cases where runs ended after a period of cooling. (D) Histograms of values within the last 2 min before the end of forward runs in wild-type (top) and RIM-ablated animals (bottom). Analysis applied to same dataset as in (C). (E) Fraction of forward runs that ended after cooling as a function of the overall duration of the forward run (orange: wild type, gray: RIM ablated).
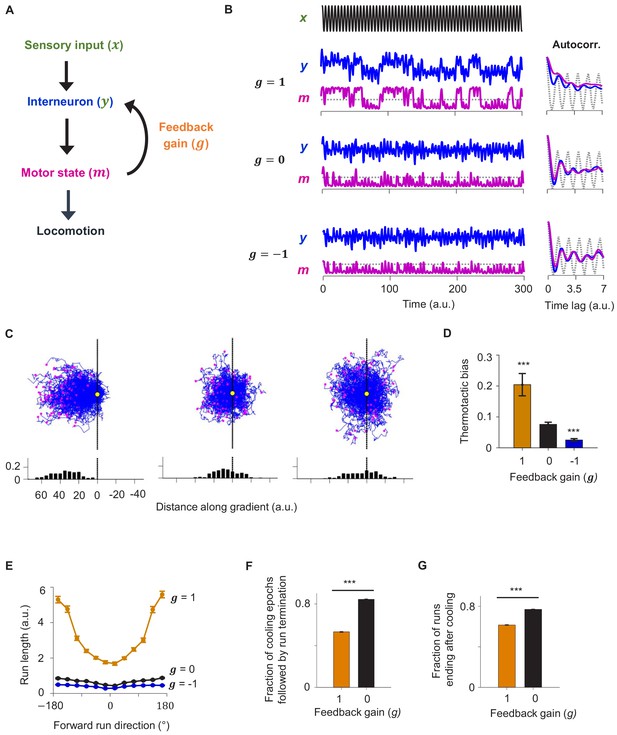
A reduced model explains the role of corollary discharge in sustaining forward locomotion during thermotaxis.
(A) Schematic of the circuit model. (B) Dynamics of the model in response to an oscillating input stimulus. Top: temporal profile of the input signal. Middle: dynamics of the model with the feedback strength set to 1 (positive feedback), 0 (no feedback), or −1 (negative feedback). (C) Simulated trajectories of navigational behavior on a 2-D arena with linear input gradient, with feedback strength set to 1 (left), 0 (middle), and −1 (right). (D) Thermotactic biases for trajectories generated by models with feedback strength equaling 1 (positive feedback), 0 (no feedback), or −1 (negative feedback). (E) Forward run duration as a function of forward run direction for the behavioral simulations in (D). (F) Fraction of cooling epochs that were followed by the termination of forward runs in simulations with the feedback gain set to 1 (blue) or 0 (black). (G) Fraction of forward runs that ended after a period of cooling in simulations with the feedback gain set to 1 (blue) or 0 (black). Error bars are 95% CI. Wilcoxon rank-sum test *p<0.05; **p<0.01; ***p<0.001.
-
Figure 7—source data 1
Computational model of the thermotaxis circuit.
- https://cdn.elifesciences.org/articles/68848/elife-68848-fig7-data1-v3.zip
Tables
Parameter values used in Figure 7B.
Parameter | Value |
---|---|
2/3 | |
2/3 | |
1 | |
1 | |
w0 | 1 |
−1, 0, or 1 | |
w21 | 1 |
k1 | 15 |
k2 | 5 |
k3 | 5 |
0 | |
1.5 | |
1.5 | |
1 | |
1 | |
C1 | 0.5 |
C2 | 0 |
Constructs and transgenic arrays.
Calcium imaging | |||
---|---|---|---|
Plasmid | Injection marker | Transgene | Strain |
pDACR1286[Pmod-1::GCaMP6s] (25 ng/µl); pDACR63[Pttx-3::mCherry ] (25 ng/µl) | pDACR218[Punc-122::dsRed] (40 ng/µl) | aeaIs003(AIY) (integrated olaEx1621*) | ADS003 |
pDACR943[Pgcy-8::GCaMP6s] (30 ng/µl);pDACR801 [Pgcy-8::mCherry] (5 ng/µl) | pDACR20[Punc122::GFP] (20 ng/µl) | aeaIs004 (AFD) (integrated olaEx1527)* | ADS004 |
pJH3338[Pglr-1-GCaMP6s::wCherry] | pL15EKlin-15AB genomic DNA ( 20 ng/µl) | hpIs471 (premotor/motor) | ZM8558 |
Optogenetic stimulation | |||
pHR2[Plgc-55B-Chrimson::wCherry] | pL15EK[lin-15AB genomic DNA] (80 ng/µl) | aeaEx003 (AVB/others) | ADS029 |
pHR6[Prig-3-Chrimson::wCherry] | pL15EK[lin-15AB genomic DNA] (80 ng/µl) | aeaEx005 (AVA/others) | ADS031 |
Cell ablation | |||
pJH2829[Pcex-1- MiniSOG::SL2::wCherry] | pL15EK[lin-15AB genomic DNA] (20 ng/µl) | hpIs327 (RIM) | ZM7978 |
pJH3311[Pinx-1- MiniSOG::SL2::wCherry] | pL15EK[lin-15AB genomic DNA] (20 ng/µl) | hpIs465(AIB) | ZM8484 |
pJH2931[Prig-3- MiniSOG::SL2::wCherry] | pL15EK[lin-15AB genomic DNA] (20 ng/µl) | hpEx3072 (AVA/others) | ZM7198 |
pJH2890[Plgc-55B- MiniSOG::SL2::wCherry] | pL15EK[lin-15AB genomic DNA] (20 ng/µl) | hpIs331(AVB/others) | ZM7297 |
pJH2890[Pnmr-1-MiniSOG::SL2::wCherry] | pL15EK[lin-15AB genomic DNA] (20 ng/µl) | hpIs321(AVA/E/D/RIM/PVC/others) | ZM7054 |
Synaptic manipulation | |||
Pttx-3::TeTx::mCherry Zhang et al., 2005 | yxIs25 (AIY) | ZC1952 | |
Ptdc-1::TeTx::mCherry Gordus et al., 2015 | kyEx4962 (RIM/RIC) | CX14993 |
-
*Gift of Daniel Colon-Ramos.
Strains.
For thermotaxis and locomotion assays | |||
---|---|---|---|
Strain | Genotype | Purpose | Figure |
Bristol N2 | Wild type | Wild-type behavior | Figure 1A–D |
ZM7978 | hpIs327 | Behavior upon RIM ablation | Figure 6 |
Calcium imaging | |||
ADS003 | aeasIs003 | AIY imaging | Figures 2E–I, 3A, B |
ADS004 | aeaIs004 | AFD imaging | Figure 2C, D |
ADS027 | aeaIs003; hpIs471 | Simultaneous imaging of AIY, AVA, RME, SMDD, SMDV, and RIM | Figure 2B, E |
ADS043 | aeaIs003; yxIs25 | AIY imaging, upon blockade of AIY chemical transmission | Figure 3B |
ADS010 | aeaIs003; hpIs327 | AIY imaging, upon ablation of RIM | Figures 4A, B, 5A, C |
ADS014 | aeaIs003; hpIs321 | AIY imaging, upon ablation of RIM, AVA, AVE, AVD, and PVC | Figure 4A |
ADS026 | aeaIs003; hpEx3072 | AIY imaging upon ablation of AVA | Figure 4A |
ADS036 | aeaIs003; hpIs331 | AIY imaging, upon ablation of AVB | Figure 4A |
ADS046 | aeaIs003; hpIs465 | AIY imaging, upon ablation of AIB | Figure 4A, B |
ADS029 | aeaEx003; aeaIs003; lite-1(ce314) | AIY imaging upon optogenetic stimulation of AVB | Figure 4C |
ADS031 | aeaEx005; aeaIs003; lite-1(ce314) | AIY imaging upon optogenetic stimulation of AVA | Figure 4E |
ADS033 | aeaEx005; aeaIs003; hpIs327; lite-1(ce314) | AIY imaging, upon RIM ablation and AVA stimulation | Figure 4E |
ADS035 | aeaEx003; aeaIs003; hpIs327; lite-1(ce314) | AIY imaging, upon RIM ablation and AVB stimulation | Figure 4C |
ADS013 | aeaIs003; kyEx4962 | AIY imaging, upon disruption of RIM/RIC chemical transmission | Figure 4—figure supplement 2 |
ADS006 | aeaIs003;tdc-1(n3419) | AIY imaging in tyramine/octopamine synthesis mutant | Figure 4—figure supplement 2 |
QW1411 | aeaIs003; eat-4(ky5) | AIY imaging in glutamate mutant | Figure 4—figure supplement 2 |
QW1175 | aeaIs003; unc-31(e928) | AIY imaging in dense core vesicle release mutant | Figure 4—figure supplement 2 |
QW1408 | aeaIs003; cat-1(e1111) | AIY imaging in biogenic amine transporter mutant | Figure 4—figure supplement 2 |