Robotic multi-probe single-actuator inchworm neural microdrive
Figures
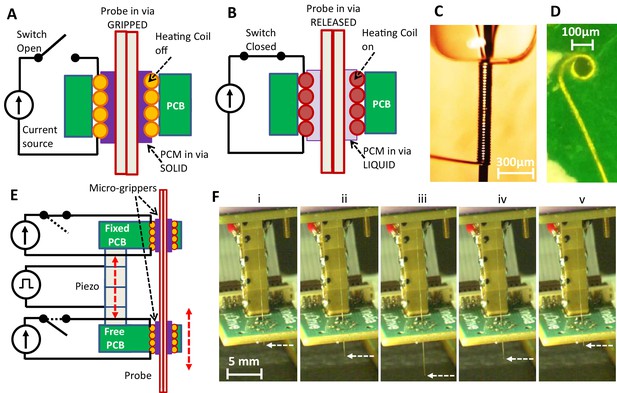
Microgripper and single-probe inchworm microdrive design.
(A–B) Side view of microgripper design. A resistive heating coil is installed in a printed circuit board (PCB) via. A probe is placed through the coil bore, and the bore is filled with a temperature-responsive phase change material (PCM). With the heating coil off (A), PCM is in the solid state, and the probe is ‘gripped’. When the coil is on (B), PCM is in the liquid state, and the probe in the microgripper is ‘released’ and can be moved axially through the bore. The PCM can be maintained in the microgripper through capillary action and does not exit the bore. (C) Photograph of the fabricated heating coil before it is placed into the board. (D) Photograph of the top view of the heating coil installed into the PCB. Schematic (E) and photos (F) of the inchworm motor. Two parallel PCBs, each with the installed microgrippers, are aligned along the probe axis and joined by a single piezo actuator. The top board is fixed while the bottom board is movable by the motion of the voltage-controlled piezo actuator. Sequential electronically controlled probe gripping and piezo extension and contraction (described in Figure 2) are used to translate the probe (white dashed arrow points to the probe tip) in either direction (up or down), as shown in F(i)–(v) and Video 1.
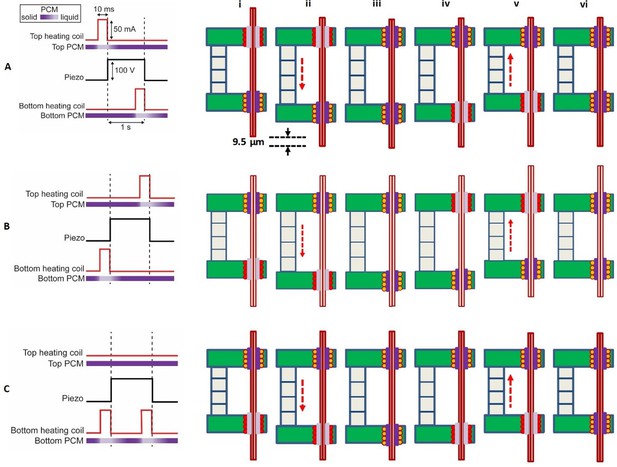
Sequence of electronic actuation steps used by the microdrive for a single translation step.
(A) A single downward step (here 9.5 µm) of the probe is performed by six inchworm motor phases (i)–(vi). Long-distance downward probe translation can be accomplished through an unlimited number of repeated single step sequences. In this schematic, the top gripper board is fixed, while the bottom gripper board moves with the piezo extension or contraction. Positive voltage applied to the piezo causes it to extend. (B) A sequence change in the activation of the microgrippers results in a single upward step. Importantly, the actuation signal to the piezo remains the same. (C) Single no-step sequence, applied to those probes that are to remain in place while other probes are moved in a multi-probe microdrive. The piezo is still actuated, extending and contracting as in (A) and (B), but the sequence of the actuation signals to the top and bottom board heaters is such that the probe does not move. Pulse timing is not shown to scale.
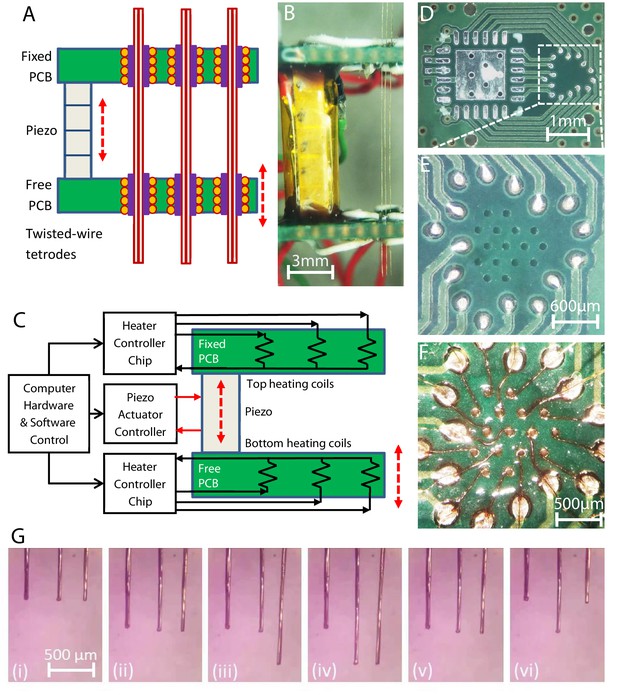
Multi-probe translation capability.
(A) A multi-probe single-actuator (MPSA) microdrive with independently movable probes. Axially aligned pairs of independently controlled microgrippers are integrated into the top and bottom microgripper PCBs. The top board is fixed, and the bottom board is free to be moved by the piezo. (B) Side view of a model MPSA microdrive loaded with three tetrodes (right). (C) Schematic of the electronic circuitry. Independent current sources are electrically connected to heater coils in the vias, while hardware and software control the sequence of electronic actuation signals. (D–F) An example unpopulated printed circuit board (D) with 16 drilled vias (E) for placement of 16 microgrippers in a hexagonal close packed arrangement (F), with 300 μm center-to-center spacing. Demonstration of multiple independent probe movements is shown in Video 2 and (G): (i) with all three probes aligned in height, (ii) all three probes moved together downward, (iii) just the right probe is moved downward, while the left and middle are held stationary, (iv) just the middle probe is moved downward, while the left and right are held stationary, (v) the right and middle probes are moved together upward, while the left is held stationary, (vi) the right and left are moved upward, while the middle electrode is held stationary. The piezo actuator always receives the same actuation sequence of signals throughout these different independent electrode translation options (down step, up step, and no step sequences of Figure 2). Forty-two piezo steps were taken between each consecutive image.
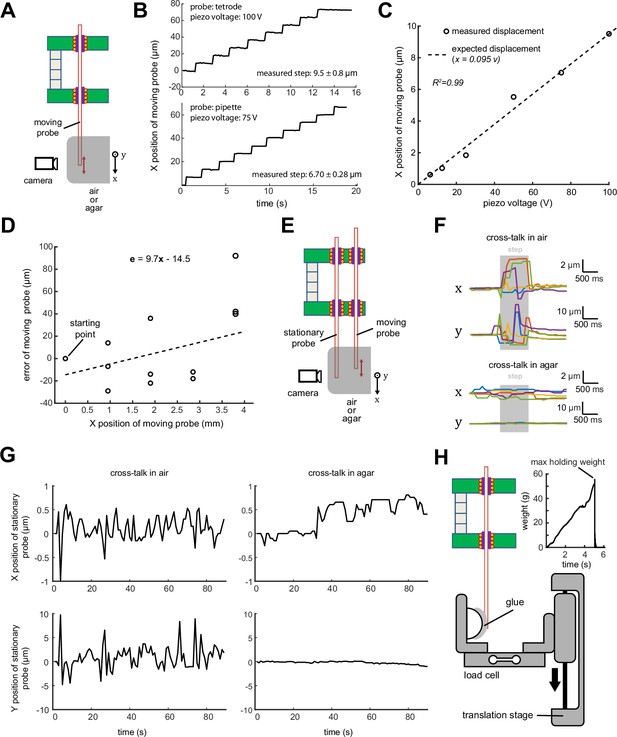
Multi-probe single-actuator (MPSA) microdrive mechanical characterization.
(A) Experimental setup diagram: microdrive is clamped such that probes move in the field of view of the camera or microscope. In some experiments, probe tips were embedded in agar. Motion in the X direction indicates ‘on-axis’ in the direction of the probe, motion in the Y direction indicates ‘off-axis’ lateral motion (in and out of the page). (B) Top: measured stepwise motion of a tetrode loaded into the microdrive. Step size was set to 9.5 µm (maximum piezo voltage). Bottom: measured stepwise motion of a micropipette loaded into the microdrive. Step size was set to 6.7 µm (75 V piezo voltage). Motion was imaged at two frames per second. (C) Correspondence between piezo command voltage and measured tetrode step size. (D) Accuracy of probe motion over 4 mm travel range. (E) Experimental setup for measuring cross-talk between a moving probe and a stationary neighboring probe. (F) Representative displacements in X (on-axis) and Y (off-axis) of a stationary pipette during a single step of a neighboring probe. Pipettes moving in agar brain phantoms (bottom) exhibited smaller displacements than those moving in air (top). Step duration is marked in gray and corresponds to actuation of the piezo stack. N=5 steps are shown. (G) Long-term drift of stationary probe during continuous stepping motion of moving probe (9.5 µm step size). (H) Experimental setup for measuring maximum holding weight of a single microgripper. A translation stage moved the load cell until the tetrode came loose from the microgripper. The maximum holding weight was considered to be the weight supported by the microgripper immediately before breaking (inset).
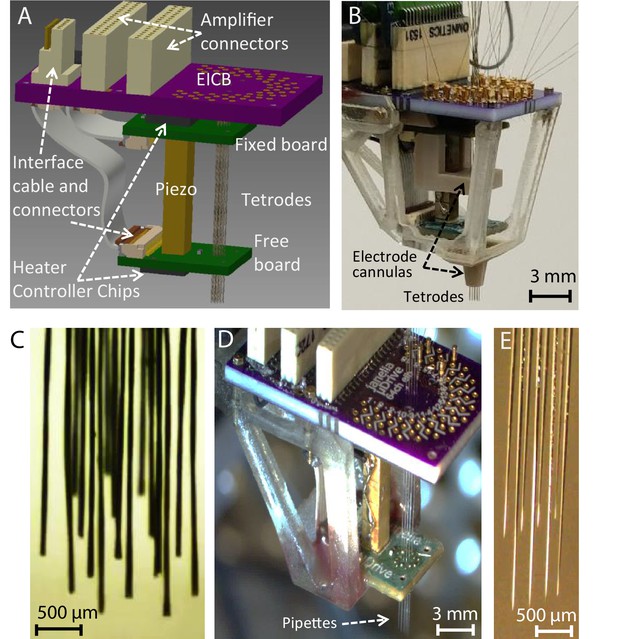
Implantable multi-probe single-actuator (MPSA) microdrives for neural recordings.
(A) Schematic showing the main MPSA microdrive with the added electrode interface and control board (EICB). (B) Photograph of the implantable 16-tetrode-drive for acute neural recordings. Cannulas were added to increase tetrode stiffness during insertion. A three-dimensional-printed cage was added on the exterior for protection and ease of handling. The tetrode-drive was used to independently position 16 independent tetrodes shown in (C) using a single piezo actuator (Video 4). (D) Pipette-drive loaded with eight glass micropipettes, and independent motion of each micropipette (E) was also demonstrated (Video 5).
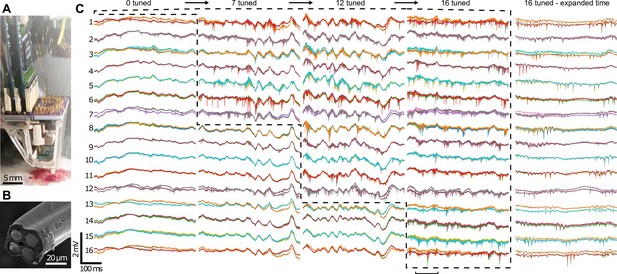
Neural recording with the implantable 16-tetrode-drive in an acute surgical setting.
(A) Photograph of the acute experiment (craniotomy at bottom). (B) Scanning electron microscope image of the cut-face of a tetrode with the four exposed neural recording sites. The tetrodes were independently advanced in 9.5 μm steps sequentially into the brain. (C) Voltage traces from 64 recording channels (4 channels per tetrode) during precise depth tuning, beginning after all tetrodes, were brought near CA1. The position of each tetrode was independently adjusted to optimize its signal; dashed lines show groups of probes that have been optimally tuned. Previously tuned tetrodes did not lose signal after tuning of neighboring electrodes. For each of the 16 tetrodes, the 4 traces from each electrode (different color for each) of a tetrode are overlaid. Rightmost, time expanded segment of traces from period marked by horizontal bar at bottom. The total time to go from 0 to 16 tetrodes tuned here was ~1 hr 10 min.
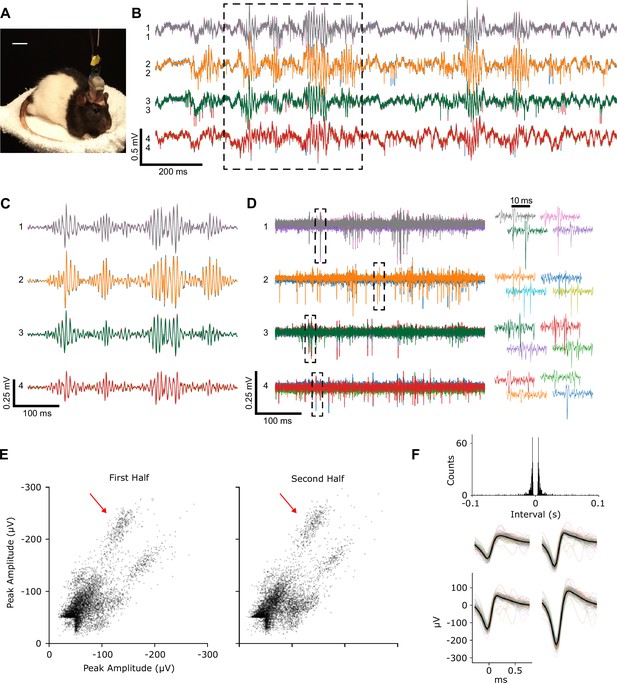
Recordings from chronically implanted head-mounted multi-probe single-actuator tetrode-drive during unrestrained, free behavior of an animal.
(A) Rat implanted with the tetrode-drive. (B) Sample raw voltage traces from four tetrodes obtained ~6 weeks after implantation showing LFP, sharp wave ripples, and spiking activity in the hippocampus. (C) Boxed voltage segment from (B) filtered to isolate sharp-wave ripples (bandpass: 100–300 Hz). (D) Boxed voltage segment from (B) filtered to isolate action potentials (bandpass: 500–8000 Hz). For each of the four tetrodes, the four traces from each electrode (different color for each) of a tetrode are overlaid. Right: spike waveforms from boxes at left. (E) Peak amplitude of each spike recorded on two of the four channels of tetrode 1 during the first and second halves of an ~17 min period between successive tetrode adjustments. (F) Inter-spike interval histogram and waveforms of the spikes on the four channels from one unit (marked by arrow in E) during the full period in E. Average waveform on each channel (thick black line) and individual spike waveforms (thin colored lines) of 100 evenly spaced spikes of that unit.
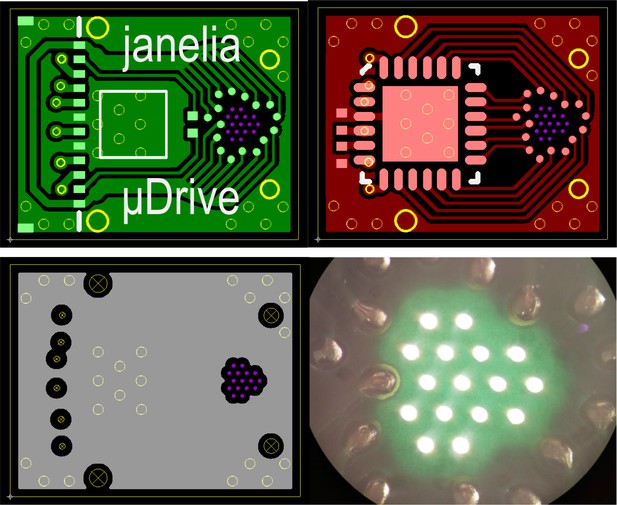
CAD images for the (top left) top, (top right) bottom, and (bottom left) inner layers of the gripper board, as well as (bottom right) an image of light passing through drilled vias and the non-copper areas of a fabricated gripper board.
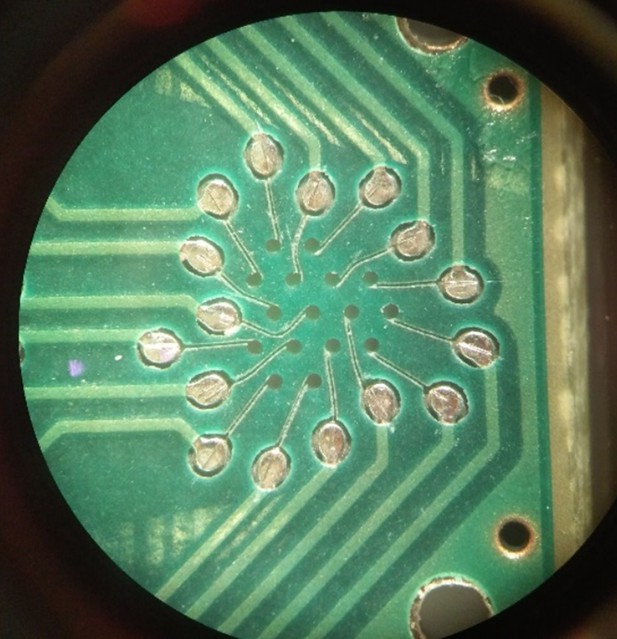
Image depicting the trenches cut into the printed circuit board solder mask for the heater coil leads.
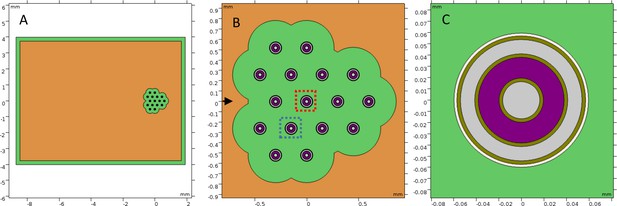
Simulation model geometry.
(A) Gripper board. (B) Gripper region with the plotted central gripper and adjacent gripper boxed in red and blue, respectively, and one-dimensional data cutline axis indicated. (C) Gripper model.
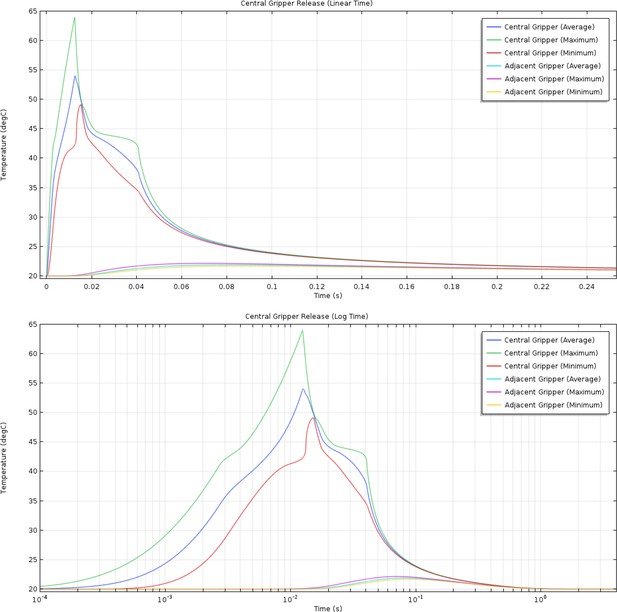
(Top) Linear and (bottom) log time plots of the simulated average, maximum, and minimum temperatures for the phase change material in a single ‘on’ gripper and one adjacent to it.
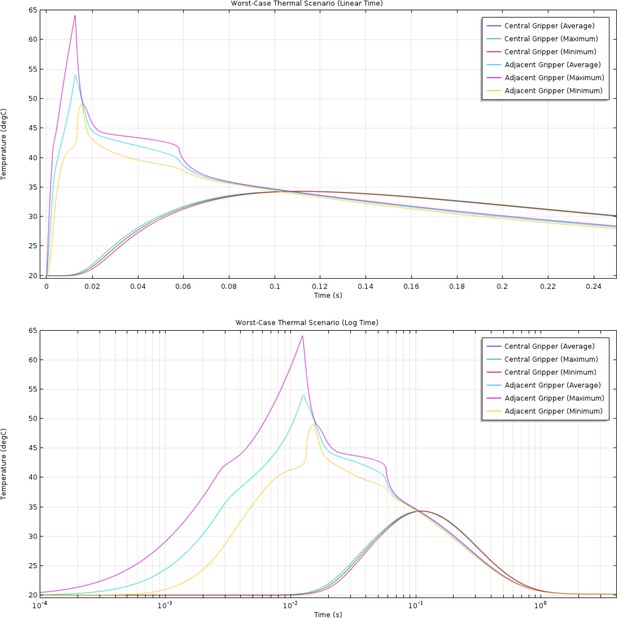
(Top) Linear and (bottom) log time plots of the simulated average, maximum, and minimum temperatures for the phase change material of an ‘on’ gripper and the central ‘off’ gripper in the ‘worst-case’ scenario.
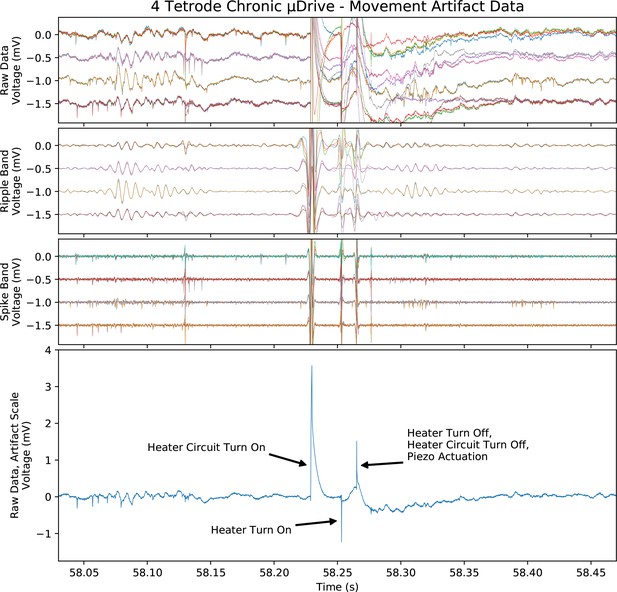
Electrical pick-up artifact from one half cycle of probe adjustment during chronic recording.
Top three plots, artifact at the scale of and with the same filtering as the chronic data in Figure 7. Bottom, unfiltered and full-scale artifact on a single electrode with major sources labeled. Note that the coil voltage was turned off between steps (‘heater circuit turn on/off’).
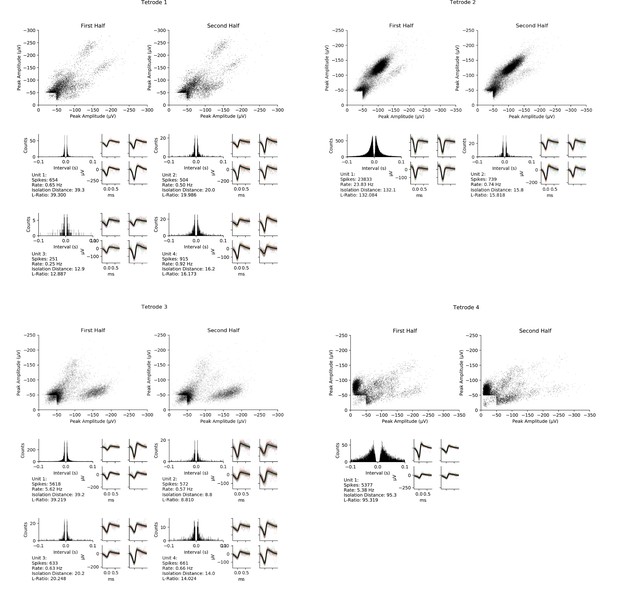
Here and next three pages: All isolated units from the four chronically implanted tetrodes (one page per tetrode) during the ~17 min period between successive tetrode adjustments, recorded from an unrestrained, freely behaving rat.
Note that some of the data from tetrode 1 is also shown in Figure 7E–F. Top, peak amplitude of each spike recorded on two of the four channels of this tetrode during the first and second halves of the period. Note that tetrodes 1 through 3 showed stable activity during the full period while tetrode 4 showed some shifting during the period, limiting the number of isolatable units from that tetrode. Bottom, inter-spike interval histograms and waveforms of the spikes on the four channels for each isolated unit during the full period. Average waveform on each channel (thick black line) and individual spike waveforms (thin colored lines) of 100 evenly spaced spikes of each unit (right), and features of each unit including spike sorting quality metrics isolation distance and L-ratio (lower left).
Videos
Up and down translation of a single tetrode in the robotic inchworm microdrive.
Translation of three tetrodes by the robotic inchworm microdrive in air, demonstrating independent tetrode positioning control with the multi-probe single-actuator concept.
Translation of three tetrodes by the robotic inchworm microdrive demonstrating significant reduction of lateral motion of the tetrodes in agar.
Translation of 16 independent tetrodes by the robotic inchworm microdrive in air with the multi-probe single-actuator (MPSA) concept.
Translation of eight independent glass pipettes by the robotic inchworm microdrive in air with the multi-probe single-actuator concept.
Insertion of four independently controlled tetrodes into the rat brain through a craniotomy.
Gripper board 15-on-1-off thermal simulation.
Center cut-line of 15-on-1-off thermal simulation.
Tables
Material properties of the simulation model.
Material | Thermal conductivity (W/(m·K)) | Heat capacity (J/(kg·K)) | Density (kg/m3) |
---|---|---|---|
FR4 | 0.9 | 1369 | 1900 |
Copper | 400 | 385 | 8700 |
FR4-copper blend | 106 | 1111 | 3685 |
PCM solid | 0.21 | 2480 | 778 |
PCM liquid | 0.21 | 2760 | 778 |
Polyimide | 0.15 | 1100 | 1300 |
Heater wire | 13.1 | 435 | 8110 |
Coil blend | 8.78 | 657 | 8540 |
Epoxy | 0.34 | 1184 | 1000 |