Rapid odor processing by layer 2 subcircuits in lateral entorhinal cortex
Figures
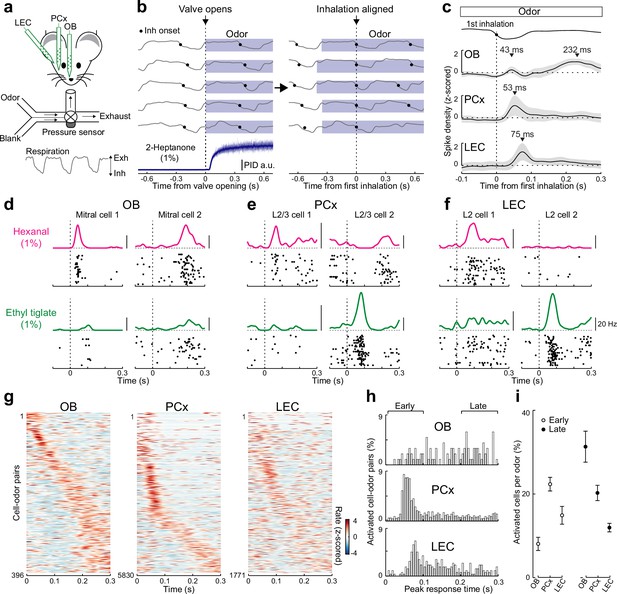
Rapid responses dominate odor-evoked activity in lateral entorhinal cortex (LEC) layer 2 (L2).
(a) Experimental setup for triple recordings of odor-evoked activity in the olfactory bulb (OB), anterior PCx, and LEC of awake head-fixed mice. Downward and upward deflections on respiration trace from pressure sensor indicate inhalation (Inh) and exhalation (Exh), respectively. (b) Left, respiration signals during odor delivery (blue shading) for five trials are plotted above PID measurement of odor (2-Heptanone) at the sampling port. Black circles, inhalation onset. Right, same respiration signals aligned to onset of first inhalation. (c) OB mitral cell odor responses during individual sniffs have both early and late components, while early responses dominate in PCx and LEC. Spike-density plots of single-unit activity averaged across all triple recordings (n = 6 recordings from five mice) from OB (n = 36 cells), PCx (n = 530 cells), and LEC (n = 161 cells) responses to 11 odors. Trials were aligned to the first inhalation during each odor (dashed line). Top trace, representative averaged respiration trace from one experiment. (d–f) Raster plots and spike-density functions of odor-evoked activity for two representative cells from OB, PCx, and LEC in response to hexanal (top) and ethyl tiglate (bottom). (g–i) OB mitral cell responses occur evenly across the entire respiration cycle, while responses of PCx L2/3 cells and LEC L2 cells often occur early following odor inhalation. Plots reflect odor-evoked firing rates for all cell–odor pairs rank ordered by time of peak firing. For cross-validation, peristimulus time histograms are plotted from even trials that are sorted relative to the peaks obtained from odd trials. (g) Plots of odor-evoked firing rates for all cell–odor pairs rank ordered by time of peak firing for OB mitral cells (left), PCx L2/3 cells (middle), and LEC L2 cells (right). (h) Histograms showing time of peak odor responses relative to inhalation onset for significantly activated cell–odor pairs in the OB, PCx, and LEC. (i) Percent of activated cells per odor in the OB, PCx, and LEC during the early (0–100 ms) and late (200–300 ms) phase of odor-evoked activity marked in (h). Error bars represent standard error of the mean (SEM).
-
Figure 1—source data 1
Source data for Figure 1.
- https://cdn.elifesciences.org/articles/75065/elife-75065-fig1-data1-v2.mat
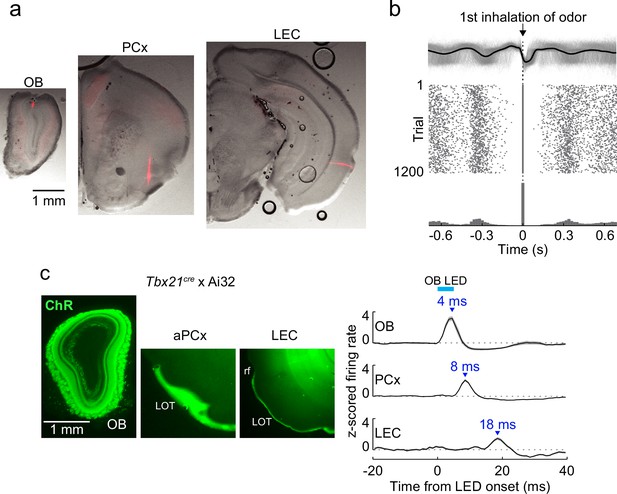
Recording sites, respiration, and propagation of optogenetic activation of mitral cells.
(a) Identification of in vivo recordings sites. Example coronal sections from one mouse showing silicon probe tracks labeled with DiI in olfactory bulb (OB), PCx, and lateral entorhinal cortex (LEC). Only superficial probe contacts are used to record from upper layers in LEC (Methods).
(b) Isolation of first inhalation during odor application. Top, respiration traces (gray) for individual trials from one experiment aligned to the first inhalation during odor delivery. Black trace, average of all trials. Middle, raster plot of inhalation onset times for each trial. Bottom, peristimulus time histogram of inhalation onset times from trials. (c) Optogenetic activation of mitral cells illustrates the minimal time course for propagation of signaling from OB. Left, sections from Tbx21cre × Ai32 mouse showing ChR2/GFP expression in OB mitral cells and lateral olfactory tract (LOT) fibers of PCx and LEC. Right, averaged firing rate of single cells recorded from OB (n = 12 cells, 2 recordings), PCx (n = 171 cells), and LEC (n = 59 cells) in response to 5-ms LED illumination (473 nm) over the OB. Error bars represent standard error of the mean (SEM).
-
Figure 1—figure supplement 1—source data 1
Source data for Figure 1—figure supplement 1.
- https://cdn.elifesciences.org/articles/75065/elife-75065-fig1-figsupp1-data1-v2.mat
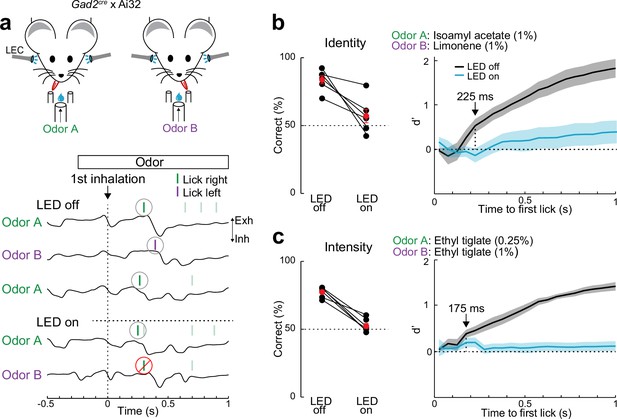
Lateral entorhinal cortex (LEC) is essential for rapid odor-driven behavior.
(a) Top, experimental setup for two-alternative forced-choice (2AFC) odor-driven behavior with bilateral optogenetic silencing of the LEC in head-fixed mice expressing ChR2 in GABAergic neurons. Bottom, representative respiration traces aligned to the first inhalation of odor and superimposed lick responses (colored bars) during odor discrimination. (b) LEC is required for discrimination of odor identity. Left, percent of correct choices for mice trained to discriminate different odors (isoamyl acetate vs. limonene, both at 1.0%) on trials with (LED on) and without (LED off) optogenetic LEC silencing (n = 6 mice). Red circles, mean ± standard error of the mean (SEM). Right: d′ of cumulative responses over time. Under control conditions (LED off), d′ becomes significantly different 225 ms following odor inhalation. (c) LEC is required for discrimination of odor intensity. Left, percent of correct choices for mice trained to discriminate different concentrations of the same odor (0.25% vs. 1.0% ethyl tiglate) for trials with and without LEC silencing (n = 6 mice). Red circles, mean ± SEM. Right: d′ of cumulative responses over time. Under control conditions (LED off), d′ becomes significantly different 175 ms following odor inhalation. Error bars represent SEM.
-
Figure 2—source data 1
Source data for Figure 2.
- https://cdn.elifesciences.org/articles/75065/elife-75065-fig2-data1-v2.mat
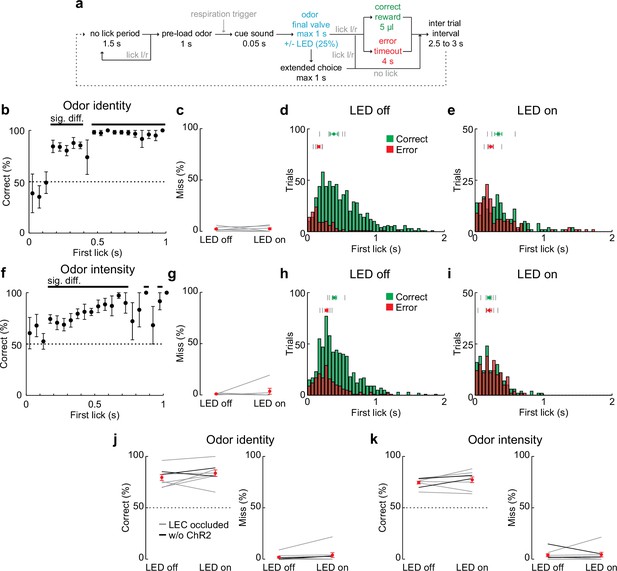
Behavioral trial structure and performance in 2AFC discrimination tasks.
(a) Schematic of behavior trial structure.
(b) Performance in odor identity discrimination for LED off trials plotted relative to the choice time (first lick after odor inhalation). Black bar, significantly different from chance. In this and subsequent panels, time zero responds to the time of first odor inhalation. (c–e) Miss rate and lick timing when lateral entorhinal cortex (LEC) is silenced during two-alternative forced-choice (2AFC) odor identity discrimination. (c) Percent of miss trials in the 2AFC with and without optogenetic silencing of the LEC during all experiments (n = 6 mice). Gray lines, individual experiments. Red circle, mean ± standard error of the mean (SEM). (d) Histograms of time to first lick for correct (green) and error (red) trials with LED off. Tick marks indicate average response times for individual mice (top, Correct; bottom, Error). Green circle, timing of correct responses averaged across mice (± SEM). Red circle, error responses (± SEM). (e) As in (d) for LED on trials. (f) Performance in odor intensity discrimination for LED off trials plotted relative to the choice time (first lick after odor inhalation). Black bar indicates significantly different from chance. (g–i) Miss rate and lick timing when LEC is silenced during odor intensity discrimination (n = 6 mice). (e–f) As for (c–e). (j) Control experiments for LEC silencing during odor identity discrimination. Left, percent of correct choices during sham optogenetic silencing of the LEC (n = 8 mice). Gray lines, same mice from experiments above but with the LEC masked from LED illumination (LEC occluded). Black lines, Gad2cre mice trained in the task but uninjected with adeno-associated virus (AAV)-ChR2. Right, percent of miss trials during sham silencing. Red symbols, average ± SEM. (k) Control experiments for LEC silencing during odor intensity discrimination (n = 8 mice). As for (g). Error bars represent SEM.
-
Figure 2—figure supplement 1—source data 1
Source data for Figure 2—figure supplement 1.
- https://cdn.elifesciences.org/articles/75065/elife-75065-fig2-figsupp1-data1-v2.mat
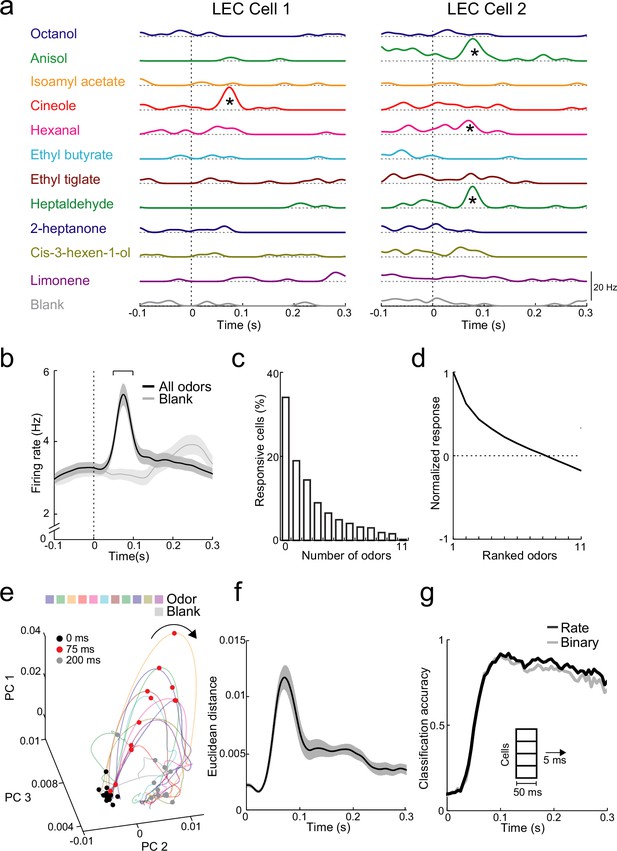
Rapid increases in firing rate encode odor identity in lateral entorhinal cortex (LEC) layer 2 (L2).
(a) Spike-density functions of inhalation-aligned odor-evoked activity (11 odors and blank) for 2 representative LEC L2 cells. Asterisks, significant odor-evoked increases in firing rate. (b) Averaged spike-density function of activity in LEC L2 (n = 576 cells) shows early increase in firing rate for odor-evoked responses (black line) vs. baseline respiration-coupled activity (blank, gray line). Bracket, 50-ms window used to measure early odor-evoked firing. (c) Percent of cells responsive from 0 to 11 odors. (d) Normalized and ranked odor-evoked firing rate change relative to blank in LEC L2. (e–g) Principal component analysis reveals that LEC cell ensembles can rapidly distinguish odor identity. (e) Trajectories of the first three principal components of odor-evoked activity in LEC L2 over time following odor inhalation (each odor indicated by different colored line). Circles represent time points relative to odor inhalation: 0 ms (black), 75 ms (red), and 200 ms (gray). (f) Euclidean distance of the first three principal components (69% explained variance) between 11 odors (binned over 1-ms time window) shows discriminability peak within 100 ms of odor inhalation. (g) A linear classifier can rapidly discriminate odor identity from early odor-evoked changes in firing rate or binary categorization of activity. Classification accuracy is plotted using mean firing rate (black) or binary measure of cell activation (gray) using a 50-ms sliding window with 5-ms steps. Error bars represent standard error of the mean (SEM).
-
Figure 3—source data 1
Source data for Figure 3.
- https://cdn.elifesciences.org/articles/75065/elife-75065-fig3-data1-v2.mat
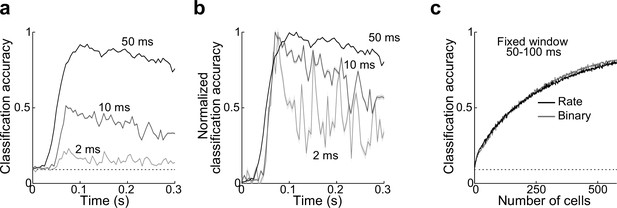
Classifier accuracy.
(a, b) Classifier accuracy is highest during early periods of the odor response.
(a) Reducing bin size from 50 to 10 and 2 ms for measurements of classifier accuracy of odor identity makes accuracy course more transient. Dotted line, chance. (b) Same as (a) but baselined to chance and scaled to peak accuracy. (c) Classification accuracy as a function of ensemble size is identical using firing rate or binary measure of activity in a fixed window at 50–100 ms. Error bars represent standard error of the mean (SEM).
-
Figure 3—figure supplement 1—source data 1
Source data for Figure 3—figure supplement 1.
- https://cdn.elifesciences.org/articles/75065/elife-75065-fig3-figsupp1-data1-v2.mat
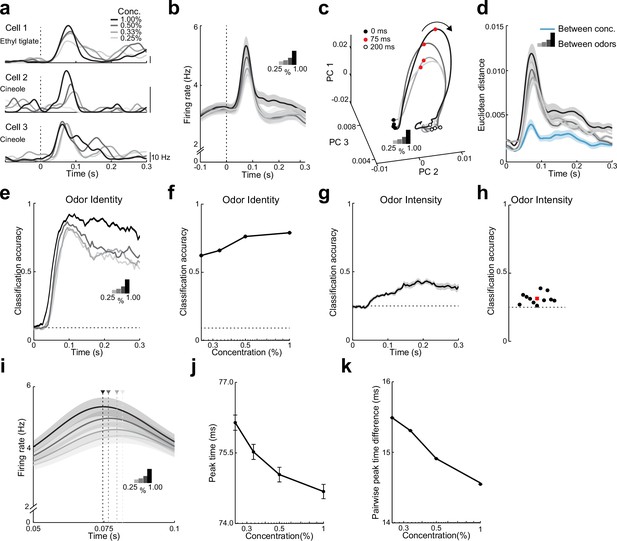
Both rate and timing of odor-evoked activity encode odor intensity in lateral entorhinal cortex (LEC) layer 2 (L2).
(a) Spike-density functions of odor-evoked activity for three representative LEC L2 cell–odor pairs at four concentrations indicated by line shading (0.25%, 0.33%, 0.50%, and 1.00%). (b) Averaged spike-density function of odor-evoked activity aligned to the first inhalation across four concentrations of the same odors for the LEC L2 cell population (n = 576 cells, 11 odors at 0.25%, 0.33%, 0.50%, and 1.00%). (c, d) Principal component analysis of the cell population for responses to four odor concentrations indicates that firing rate can be used to discriminate odor intensity, but discriminability is poorer than that for odor identity. (c) Trajectories of the first three principal components of odor-evoked activity over time following odor inhalation (each odor concentration indicated by a different line shading). Circles represent time points relative to odor inhalation: 0 ms (filled black), 75 ms (red), and 200 ms (open black). (d) Euclidean distance of the first three principal components of odor-evoked activity in the LEC between responses of the four concentrations (blue) shows a peak in discriminability within 100 ms of odor inhalation. The discriminability between responses of the same cells to 11 odors for the four different intensities (gray to black) is much higher. Responses are binned with a 1-ms time window. (e–h) A linear classifier shows that odor identity discrimination somewhat improves as odor concentration increases and that odor intensity can be weakly discerned from firing rate changes. (e) Classification accuracy for odor identity at the four concentrations indicated by the shaded lines using a 50-ms sliding window with 5-ms steps. (f) Classification accuracy of odor identity at different concentrations based on average firing rate in a fixed window 50–100 ms following odor inhalation. (g) Classification accuracy of odor intensity for each odor based on average firing rate using a sliding window. (h) Classification accuracy of odor intensity for each odor based on average firing rate in a fixed window 50–100 ms following odor inhalation. (i–k) Spike timing effectively encodes odor intensity. (i) Blow-up of data from (b) shows shift in timing of peak responses with odor concentration. Arrowheads mark peak times at the four concentrations. (j) Peak time of odor-evoked activity averaged across all cell–odor pairs shifts with changes in odor concentration. (k) Mean pairwise peak time difference of odor-evoked activity for cell–odor pairs indicates that spike firing becomes more synchronized as odor concentration increases. Error bars represent standard error of the mean (SEM).
-
Figure 4—source data 1
Source data for Figure 4.
- https://cdn.elifesciences.org/articles/75065/elife-75065-fig4-data1-v2.mat
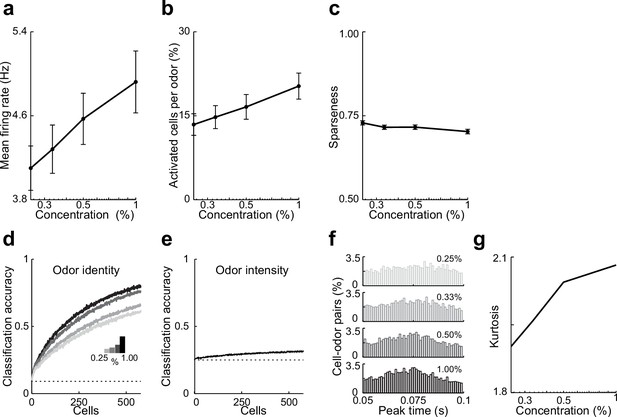
Summary data and classifier accuracy describing effects odor concentration on responses in lateral entorhinal cortex (LEC) layer 2 (L2).
(a–c) Summary data describing effects of odor concentration on responses in lateral entorhinal cortex (LEC) layer 2 (L2). (a) Mean firing rate at each concentration for all cell–odor pairs. (b) Percent of activated cells per odor (rate during 50–100-ms window postinhalation) at different intensities. (c) Lifetime sparseness of odor identity for the four different concentrations. (d, e) Odor identity vs. odor intensity rate coding as a function of ensemble size. (d) Odor identity classification accuracy using mean firing rate at different intensities (fixed window 50–100-ms postinhalation). (e) Odor intensity classification accuracy using same measure. (f–g) Activity becomes more synchronized as odor concentration increases. (f) Peak time histograms of odor-evoked activity at different intensities for all cell–odor pairs. (g) Kurtosis of the peak time distributions shown in (C1). Error bars represent standard error of the mean (SEM).
-
Figure 4—figure supplement 1—source data 1
Source data for Figure 4—figure supplement 1.
- https://cdn.elifesciences.org/articles/75065/elife-75065-fig4-figsupp1-data1-v2.mat
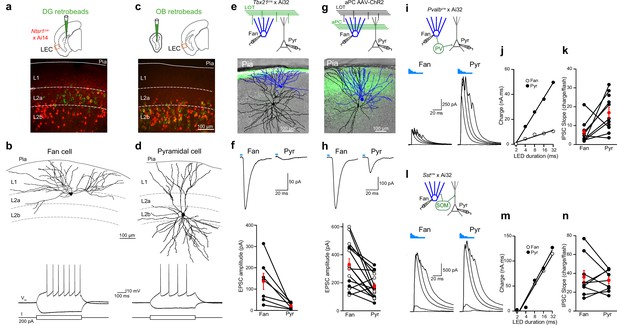
Layer 2 (L2) fan and pyramidal cells differ in olfactory input and inhibitory circuit connectivity.
(a) Top, schematic of retrobead injection into the DG of Ntsr1(209)cre mice expressing TdTomato. Bottom, image of lateral entorhinal cortex (LEC) region outlined in schematic showing DG-projecting cells (green) located in L2a and Ntsr1-positive cells (red) concentrated in L2b. (b) Targeted brain slice recording from a DG-projecting fan cell. Top, reconstruction of fan cell dendritic arbor. Bottom, membrane potential response to current steps. (c) Top: schematic of retrobead injection into the olfactory bulb (OB) of Ntsr1(209)cre mice expressing TdTomato. Bottom, image of LEC region showing OB-projecting cells (green) and Ntsr1-positive cells (red) colocalize in L2b. (d) Targeted brain slice recording from an OB-projecting pyramidal cell. Top, reconstruction of pyramidal cell dendritic arbor. Bottom, membrane potential response to current steps. (e, f) Fan cells receive stronger direct OB input than pyramidal cells. (e) Top, recording schematic for paired recordings of fan and pyramidal cells in LEC slices expressing ChR2 in lateral olfactory tract (LOT) fibers. Bottom, reconstruction of simultaneously recorded fan cell (blue) and pyramidal cell (black) overlaid on image of LEC slice showing ChR2 expression in LOT (green). (f) Top, LED-evoked excitatory postsynaptic currents (EPSCs) in fan and pyramidal cells from e. Blue bar, LED flash. Bottom, summary of EPSC amplitudes elicited by stimulation of OB input. Black circles, individual cells. Red circles, mean ± standard error of the mean (SEM). (g, h) Fan cells receive stronger direct PCx input than pyramidal cells. (g) Top, recording schematic for paired recordings in LEC slices expressing ChR2 in PCx. Bottom, reconstruction of simultaneously recorded fan cell (blue) and pyramidal cell (black) overlaid on image of LEC slice showing ChR2 expression in PCx fibers (green). (h) Top, LED-evoked EPSCs in fan and pyramidal cells from (g). Blue bar, LED. Bottom, summary of EPSC amplitudes elicited by stimulation of PCx input. Black circles, cells in control artificial cerebrospinal fluid (aCSF). Open circles, cells recorded in tetrodotoxin (1 µM) and 4-aminopyridine (100 µM). Red circles, mean ± SEM. (i–k) Fan cells receive less parvalbumin (PV) cell-mediated inhibition than pyramidal cells. (i) Top, recording schematic. Bottom, LED-evoked inhibitory postsynaptic currents (IPSCs) elicited in simultaneously recorded fan and pyramidal cells. Traces indicate responses to five LED pulses of increasing duration (blue bars). (j) Inhibitory charge for fan cell (open circles) and pyramidal cell (filled circles) shown in (i). Lines fit to points are used to measure slope of input–output relationship. (k) Summary of IPSC slopes for fan and pyramidal cells in response to activation of PV cells. Black circles, individual cells. Red circles, mean ± SEM. (l–n) Fan cells and pyramidal cells receive similar somatostatin (SOM) cell-mediated inhibition. (l) Top, recording schematic. Bottom, LED-evoked IPSCs elicited in simultaneously recorded fan and pyramidal cells. Traces indicate responses to five LED pulses of increasing duration (blue bars). (m) Inhibitory charge for fan cell (open circles) and pyramidal cell (filled circles) shown in (l). (n) Summary of IPSC slopes for fan and pyramidal cells in response to activation of SOM cells. Black circles, individual cells. Red circles, mean ± SEM.
-
Figure 5—source data 1
Source data for Figure 5.
- https://cdn.elifesciences.org/articles/75065/elife-75065-fig5-data1-v2.xls
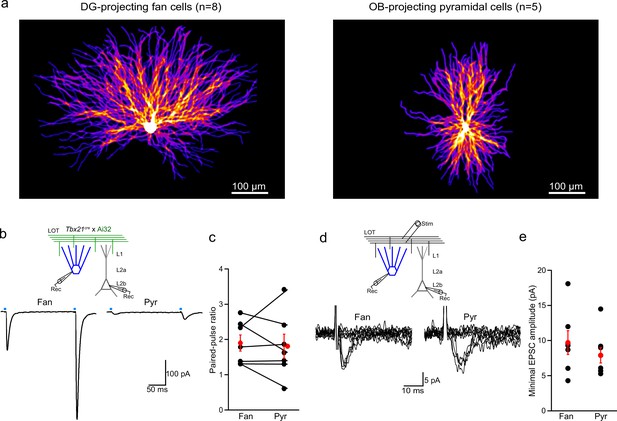
Dendritic arbors, paired-pulse ratio, and minimal stimulation for fan and pyramidal cells in lateral entorhinal cortex (LEC).
(a) The dendritic arbors of lateral entorhinal cortex (LEC) fan cells take up more cortical space than pyramidal cells.Left, heat map of reconstructed DG-projecting LEC layer 2 (L2) fan cells (n = 8 cells). Image shows average of all cells superimposed. Brighter colors indicate more overlap of dendrites across cells. Right, same for LEC L2 pyramidal cells (n = 5 cells). (b, c) Paired-pulse ratios of lateral olfactory tract (LOT)-evoked excitatory postsynaptic currents (EPSCs) onto fan and pyramidal cells suggest synapses of both cell types have similar release probabilities. (b) Top, recording schematic for paired whole-cell recordings of LEC L2 fan and pyramidal cells and optogenetic stimulation (4 ms) of the LOT input. Bottom, representative EPSCs elicited by paired-pulse stimulation (interstimulus interval, 200 ms). (c) Paired-pulse ratio of responses from paired recordings. Black circles and lines, individual cell pairs. Red circles, mean ± standard error of the mean (SEM). (d, e) Minimal LOT stimulation indicates that fan and pyramidal cells have similar single fiber responses. (d) Top, recording schematic showing LOT minimal electrical stimulation. Bottom, overlay of superimposed trials (n = 10) showing failures and successes recorded in a fan (left) and pyramidal cell (right) using threshold stimulation. (e) Summary of minimal EPSC amplitude (average of success) in fan and pyramidal cells (n = 7 and 6, respectively). Black circles, individual recordings. Red circles, mean ± SEM. Error bars represent SEM.
-
Figure 5—figure supplement 1—source data 1
Source data for Figure 5—figure supplement 1.
- https://cdn.elifesciences.org/articles/75065/elife-75065-fig5-figsupp1-data1-v2.xls
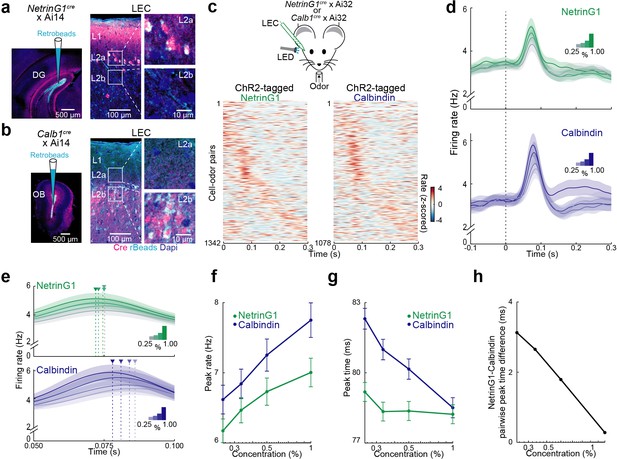
Temporal coding of odor intensity by fan and pyramidal cells.
(a, b) Transgenic mouse lines allow targeting of layer 2 (L2) fan and pyramidal cells in lateral entorhinal cortex (LEC). (a) NetrinG1-cre expression in L2a fan cells. Left, site of DG retrobead (blue) injection in NetrinG1cre × Ai14 mouse. Right, LEC section indicates cre restricted to DG-projecting cells in L2a. (b) Calb1-cre expression in L2b pyramidal cells. Left, site of olfactory bulb (OB) retrobead (blue) injection in Calb1cre × Ai14 mouse. Right, LEC section indicates cre within OB-projecting cells in L2b. (c) Odors evoke rapid responses in optogenetically tagged fan and pyramidal cells. Top, experimental setup. Bottom, plots of odor-evoked firing rates for all cell–odor pairs rank ordered by time of peak firing for ChR2-tagged NetrinG1 (left) and calbindin (right) neurons (n = 122 and 98 cells, respectively). Peristimulus time histograms using only even trials are sorted relative to the peaks obtained from odd trials. (d) Odor concentration-dependent timing of pyramidal cell activity. Average time course of activity evoked by different odor concentrations (0.25%, 0.33%, 0.50%, and 1.00%) for tagged NetrinG1/fan (top) and calbindin/pyramidal (bottom) cells. (e) Traces in (d) shown on a faster timescale. Arrowheads mark times of peak firing for the different intensities. (f) Peak rates of odor-evoked activity at different intensities for NetrinG1/fan and calbindin/pyramidal cell–odor pairs. (g) Peak times of odor-evoked activity at different intensities reveal strong dependence on odor concentration for calbindin/pyramidal but not NetrinG1/fan cells. (h) Synchrony between fan and pyramidal cell firing depends on odor concentration. Pairwise peak time differences of NetrinG1/fan and calbindin/pyramidal cell–odor pairs decrease as odor concentration increases. Error bars represent standard error of the mean (SEM).
-
Figure 6—source data 1
Source data for Figure 6.
- https://cdn.elifesciences.org/articles/75065/elife-75065-fig6-data1-v2.mat
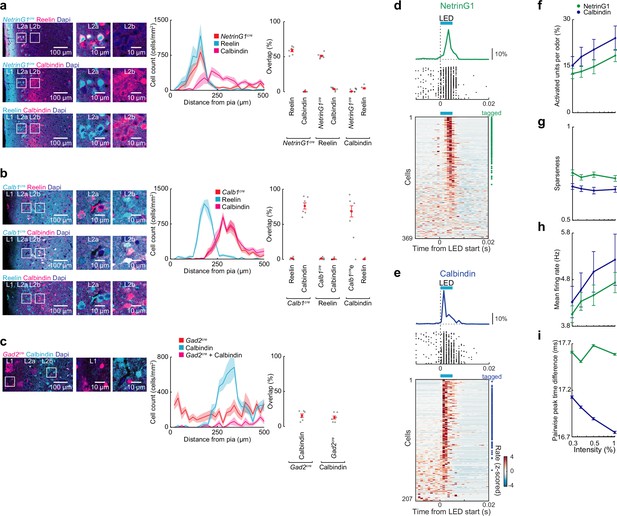
Expression specificity for NetrinG1-cre and Calbindin-cre mice and optotagging of princpal neurons in lateral entorhinal cortex (LEC).
(a) NetrinG1cre labeling of fan cells. Left, coronal sections of lateral entorhinal cortex (LEC) from mice expressing tdTomato in cre+ neurons immunostained for Reelin and Calbindin. Center, locations of labeled neurons (n = 6 slices, three mice). Right, percent overlap between labeled neurons in layer 2 (L2) (n = 6 slices from three mice). (b) Calb1cre labeling of pyramidal cells. Left, coronal sections of LEC from mice expressing tdTomato in cre+ neurons immunostained for Reelin and Calbindin. Center, locations of labeled neurons (n = 6 slices, three mice). Right, percent overlap between labeled neurons in L2 (n = 6 slices, three mice). (c) Only a small fraction of calbindin-expressing cells in LEC L2 are GABAergic. Left, representative images of LEC L2 from coronal sections of mice expressing tdTomato in Gad2-positive neurons immunostained for calbindin. Center, distance of labeled neurons from the pia (n = 6 slices from three mice). Right, overlap between labeled neurons (n = 6 slices, three mice). (d, e) Optogenetic tagging of ChR2-expressing neurons in NetrinG1 cre and Calb1cre mice. (d) Single units identified as fan cells in NetrinG1cre mice. Top, spike raster and PSTH from a ChR2-expressing cell responding to LED illumination (5 ms, 473 nm). Bottom, plot of all L2 cells recorded during phototagging experiments rank ordered by LED-evoked firing rate. Symbols at right indicate cells meeting tagging criteria (see Methods). (e) Identified pyramidal cells in Calb1cre mice as in (d). The longer latency for activation of NetrinG1 cells may reflect lower levels of ChR2 expression compared to pyramidal cells. (f–i) Summary of NetrinG1cre/fan and Calb1cre/pyramidal cell responses (50- to 100-ms postinhalation). (f) Percent of activated NetrinG1 (green) and calbindin (purple) cells. (g) Lifetime sparseness of odor-evoked activity. (h) Mean firing rate of odor-evoked activity all cell–odor pairs. (i) Mean pairwise peak time difference at different intensities. Error bars represent standard error of the mean (SEM).
-
Figure 6—figure supplement 1—source data 1
Source data for Figure 6—figure supplement 1.
- https://cdn.elifesciences.org/articles/75065/elife-75065-fig6-figsupp1-data1-v2.mat
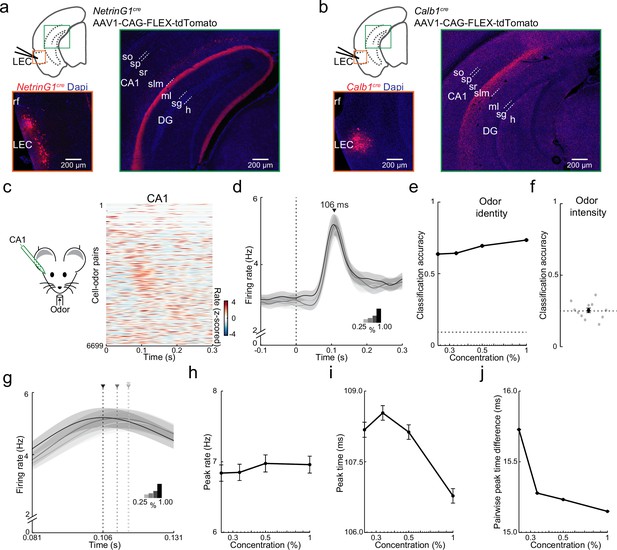
Purely temporal coding of odor intensity in CA1.
(a, b) Fan and pyramidal cells target distinct hippocampal regions. (a) Schematic of fan cell anterograde viral tracing strategy in NetrinG1cre mice and images showing AAV-FLEX-tdTomato expression (red) at lateral entorhinal cortex (LEC) injection site (left) and projections concentrated in DG (right). (b) Schematic of pyramidal cell tracing strategy in Calb1cre mice and images showing AAV-FLEX-tdTomato expression (red) at LEC injection site (left) and projections concentrated in CA1 stratum (s) lacunosum moleculare (slm, right). Hippocampal regions: so, s. oriens; sp, s. pyramidale; sr, s. radiatum; ml, DG molecular layer; sg, DG s. granulosum; h, DG hilus. (c–f) Rapid odor-evoked changes in firing rate encode odor identity but not intensity in CA1 pyramidal cells. (c) CA1 pyramidal cells respond rapidly to odors. Left, experimental setup. Right, odor-evoked firing rates for all cell–odor pairs rank ordered by time of peak firing in CA1 (n = 609 cells, 11 odors). Peristimulus time histograms using only even trials are sorted relative to the peaks obtained from odd trials. (d) Average activity at four odor concentrations for CA1 cells aligned to inhalation onset show that firing rate is independent of odor concentration. (e) Firing rate encodes odor identity in CA1. Plot shows classification accuracy of odor identity at the different intensities (0.25%, 0.33%, 0.50%, and 1.00%) based on the average firing rate in a fixed time window (81–131 ms after inhalation onset). Chance, dotted line. (f) Firing rate does not encode odor intensity. Classification accuracy of odor intensity for 11 odors based on average firing rate in the same fixed time is no better than chance (dotted line). (g–j) Odor intensity encoded by spike timing changes in CA1. (g) Data from (d) on a faster timescale show odor concentration-dependent shift in timing of peak response. Arrowheads indicate peak times for the concentrations indicated by different shading. (h) Peak firing rate of odor-evoked activity for all cell–odor pairs is independent of concentration. (i) Peak time of odor-evoked activity for cell–odor pairs is concentration dependent. (j) Pairwise peak time differences between neurons indicates that odor-evoked firing in CA1 becomes more synchronous as odor concentration is increased. Error bars represent standard error of the mean (SEM).
-
Figure 7—source data 1
Source data for Figure 7.
- https://cdn.elifesciences.org/articles/75065/elife-75065-fig7-data1-v2.mat
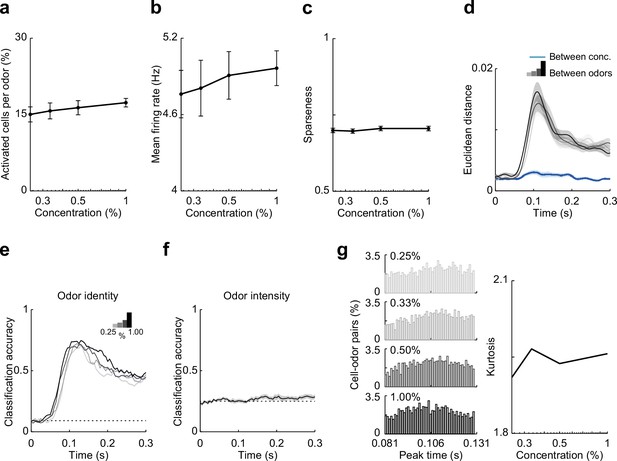
Summary data and classifier accuracy describing effects odor concentration on responses in hippocampal CA1.
(a–d) Summary of CA1 responses to odor concentrations (81- to 132-ms postinhalation). (a) Percent of activated cells per odor. (b) Mean firing rate of odor-evoked activity for all cell–odor pairs. (c) Lifetime sparseness of odor-evoked activity. (d) Euclidean distance of the first three principal components (74% explained variance) of odor-evoked activity between 11 odors (gray to black) at the different concentrations (binned with 1-ms time window). Blue line indicates Euclidean distance between intensities for any given odor. (e, f) Linear classifier performs well using CA1 firing rate to decode odor identity but performs poorly decoding odor intensity. (e) Classification accuracy for odor identity at different intensities (0.25%, 0.33%, 0.50%, and 1.00%) based on average firing rate (50-ms sliding window with 5-ms steps). (f) Classification accuracy of odor intensity for all 11 odors based on average firing rate. (B3) CA1 activity becomes more synchronized as odor concentration increases. Left, peak time histograms of odor-evoked activity at different intensities for all cell–odor pairs. Right, kurtosis of the peak time distributions shown at left. Error bars represent standard error of the mean (SEM).
-
Figure 7—figure supplement 1—source data 1
Source data for Figure 7—figure supplement 1.
- https://cdn.elifesciences.org/articles/75065/elife-75065-fig7-figsupp1-data1-v2.mat