Understanding implicit sensorimotor adaptation as a process of proprioceptive re-alignment
Figures
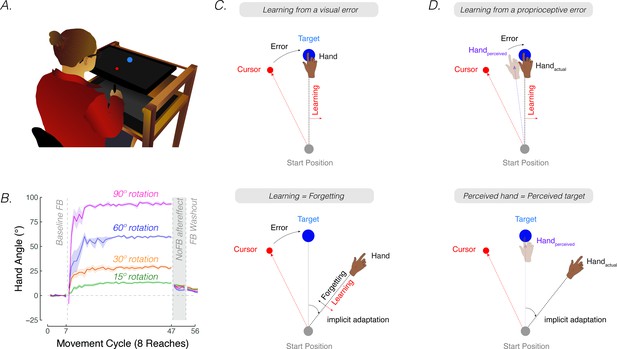
Contrasting visuo-centric and proprioceptive-centric views of implicit motor adaptation.
(A) Experiment setup. (B) Mean time courses of hand angle for 15° (green), 30° (yellow), 60° (purple), and 90° (pink) rotation conditions (adapted from Figure 7A in Bond and Taylor, 2015). Hand angle is presented relative to the target (0°) during veridical feedback, rotation, and no-feedback trials (grey background). Hand angle is similar during the no-feedback trials for all four perturbation sizes, indicating equivalent implicit adaptation. Shaded region denotes SEM. Note that (Bond and Taylor, 2015) used eight target locations, and thus, had eight reaches per cycle. (C) The cursor feedback (red dot) follows a trajectory that is rotated relative to the line connecting the start position and target (blue dot). With visual clamped feedback, the angular trajectory of the visual cursor is not spatially tied to the angular trajectory of the participant’s (hidden) hand but follows a trajectory that is at an invariant angle relative to the target. Despite awareness of the manipulation and instructions to always reach directly to the target, participants show a gradual change in heading direction that eventually reaches an asymptote. According to visuo-centric models, the goal of implicit adaptation is to minimize a visual error (i.e. error = visual cursor – target; upper panel), with the extent of implicit adaptation being the point of equilibrium between learning and forgetting (lower panel). (D) According to the proprioceptive re-alignment model (PReMo), the goal of implicit adaptation is to minimize a proprioceptive error (i.e. error = perceived hand position – target, upper panel). The perceived (shaded) hand position is influenced by the actual, the expected (based on efference copy), and seen (i.e. visual cursor) hand location. The extent of implicit adaptation corresponds to the point in which the perceived hand position is at the target (lower panel).
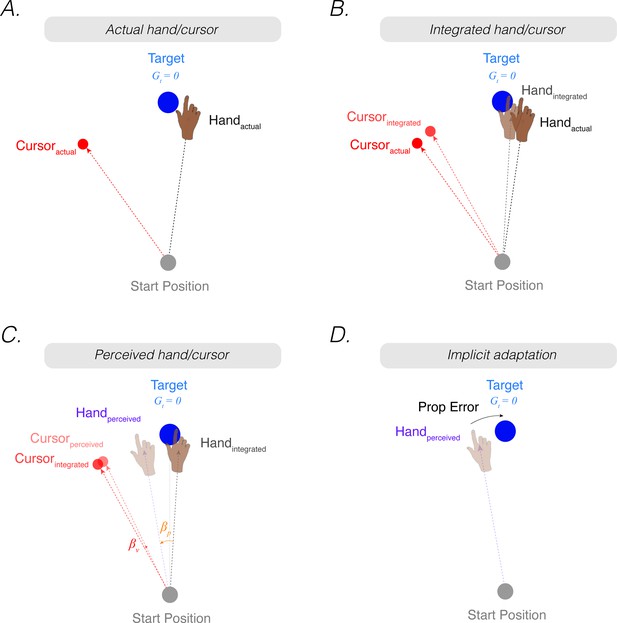
The proprioceptive re-alignment model (PReMo).
(A) When the feedback is rotated, the position of the feedback cursor (red dot) is rotated counterclockwise with respect to the location of the unseen hand (deviation depicted here arises from motor noise). (B) Due to intramodal integration of sensory input and sensory expectations from the motor command, the integrated hand would lie between the visual target and the actual position of the hand, and the integrated cursor would lie between the visual target and the actual cursor. (C) Due to crossmodal sensory recalibration, the integrated hand shifts toward the integrated cursor (proprioceptive shift, ) and the integrated cursor shifts toward the integrated hand (visual shift, ), forming the perceived hand and perceived cursor locations. (D) The proprioceptive error (mismatch between the perceived hand position and the target, ) drives implicit adaptation in the clockwise direction, opposite to the imposed counterclockwise rotation.
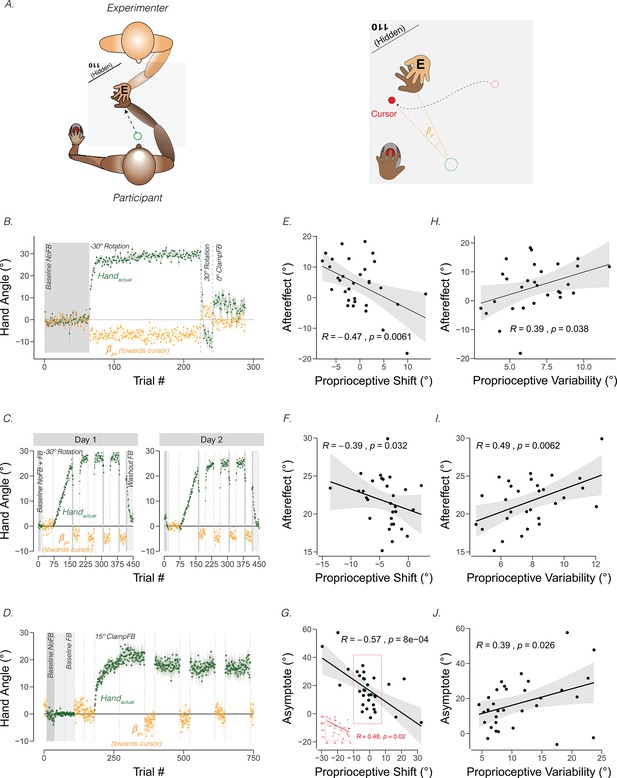
Proprioceptive shift and variability correlate with the upper bound of adaptation.
(A) Experimental setup for proprioceptive probe trials in Tsay et al., 2021a. The experimenter sat opposite the participant and moved the participant’s hand from the start position to a location specified in the corner of the monitor (e.g. 110°) that was only visible to the experimenter. After the participant’s hand was passively moved to the probe location, a cursor appeared at a random position on the screen (right panel). The participant used their left hand to move the cursor to the perceived hand position. A similar method was used in Ruttle et al., 2021, but instead of the experimenter, a robot manipulandum was programmed to passively move the participant’s arm. (B) Abrupt visuomotor rotation design from Ruttle et al., 2021 (Exp 1; adapted from Figure 2a in Ruttle et al., 2021). After a baseline veridical feedback block, participants were exposed to a –30° rotated cursor feedback block, a 30° rotated feedback block, and a 0° clamped feedback block. Vertical dotted lines indicate block breaks. Green dots denote hand angle. Orange dots denote proprioceptive probe trials. Shaded error bars denote SEM. (C) Gradual visuomotor rotation design from Tsay et al., 2021b (Exp 1; adapted from Figure 3a in Tsay et al., 2021c). After baseline trials without feedback (dark grey) and veridical feedback (light grey), participants were exposed to a perturbation that gradually increased to –30° and then held constant. There were periodic proprioceptive probe blocks (orange dots) and no feedback motor aftereffect blocks (dark grey). (D) Clamped rotation design from Tsay et al., 2021b (Exp 2; adapted from Figure 5a in Tsay et al., 2021c). After a period of baseline trials, participants were exposed to clamped visual feedback that moves 15° away from the target. (E – G) Correlation between proprioceptive shift and the extent of implicit adaptation. Note that the correlations are negative because a leftward shift in proprioception (toward the cursor) will push adaptation further to the right (away from the target and in the opposite direction of the cursor). Black dots represent individual participants. (H – J) Correlation between variability on the proprioceptive probe trials during baseline and the extent of implicit adaptation in the three experiments depicted in (B-D).
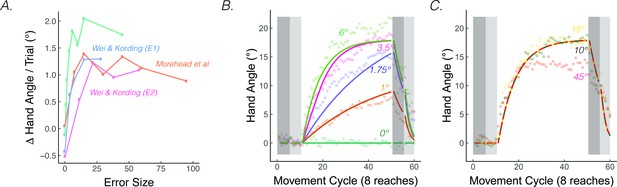
The rate of implicit adaptation saturate.
(A) Early adaptation rates saturate for studies using different methodologies. (B) Data from Kim et al., 2018. Different groups of participants made reaching movements with clamped visual feedback of varying sizes (0° - 45°; groups were divided into two panels for visualization purposes). Groups with smaller clamps (less than 6°) exhibited early adaptation rates that scaled with the size of the clamped feedback, but groups with larger clamped feedback (6° and above) showed a saturated early adaptation rate. Lines denote model fits of the proprioceptive re-alignment model ().
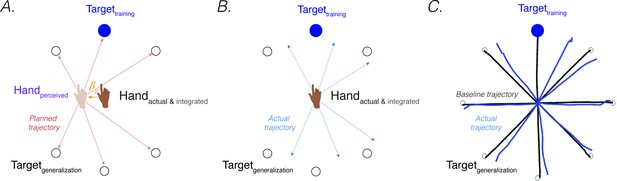
Proprioceptive shift at the start position explain patterns of generalization following a visuomotor rotation.
(A) Planned movement trajectories are formed by participants planning to make a movement initiated at their perceived hand position to the target location (red solid lines). The perceived hand position is assumed to be biased by a proprioceptive shift (). (B) The predicted generalization pattern of the proprioceptive re-alignment model (dashed blue lines; the planned trajectory is initiated from the actual/integrated hand position at the start position). (C) Pattern of generalization assessed during no-feedback generalization trials following training with a 45° CCW rotation in one direction (upward). Black lines are baseline trajectories; blue lines are generalization trajectories. Note match of observed generalization pattern with predicted pattern shown in B, with some trajectories deviated in the clockwise direction, others in the counterclockwise direction, and no change in heading for the reaches along the horizontal meridian. Figure adapted from Figure 5a in Taylor et al., 2013.
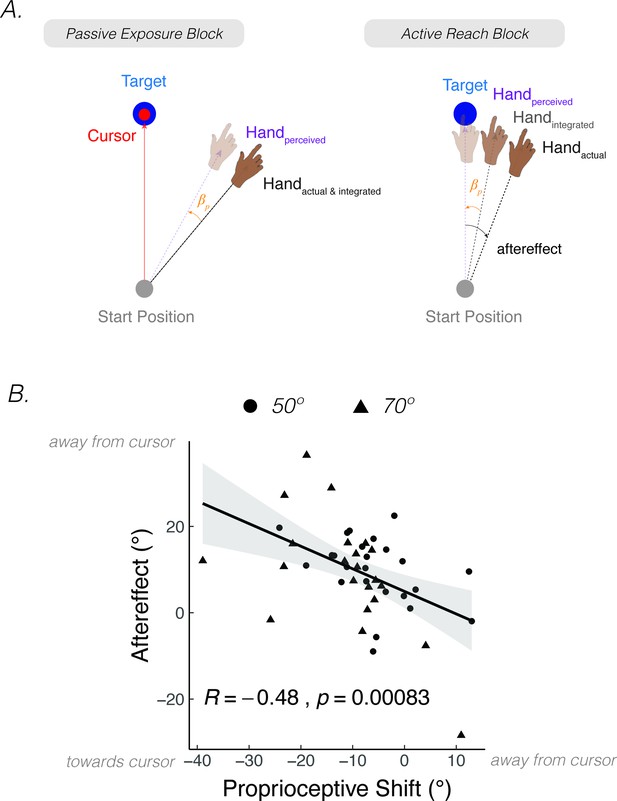
After effects are elicited after passive exposure to a visuo-proprioceptive discrepancy.
(A) In Salomonczyk et al., 2013, the hand was passively moved by a robot during the exposure block, with a gradual perturbation introduced that eventually reached 50° or 70° from the target (between-participant design). Simultaneous online visual feedback was provided, with the cursor moving directly to the target position. An aftereffect was measured during the active reach block in which the participant was instructed to reach directly to the target without visual feedback. (B) Magnitude of shift in perceived hand position assessed during the passive exposure block was correlated with the motor aftereffect (Dots = 50° group; Triangles = 70° group). The more negative values on x-axis indicate a larger proprioceptive shift towards the target. The larger values on the y-axis indicate a larger motor aftereffect. Figure adapted from Figure 5 of Salomonczyk et al., 2013.
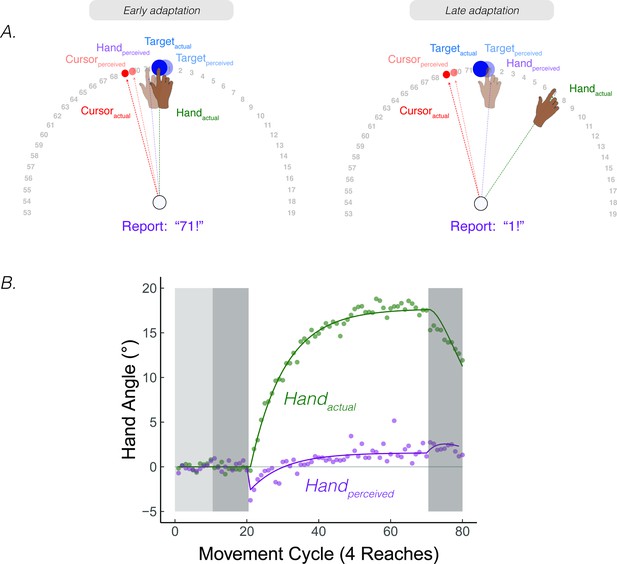
Continuous reports of perceived hand position during implicit adaptation.
(A) On each trial, participants reached to a target (blue dot) with and received 15° clamped visual feedback (red dot). After each reach, a number wheel appeared on the screen, prompting the participant to verbally report the angular position of their (unseen) hand. Participants perceived their hand on the left side of the target, shifted toward the clamped visual cursor early in adaptation. The left side shows the state of each variable contributing to perceived hand position right after the introduction of the clamp and the right side shows their states late in the adaptation block. (B) After baseline trials (light grey = veridical feedback, dark grey = no feedback), participants exhibited 20° of implicit adaptation (green) while the hand reports (purple) showed an initial bias towards the visual cursor, followed by a reversal, eventually overshooting the target by ~2°. Lines denote model fits of the proprioceptive re-alignment model (). Figure adapted from Figure 2 of Tsay et al., 2020a.
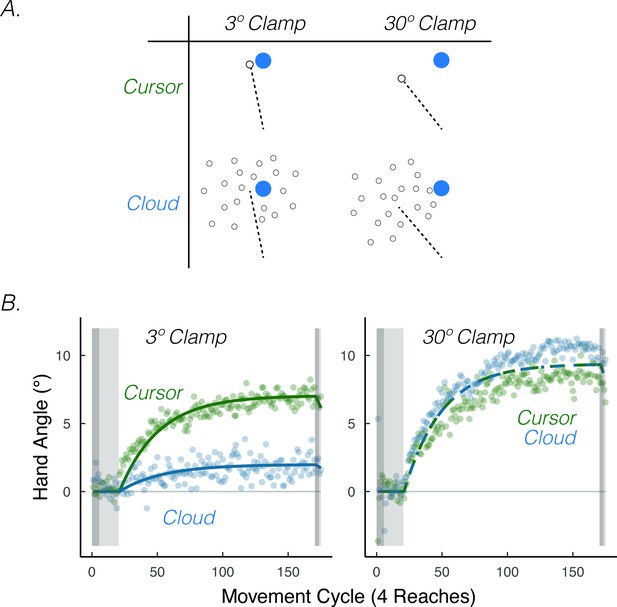
Visual uncertainty attenuates implicit motor adaptation in response to small visual errors, but not large visual errors.
(A) Experimental design in Tsay et al., 2020a. Participants made reaching movements in a similar setup as Figure 1A. Feedback was provided as a small 3.5° visual clamp or large 30° visual clamp, either in the form of a cursor or cloud (2 × 2 between-subject factorial design). (B) Tsay et al., 2020a Results. Implicit adaptation was attenuated by the cloud feedback when the clamp size was 3.5° but not when the clamp size was 30°. Lines denote model fits of the proprioceptive re-alignment model () Figure adapted from Figure 3 of Tsay et al., 2020b.
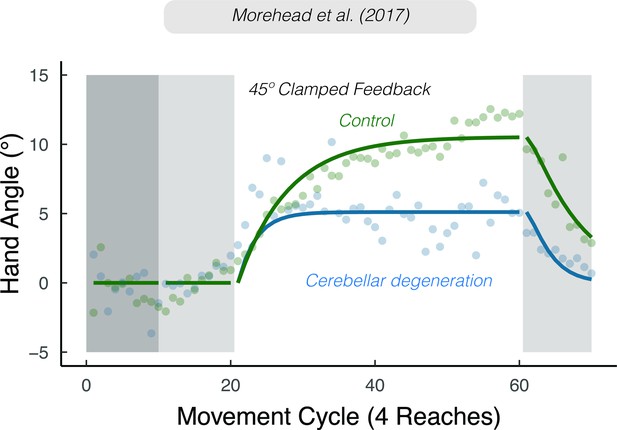
Sensory prediction uncertainty attenuates implicit adaptation.
Attenuated adaptation in individuals with cerebellar degeneration compared to matched controls in response to 45° clamped feedback. Lines denote model fits of the proprioceptive re-alignment model (). Figure adapted from Figure 3a of Morehead et al., 2017.
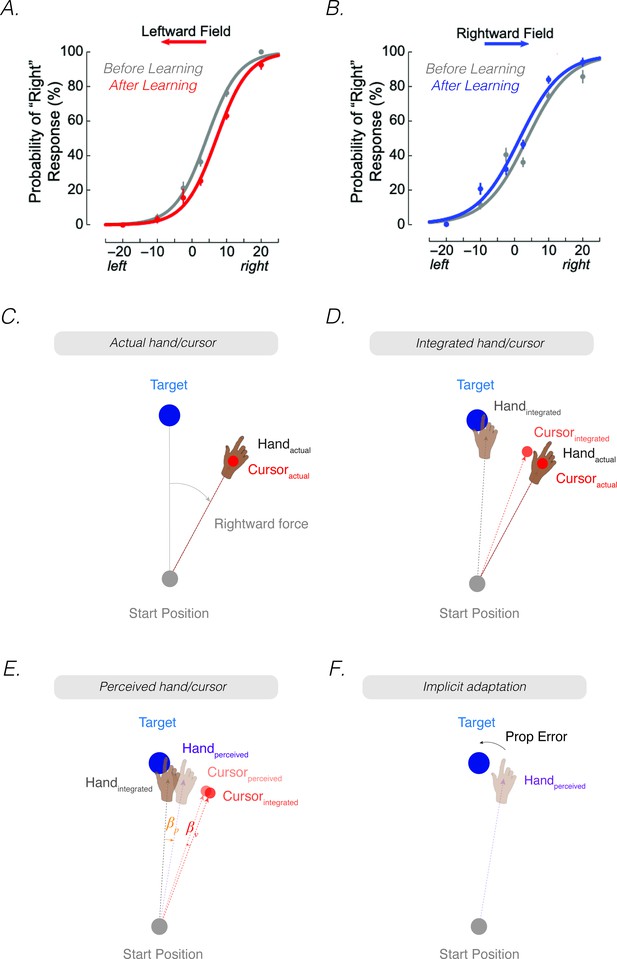
The proprioceptive re-alignment model explains sensory and motor changes during force-field adaptation.
(A, B) Participants’ perceptual judgments of actual hand position are biased in the direction of the recently experienced force-field. Figure adapted from Figure 2 of Ostry et al., 2010. These psychometric curves were obtained using a staircase method performed before and after force-field adaptation: the participant made center-out reaching movements toward a target. Force channels pushed the participant’s hand towards the left or right of the target by varying amounts. At the end of the movement, the participant judged the position of their hand relative to the target (left or right). Each participant’s shift quantified as the change in the point of subjective equality (PSE). The shift in the PSE in panels A and B indicate that the perceived hand position following force-field adaptation was shifted in the direction of the force-field. (C) Upon introduction of the force-field perturbation, the hand and (veridical) cursor are displaced in the direction of the force-field, especially at peak velocity. Note that the hand and cursor positions are illustrated at the endpoint position for ease of exposition. (D) Assuming that proprioceptive uncertainty is greater than visual uncertainty, the integrated hand position would be closer to the target than the integrated cursor position due to principles of optimal integration. (E) The two integrated positions then mutually calibrate, resulting in a proprioceptive shift () of the integrated hand towards the integrated cursor position, and a visual shift () of the integrated cursor position towards the integrated hand position, forming the perceived hand and perceived cursor locations. (F) The proprioceptive error (mismatch between the perceived hand position and the target) drives adaptation, a force profile in the opposition direction of the force-field.