Postsynaptic plasticity of cholinergic synapses underlies the induction and expression of appetitive and familiarity memories in Drosophila
Figures
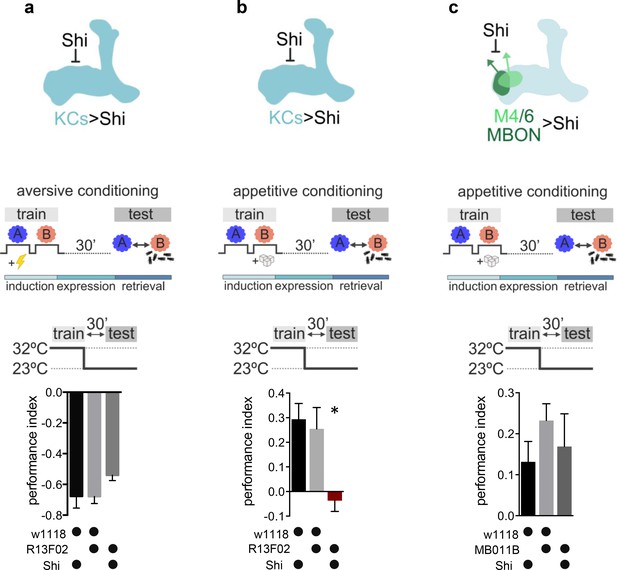
Kenyon cell (KC) neurotransmitter release is required for the acquisition of appetitive memories.
(a–c) Flies expressing temperature-sensitive Shibire (Shi) within KCs or mushroom bodies output neurons (MBONs) are trained at restrictive temperature (32°C), and subsequently placed at permissive temperature (23°C) throughout the consolidation and retrieval phase. Memory performance was tested 30 min after training at permissive temperature. Shi blocks neurotransmitter release at 32°C. (a) Block of neurotransmitter release from KCs (driver line R13F02-Gal4) during training does not impact 30-min aversive memory performance. Bar graphs: mean ± SEM; n=7–8; one-way ANOVA followed by Tukey’s test (p>0.05). (b) Block of neurotransmitter release from KCs (driver line R13F02-Gal4) during training impairs 30-min appetitive memory performance. Bar graphs: mean ± SEM; n=10–16; Kruskal-Wallis followed by Dunn’s test (p<0.05), *p<0.05. (c) Block of neurotransmitter release from M4/6 MBONs (driver line MB011B [Split-GAL4]) during training does not impact 30-min appetitive memory performance. Bar graphs: mean ± SEM; n=14–24; one-way ANOVA followed by Tukey’s test (p>0.05). Also see Figure 1—figure supplement 1 for further information.
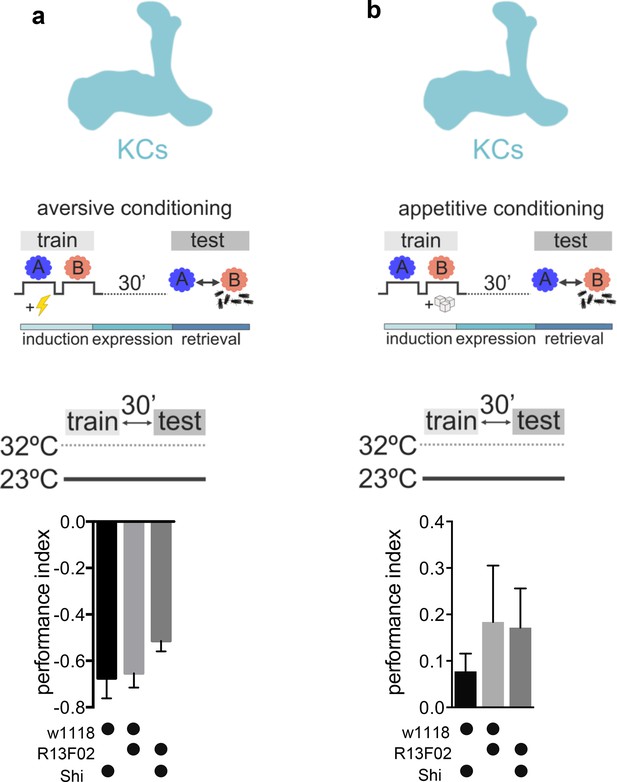
Permissive temperature controls accompanying Figure 1.
(a) Permissive temperature control for experiments shown in Figure 1a. A 30-min aversive memory performance when training at 23°C (driver line R13F02-Gal4). Bar graphs: mean ± SEM; n=7–9; Kruskal-Wallis followed by Dunn’s test (p>0.05). (b) Permissive temperature control for experiments shown in Figure 1b. 30-min appetitive memory performance when training at 23°C (driver line R13F02-Gal4). Bar graphs: mean ± SEM; n=6–8; Kruskal-Wallis followed by Dunn’s test (p>0.05).
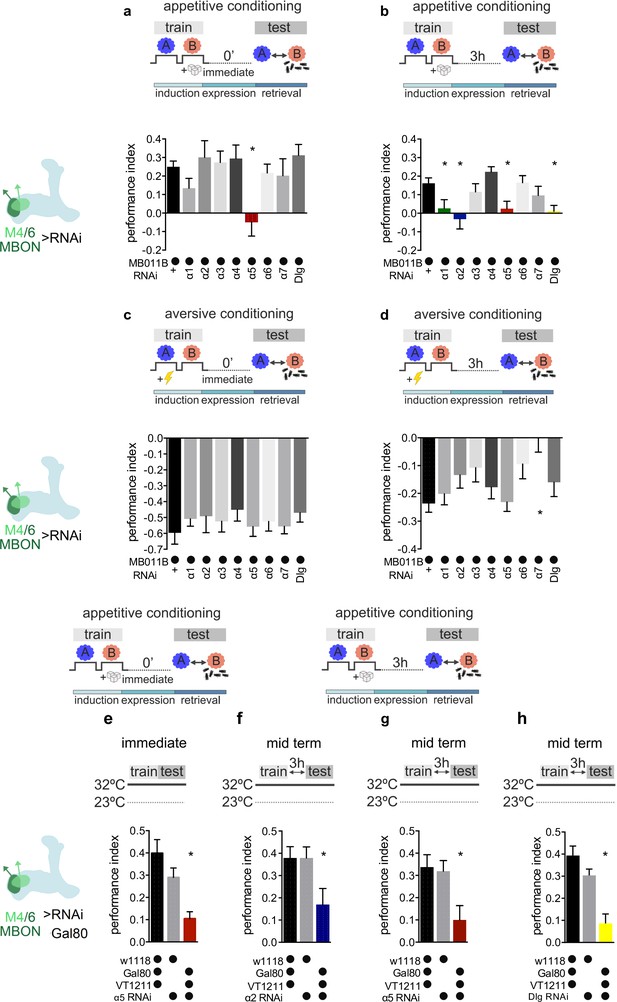
Specific nicotinic acetylcholine receptor (nAChR) α subunits are needed for specific memories in M4/6 neurons.
Training and testing protocols indicated schematically. A and B indicate different odors. (a) Immediate appetitive memories are impaired following RNAi knockdown of the α5 nAChR subunit in M4/6 mushroom bodies output neurons (MBONs; driver line MB011B [Split-GAL4]). Bar graphs: mean ± SEM; n=8–13, for controls: n=20; one-way ANOVA followed by Dunnett’s test (p<0.05), *p<0.05. Note: data depicted correspond to initial screen, please see Figure 2—figure supplement 2e for alternate display including all genetic controls. (b) RNAi knockdown of the α1, α2, α5 nAChR subunits or discs large (Dlg) in M4/6 MBONs (driver line MB011B [Split-GAL4]) impairs 3-hr appetitive memories. Bar graphs: mean ± SEM; n=12–26, for controls: n=38; Kruskal-Wallis followed by Dunn’s test (p<0.05), *p<0.05. Note: data depicted correspond to initial screen, please see Figure 2—figure supplement 2 f–i for alternate display including all genetic controls. (c) Immediate aversive learning is not impaired by RNAi knockdown of any subunit in M4/6 MBONs (driver line MB011B [Split-GAL4]). Bar graphs: mean ± SEM; n=6–8, for controls: n=12; Kruskal-Wallis followed by Dunn’s test (p>0.05). (d) 3-hr aversive memory is not affected by knockdown of α subunits with the exception of α7 (driver line MB011B [Split-GAL4]). Bar graphs: mean ± SEM; n=21–32, for controls: n=61. Kruskal-Wallis followed by Dunn’s test (p<0.05), *p<0.05. (e) RNAi knockdown of the α5 subunit in M4/6 MBONs (driver line VT1211-Gal4) is suppressed during development using Gal80ts. 3–5 days before the experiment RNAi knockdown was induced. Immediate memory is significantly impaired. Bar graphs: mean ± SEM; n=6–7; Kruskal-Wallis followed by Dunn’s test (p<0.05), *p<0.05. (f) RNAi knockdown of the α2 subunit in M4/6 MBONs (driver line VT1211-Gal4) is suppressed during development using Gal80ts. 3–5 days before the experiment RNAi knockdown was induced. 3-hr memories are significantly impaired. Bar graphs: mean ± SEM; n=16–17; one-way ANOVA followed by Tukey’s test (p<0.05), *p<0.05. (g) RNAi knockdown of the α5 subunit in M4/6 MBONs (driver line VT1211-Gal4) is suppressed during development using Gal80ts. 3–5 days before the experiment RNAi knockdown was induced. 3-hr memories are significantly impaired. Bar graphs: mean ± SEM; n=25–27; one-way ANOVA followed by Tukey’s test (p<0.05), *p<0.05. (h) RNAi knockdown of Dlg in M4/6 MBONs (driver line VT1211-Gal4) is suppressed during development using Gal80ts. 3–5 days before the experiment RNAi knockdown was induced. 3-hr memories are significantly impaired. Bar graphs: mean ± SEM; n=8–11; one-way ANOVA followed by Tukey’s test (p<0.05), *p<0.05. Also see Figure 2—figure supplements 1 and 2 for further information.
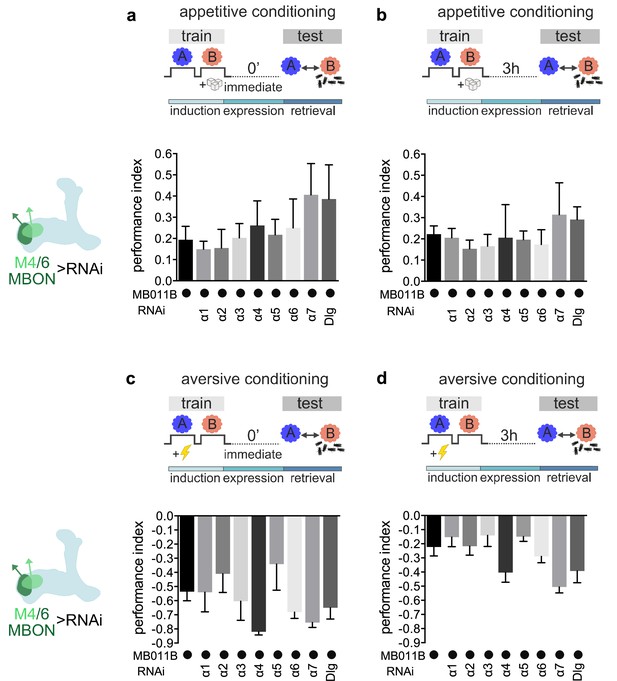
Genetic controls and alternate data display of data presented in Figure 2.
(a) Immediate appetitive memory is not impaired in genetic control groups. Bar graphs: mean ± SEM; n=7–11, for controls: n=16, one-way ANOVA followed by Tukey’s test (p>0.05). (b) 3-hr appetitive memory is not impaired in genetic control groups. Bar graphs: mean ± SEM; n=7–33, for controls: n=41, one-way ANOVA followed by Tukey’s test (p>0.05). (c) Immediate aversive learning is not impaired in genetic control groups. Bar graphs: mean ± SEM; n=6–10, for controls: n=13, Kruskal-Wallis followed by Dunn’s test (p>0.05). (d) 3-hr aversive memory is not impaired in genetic control groups. Bar graphs: mean ± SEM; n=6–10, for controls: n=15, Kruskal-Wallis followed by Dunn’s test (p>0.05). Note that data in panels a–d were replotted to allow comparison between all genotypes.
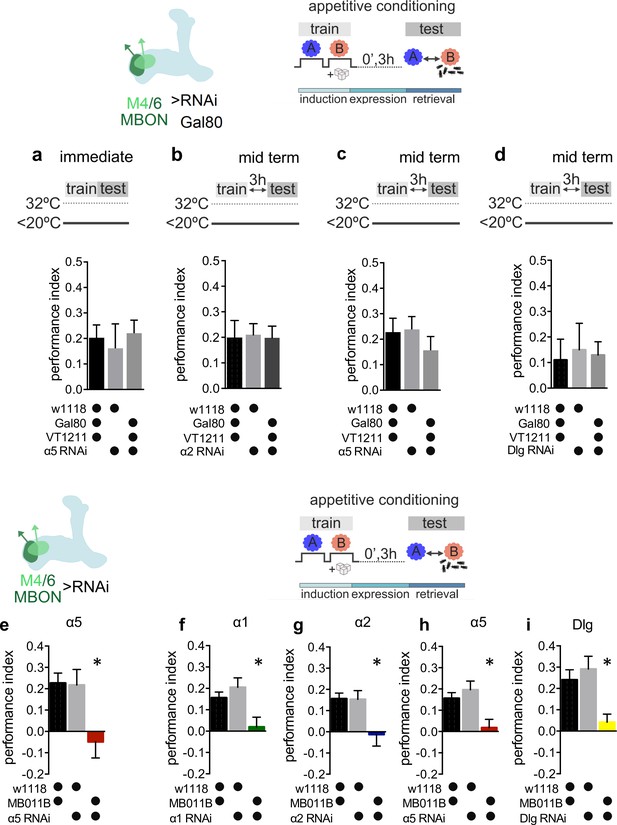
Genetic controls and alternate data display of data presented in Figure 2.
(a) RNAi knockdown of the α5 subunit in M4/6 mushroom bodies output neurons (MBONs; driver line VT1211-Gal4) is suppressed using Gal80ts. Immediate memories are not impaired. Bar graphs: mean ± SEM; n=9; one-way ANOVA followed by Tukey’s test (p>0.05). (b) RNAi knockdown of the α2 subunit in M4/6 MBONs (driver line VT1211-Gal4) is suppressed using Gal80ts. Midterm memory is not impaired. Bar graphs: mean ± SEM; n=9–11, one-way ANOVA followed by Tukey’s test (p>0.05). (c) RNAi knockdown of the α5 subunit in M4/6 MBONs (driver line VT1211-Gal4) is suppressed using Gal80ts. Midterm memory is not impaired. Bar graphs: mean ± SEM; n=10–11, one-way ANOVA followed by Tukey’s test (p>0.05). (d) RNAi knockdown of discs large (Dlg) in M4/6 MBONs (driver line VT1211-Gal4) is suppressed using Gal80ts. Midterm memory is not impaired. Bar graphs: mean ± SEM; n=8–10, one-way ANOVA followed by Tukey’s test (p>0.05). (i–m) Alternate display (see Methods for details) for appetitive memory experiments shown in Figure 2a and b: (e) Immediate appetitive memories are impaired following RNAi knockdown of the α5 nicotinic acetylcholine receptor (nAChR) subunit in M4/6 MBONs (driver line MB011B [Split-GAL4]). Bar graphs: mean ± SEM; n=9–21, one-way ANOVA followed by Tukey’s test (p<0.05), *p<0.05. (f) 3-hr appetitive learning is impaired by RNAi knockdown of the α1 nAChR subunit in M4/6 MBONs (driver line MB011B [Split-GAL4]). Bar graphs: mean ± SEM; n=25–51, Kruskal-Wallis followed by Dunn’s test (p<0.05), *p<0.05. (g) RNAi knockdown of the α2 nAChR subunit in M4/6 MBONs (driver line MB011B [Split-GAL4]) impairs 3-hr appetitive memories. Bar graphs: mean ± SEM; n=27–51, Kruskal-Wallis followed by Dunn’s test (p<0.05), *p<0.05. (h) 3-hour appetitive learning is impaired by RNAi knockdown of the α5 nAChR subunit in M4/6 MBONs (driver line MB011B [Split-GAL4]). Bar graphs: mean ± SEM; n=27–51, one-way ANOVA followed by Tukey’s test (p<0.05), *p<0.05. (i) RNAi knockdown of Dlg in M4/6 MBONs (driver line MB011B [Split-GAL4]) impairs 3-hr appetitive memories. Bar graphs: mean ± SEM, n=13–31; one-way ANOVA followed by Tukey’s test (p<0.05), *p<0.05. Note that data in panels e–i were replotted to allow comparison between all genotypes.
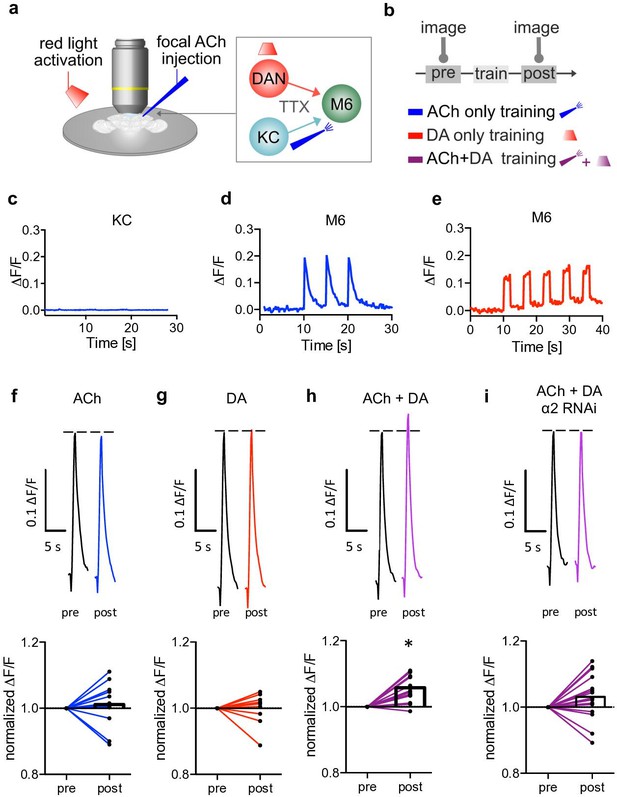
Induction of postsynaptic plasticity bypassing the presynapses.
(a) Left: explant brain experimental configuration. Right: connectivity scheme of mushroom bodies (MB) output synapses. Cholinergic Kenyon cells (KCs) and dopaminergic neurons are presynaptic to M6 MB output neurons (MBONs). Only connections relevant for this protocol are shown for simplicity. Red light pulses trigger release of dopamine (DA) from dopaminergic neurons (R58E02-LexA>lexAop-CsChrimsontdTomato), while KC input is circumvented and mimicked by focal acetylcholine (ACh; 0.1 mM) injections to M6 dendrites in an explant brain preparation. Postsynaptic responses at the level of M6 are measured using GCaMP6f (MB011B [Split-GAL4] >UAS-GCaMP6f). TTX (tetrodotoxine) in the bath suppresses feedback signaling and overall network activity within the circuit. (b) Training scheme (top). Baseline responses to ACh application were initially established (pre). Subsequent training protocols consist of either pairing ACh application with simultaneous activation of dopaminergic neurons (purple connection lines), activation of dopaminergic neurons (red light only, red connection lines), or ACh only (blue, connection lines). This is followed by a test trial (post) through ACh application. (c) Averaged traces of axonal KC calcium changes induced by focal ACh injections. No apparent transients are observable; n=7. Line is mean ± SEM. (d) Sample trace of dendritic M6 calcium changes induced by focal ACh injections. (e) Sample trace of dendritic M6 calcium changes induced by red-light pulses. (f-i) Above: sample calcium traces in response to ACh injections recorded from M6 dendrites pre (black traces) and post (colored traces) training. Below: peak quantification. (f) Changes in ACh-evoked calcium transients; comparison of mean peaks pre- and post-‘ACh only’ training. Before-after plots and bar graphs (mean); n=13, ratio paired t-test. (g) Changes in ACh-evoked calcium transients; comparison of mean peaks pre- and post ‘red light only’ training. Before-after plots and bar graphs (mean); n=10, ratio paired t-test. (h) Changes in ACh-evoked calcium transients; comparison of mean peaks pre- and post ‘paired’ training. Before-after plots and bar graphs (mean); n=18, ratio paired t-test, *p<0.05. (i) Changes in ACh-evoked calcium transients following RNAi knockdown of the α2 subunit in M4/6 MBONs; comparison of mean peaks pre- and post ‘paired’ training. Before-after plots and bar graphs (mean); n=15, ratio paired t-test. Also see Figure 3—figure supplement 1 for further information.
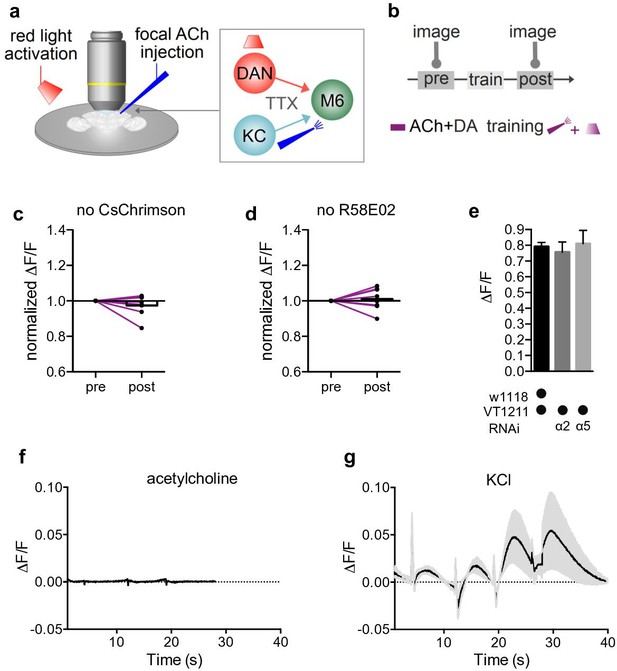
Control experiments and non-normalized data display for Figure 3.
(a) Explant brain configuration and connectivity scheme of mushroom bodies (MB) output synapses. Also shown in Figure 3. (b) Training scheme. (c) Control experiments for Figure 3f–h. Paired training protocol in the absence of LexAoP-CsChrimson. Before-after plots and bar graphs (mean); n=9, ratio paired t-test. (d) Control experiments for Figure 3f–h. Paired training protocol in the absence of R58E02-LexA. Before-after plots and bar graphs (mean); n=8, Wilcoxon matched-pairs signed rank test. (e) GCaMP6f responses following focal acetylcholine (ACh) injections to the γ5 compartment of the MB are not significantly altered following α2 or α5 knockdown in the M4/6 MB output neurons (driver line VT1211-Gal4). The bath contains TTX to suppress spontaneous neural activity. Bar graphs: mean ± SEM; n=12–23, one-way ANOVA followed by Dunnett’s test (p>0.05). (f) Averaged SynaptopHluorin responses of KCs following focal ACh injections (0.1 mM) to the γ5 compartment of the MB; OK107-Gal4 driver (KCs), n=8. Shaded area depicts SEM. (g) Averaged KC SynaptopHluorin responses following focal KCl injections (300–400 mM) to the γ5 compartment of the MB; OK107-Gal4 driver (KCs), n=4. Shaded area depicts SEM.
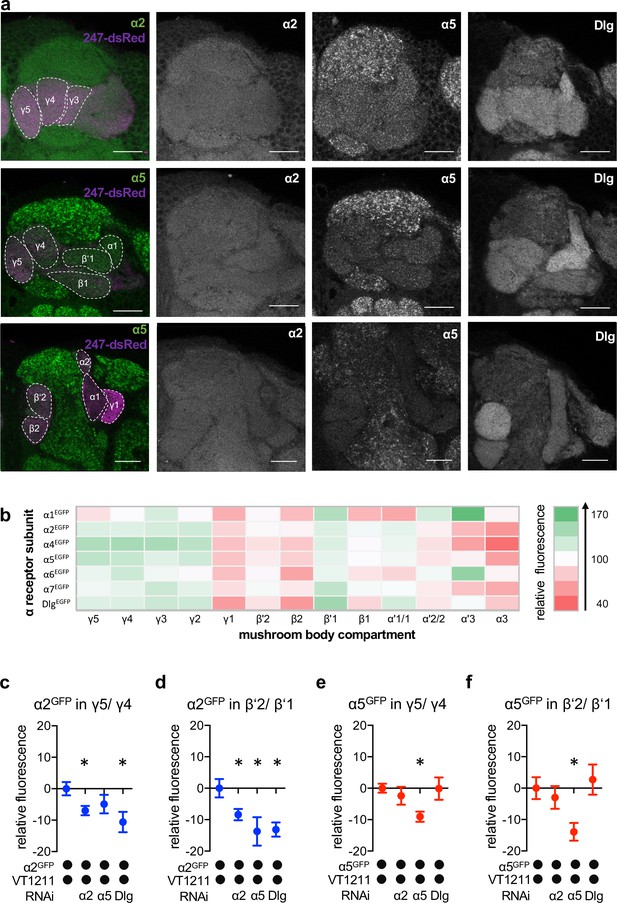
Nicotinic acetylcholine receptor (nAChR) α subunit localization throughout the mushroom bodies (MB): MB output neuron (MBON)-specific RNAi alters subunit distribution.
(a) Representative images of the GFP-tagged nAChR subunits α2 and α5 as well as discs large (Dlg). Individual images displayed here are taken from different animals. For other subunits, see Figure 4—figure supplement 1. Scale bar: 20 μm. Left: merge of α subunit signal (green) with MB compartments marked with 247-dsRed (magenta). Compartments are indicated by dashed lines. Top row: γ compartments; middle row: α, β’, β, and γ compartments, bottom row: α’, α, and β compartments. (b) Quantification of all GFP-tagged α receptors (except for the α3 subunit). GFP signals for the indicated MB compartments are relative to the mean intensity of the GFP signal of the complete MB; n=7–18. (c) Knockdown of Dlg or α2 in M4/6 neurons (driver line VT1211-Gal4) significantly reduces the α2GFP fluorescence within the γ5 compartment (relative to unmanipulated γ4). Bar graph: normalized mean ± SEM; n=8–20; Kruskal-Wallis followed by Dunn’s test (p<0.05), *p<0.05. (d) Knockdown of either the α2 or the α5 nAChR subunit or Dlg in M4/6 neurons (driver line VT1211-Gal4) decreases the relative fluorescence signal of α2GFP in the β’2 compartment (relative to unmanipulated β’1). Bar graph: normalized mean ± SEM; n=9–20; one-way ANOVA followed by Dunnett’s test (p<0.05), *p<0.05. (e) Knockdown of α5 in M4/6 neurons (driver line VT1211-Gal4) decreases the α5GFP signal in the γ5 compartment (relative to unmanipulated γ4). Bar graph: normalized mean ± SEM; n=9–28; Kruskal-Wallis followed by Dunn’s test (p<0.05), *p<0.05. (f) α5GFP fluorescence is significantly decreased in the β’2 compartment (relative to unmanipulated β’1) after knockdown of α5 in M4/6 neurons (driver line VT1211-Gal4). Bar graph: normalized mean ± SEM; n=10–28; Kruskal-Wallis followed by Dunn’s test (p<0.05), *p<0.05. Also see Figure 4—figure supplement 1 for further information.
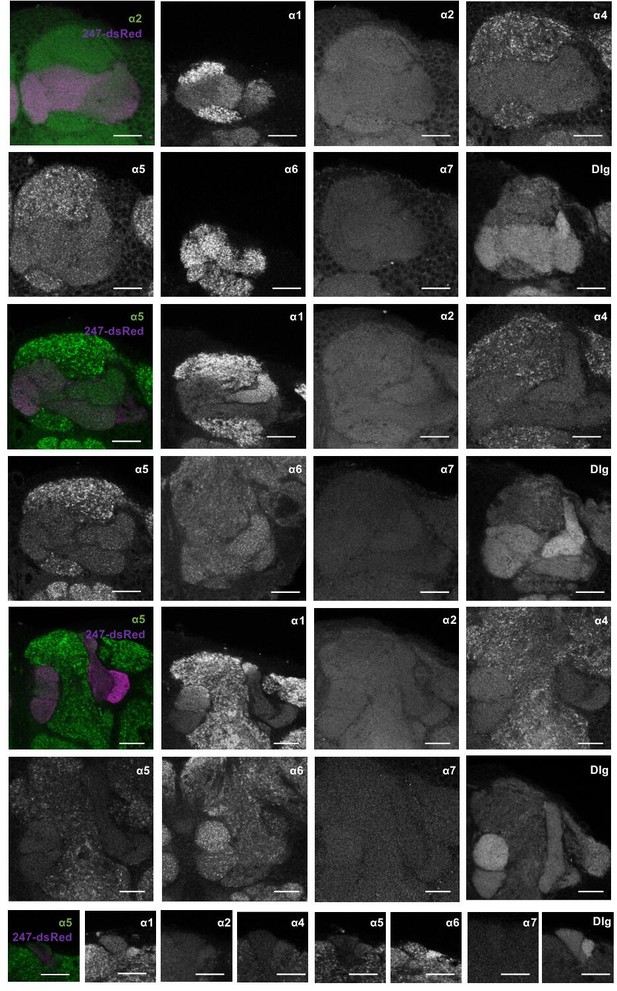
Detailed distribution of α subunits in the mushroom bodies (MB) accompanying Figure 4.
Example image planes of GFP expression at the level of the MB compartments for all α nicotinic acetylcholine receptor subunits (except for α3) and discs large. Pictures are taken from different animals. Scale bar: 20 μm. Left: merged image of α subunit signal (green) with MB compartments marked by 247-dsRed (magenta). Top two rows: γ compartments; middle two rows: γ, β’, and β compartments, bottom three rows: α’/β’ and α/β, with bottom row: α’ and α compartments.
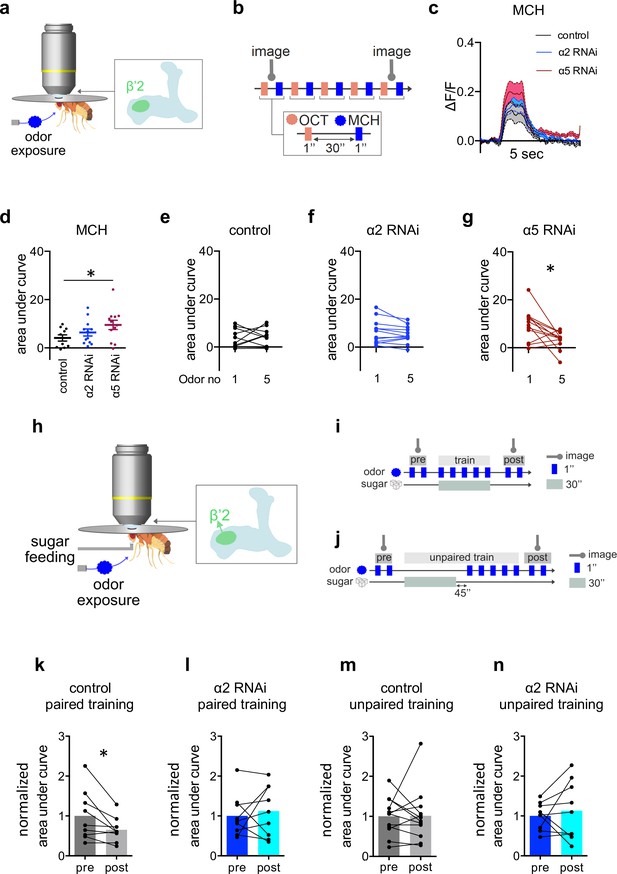
α2 is required for learning-associated plasticity in vivo.
(a) Scheme indicating the dendritic imaging area (c–g) at the level of the β’2 compartment. (b) Odor exposure protocol. Five octanol (OCT) stimuli were alternatingly administered with five methylcyclohexanol (MCH) stimuli. 1-s odor puffs were separated by 30 s breaks. (c) Averaged traces of GCaMP6f (calcium) responses to MCH from control (black), α2 (blue), and α5 RNAi (red; driven in M4/6, respectively, driver line VT1211-Gal4) flies. Solid traces are mean, shaded areas SEM; n=10–12. (d) Area under curve (AUC) quantification of averaged initial odor responses to MCH following α5 knockdown in M4/6 neurons (driver line VT1211-Gal4). Mean ± SEM; one-way ANOVA followed by Dunnett’s test; *p<0.05; n=10–12. (e) Control flies show no significant increase between the first and the fifth response to MCH. n=10; paired t-test. (f) α2 RNAi flies show no difference between the first and fifth odor response to MCH. Nicotinic acetylcholine receptor (nAChR) subunit RNAi is driven in M4/6 neurons (driver line VT1211-Gal4). n=12; paired t- test. (g) α5 RNAi flies show a significant decrease in calcium transients over the course of consecutive odor exposures. nAChR subunit RNAi is driven in M4/6 neurons (driver line VT1211-Gal4). n=12; Wilcoxon matched-pairs signed rank test; *p<0.05. (h) Arrow in scheme for absolute training indicates the axonal imaging area of M4 (k–n). (i) Scheme for absolute paired training under the microscope: flies are exposed to two brief stimuli of the odor (MCH) before training while recording GCaMP6f signals (preresponse). Immediately after, flies are presented with a sugar solution for 30 s accompanied by 5 pulses of odor stimuli. 1 min after training, flies are exposed to two brief odor stimuli again while recording GCaMP6f signals (postresponse). Axonal imaging area indicated by arrow. (j) Scheme for absolute unpaired training under the microscope: as in (i) except flies are exposed to 5 pulses of odor stimuli 45 s after presenting the sugar solution for 30 s. 1 min after training, the flies are exposed to two brief odor stimuli again while recording GCaMP6f signals (postresponse). Axonal imaging area indicated by arrow. (k) Control flies show a significant decrease in odor-evoked GCaMP6f signals. AUC of response to MCH (trained odor) following paired training (driver line VT1211-Gal4, axonal imaging). Before-after plots and bar graphs (mean); n=9; paired t-test; *p<0.05. (l) α2 RNAi flies show no significant decrease in odor-evoked GCaMP6f signals. AUC of response to MCH (trained odor) following paired training (driver line VT1211-Gal4, axonal imaging). Before-after plots and bar graphs (mean); n=9; paired t-test. (m) Control flies show no significant decrease in odor-evoked GCaMP6f signals. AUC of response to MCH (trained odor) following unpaired training (driver line VT1211-Gal4, axonal imaging). Before-after plots and bar graphs (mean); n=12; Wilcoxon matched-pairs signed rank test. (n) α2 RNAi flies show no significant decrease in odor-evoked GCaMP6f signals. AUC of response to MCH (trained odor) following unpaired training (driver line VT1211-Gal4, axonal imaging). Before-after plots and bar graphs (mean); n=9; paired t-test. Also see Figure 5—figure supplement 1 for further information.
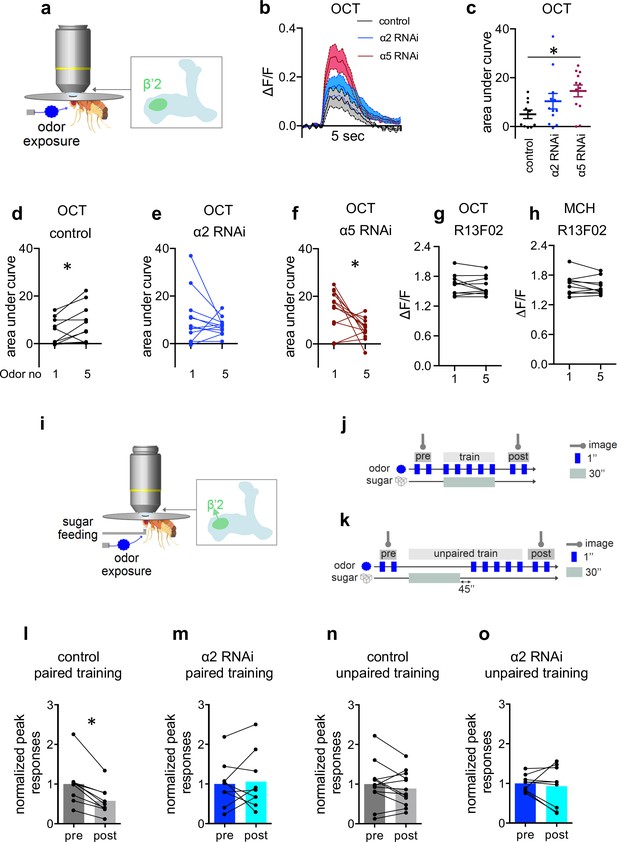
Additional data and display of non-normalized data accompanying Figure 5.
(a) Scheme indicating the dendritic imaging area at the level of the β’2 compartment. (b) Averaged traces of GCaMP6f responses to octanol (OCT) from control (black), α2 subunit RNAi (blue), and α5 subunit RNAi (red; driven in M4/6, respectively; VT1211-Gal4 as driver line) flies. Solid traces are mean, shaded areas SEM; n=10–12. (c) Area under curve quantifications of averaged odor responses show significantly elevated initial odor responses to OCT following α5 knockdown in M4/6 neurons (driver line VT1211-Gal4). Mean ± SEM; n=10–12; Kruskal-Wallis followed by Dunn’s test (p<0.05); *p<0.05. (d) Control flies show a significant increase between the first and the fifth response to OCT. Before-after plots; n=10; paired t-test; *p<0.05. (e) α2 RNAi flies show no difference between the first and fifth odor response to OCT. Nicotinic acetylcholine receptor (nAChR) subunit RNAi is driven in M4/6 neurons (driver line VT1211-Gal4). Before-after plots; n=12; Wilcoxon matched-pairs signed rank test. (f) α5 RNAi flies show a significant decrease in calcium transients over the course of consecutive odor exposures. nAChR subunit RNAi is driven in M4/6 neurons (driver line VT1211-Gal4). Before-after plots; n=12; paired t-test; *p<0.05. (g) Kenyon cells (KCs; driver line R13F02-Gal4) show no significant change in calcium transients over the consecutive OCT odor exposures. Before-after plots. n=10, paired t- test. (h) KCs (driver line R13F02-Gal4) show no significant change in calcium transients over the consecutive methylcyclohexanol (MCH) odor exposures. Before-after plots. n=10, paired t- test. (i) Arrow in scheme for absolute training indicates the axonal imaging area of M4 (l–o). (j) Scheme for absolute paired training under the microscope: flies are exposed to two brief stimuli of the odor (MCH) before training while recording GCaMP6f signals (preresponse). Immediately after, flies are presented with a sugar solution for 30 s accompanied by 5 pulses of odor stimuli. 1 min after training, flies are exposed to two brief odor stimuli again while recording GCaMP6f signals (postresponse). (k) Scheme for absolute unpaired training under the microscope: as in (j) except flies are exposed to 5 pulses of odor stimuli 45 s after presenting the sugar solution for 30 s. 1 min after training, the flies are exposed to two brief odor stimuli again while recording GCaMP6f signals (postresponse). (l) Control flies show a significant decrease in in odor-evoked GCaMP6f signals. Peak responses to MCH (trained odor) following absolute paired training (driver line VT1211-Gal4, axonal imaging). Before-after plots and bar graphs (mean); n=9; Wilcoxon matched-pairs signed rank test; *p<0.05. (m) α2 RNAi flies show no significant decrease in odor-evoked GCaMP6f signals. Peak response to MCH (trained odor) following absolute paired training (driver line VT1211-Gal4, axonal imaging). Before-after plots and bar graphs (mean); n=9; paired t-test. (n) Control flies show no significant decrease in odor-evoked GCaMP6f signals. Peak response to MCH (trained odor) following absolute unpaired training (driver line VT1211-Gal4, axonal imaging). Before-after plots and bar graphs (mean); n=12; paired t-test. (o) α2 RNAi flies show no significant decrease in odor-evoked GCaMP6f signals. Peak response to MCH (trained odor) following absolute unpaired training (driver line VT1211-Gal4, axonal imaging). Before-after plots and bar graphs (mean); n=9; paired t-test.
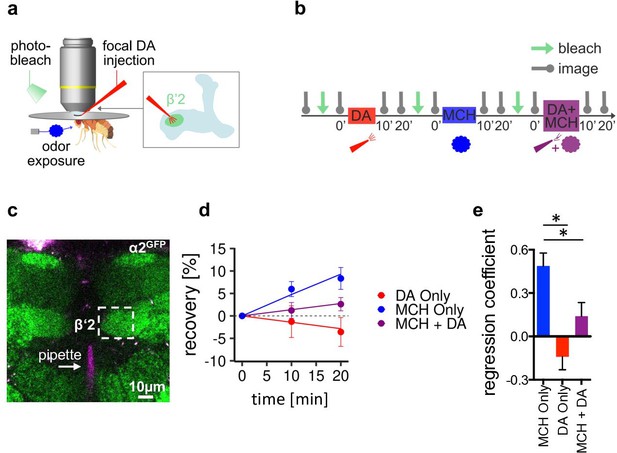
α2 nicotinic acetylcholine receptor (nAChR) subunits dynamically rearrange.
(a) In vivo imaging configuration and scheme of site of dopamine (DA) injection during fluorescence recovery after photobleaching (FRAP) experiments at the level of the Kenyon cell to mushroom body output neuron synapses of the β’2 compartment. (b) FRAP experimental protocol. After bleaching, the baseline picture was taken followed by DA injection, odor presentation, and odor presentation simultaneously with DA injection in the same fly. Fluorescence recovery was monitored at the 10- and 20-min time points. (c) Example image of α2GFP expression; white dashed box shows the β’2 output zone; DA injection pipette (with Texas red) is labeled in magenta. Scale bar: 10 µm. (d) Inverse exponential decay fit of FRAP following methylcyclohexanol (MCH) exposure (blue line), MCH exposure simultaneously with DA injection (purple line), and DA injection alone (red line). (e) Regression coefficient for the inverse exponential decay fit. Bar graphs: regression coefficients of recovery kinetics ± standard error of regression; n=9–10, linear mixed effects model followed by pairwise comparison from estimated marginal trends. *p < 0.05. Also see Figure 6—figure supplement 1 for further experiments.
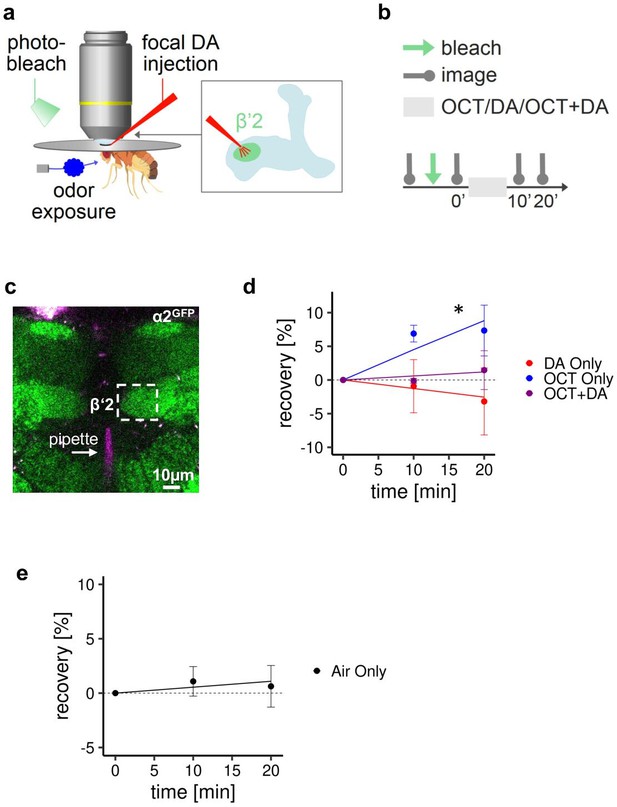
Receptor subunit recovery, accompanying Figure 6.
(a) In vivo imaging configuration (left) and scheme of site of dopamine (DA) injection during fluorescence recovery after photobleaching (FRAP) experiments at the level of the Kenyon cell to mushroom body output neuron synapses of the β’2 compartment. (b) FRAP experimental protocol. After bleaching, a baseline picture was taken followed by odor presentation or odor presentation simultaneously with DA injection or DA injection by itself. Fluorescence recovery was monitored at the 10- and 20-min time points. (c) Example image of α2GFP expression (the same image is shown here as in Figure 6); white dashed box shows the β’2 output zone; DA injection pipette (with Texas red) is labeled in magenta. Scale bar: 10 µm. (d) Inverse exponential decay fit of FRAP following octanol (OCT) exposure (blue line), or OCT exposure simultaneously with DA injection (purple line), or DA injection alone (red line). n=3–5, t-test, Satterthwaite’s method for approximating the degrees of freedom. *: regression coefficient p<0.05 in the linear mixed effect model. Only ‘OCT only’ exposure shows recovery that significantly differs from 0. (e) Control settings for Figure 6 and the experiments shown in this figure. After bleaching, α2GFP flies were exposed to air only. Inverse exponential decay fit of fluorescence recovery. n=9, t-test, Satterthwaite’s method for approximating the degrees of freedom. The regression coefficient is not significantly different from 0.
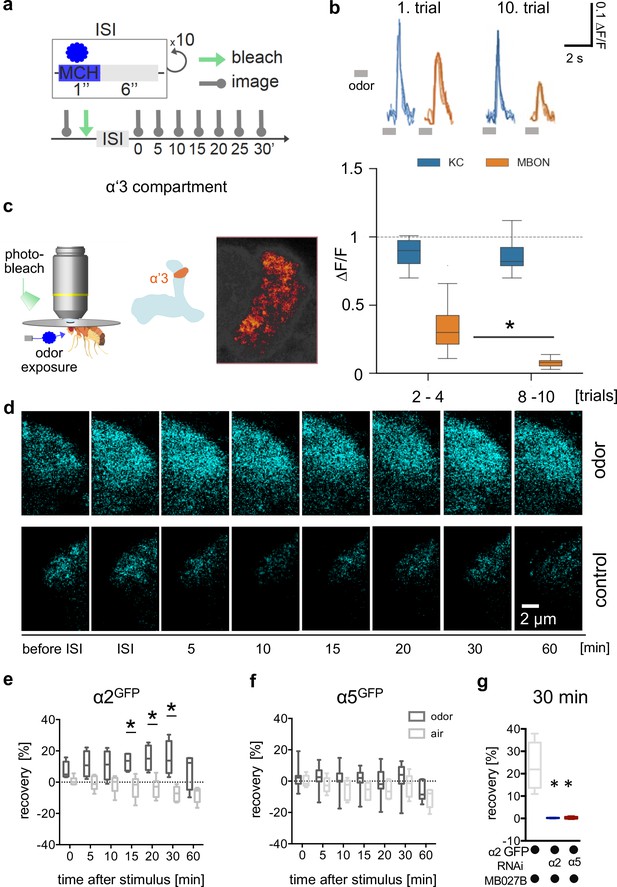
Non-associative plasticity alters postsynaptic α2 subunit receptor dynamics.
(a) Training scheme indicating odor application, bleaching, and imaging time points. MCH was given 10 times for 1 s with a pause of 6 s in-between. Images were taken after training in absence of odor immediately afterward and 5, 10, 15, 20, 30, and 60 min later. (b) Top: Calcium peaks in response to odor stimuli of presynaptic Kenyon cells (KCs; MB369B as driver line) and adjacent postsynaptic mushroom body output neurons (MBONs; driver line: MB027B [Split-GAL4]). Individual calcium responses to trials 1 and 10 for MBONs (orange lines) and KCs (blue lines). Bottom: Averaged calcium responses to odor stimuli of presynaptic KCs and postsynaptic MBONs of trials 2–4 and 8–10, respectively. Responses decrease at the level of MBONs but not at the level of KCs over 10 trials. Box plots are median and 75% quartiles; n=15; Kruskal-Wallis followed by Dunn’s test (p<0.05), *p<0.05. (c) In vivo imaging configuration (left), scheme of α’3 compartment analyzed (right), and representative α5GFP fluorescent image (smoothed). Scale bar: 10 μm. (d) Example images of α2GFP fluorescence recovery after photobleaching (FRAP) experiment at the level of the α’3 compartment at specific time points before and after training. Top row: after training; bottom row: control settings. Scale bar: 2 μm. (e) FRAP of α2GFP nicotinic acetylcholine receptor (nAChR) subunit in the α’3 compartment after odor presentation. α2GFP shows significant recovery following odor training compared to the controls. Recovery rate is normalized to the baseline recorded after selective bleaching of the α’3 mushroom bodies (MB) compartment. Box plots are median and 75% quartiles; n=4–6; multiple t-tests with Sidak-Bonferroni correction, *p<0.05. (f) FRAP of α5GFP subunit in the α’3 compartment after odor presentation. α5GFP did not show significant recovery compared to the controls. Recovery rate is normalized to the baseline recorded after selective bleaching of α’3 MB compartment. Box plots are median and 75% quartiles; n=5–7, multiple t-tests with Sidak-Bonferroni correction. (g) FRAP of α2GFP nAChR subunit in the α’3 compartment after odor presentation and knockdown of either the α2 or α5 subunit (RNAi) in the α’3 MBON (driver line MB027B [Split-GAL4]). α2GFP shows no recovery 30 min after odor training in α2 or α5 knockdown animals compared to the controls. Recovery rate is normalized to the baseline recorded after selective bleaching of the α’3 MB compartment. Box plots are minimum value to maximum value; n=4–5; Kruskal-Wallis followed by Dunn’s test (p<0.05), *p<0.05. Also see Figure 7—figure supplement 1 for further information.

Discs large GFP (DlgGFP) fluorescence recovery after photobleaching (FRAP), accompanying Figure 7.
(a) FRAP of DlgGFP in α’3 mushroom body (MBs) output neurons after odor presentation. DlgGFP did not show significant recovery following odor training compared to the controls. Recovery rate is normalized to the baseline recorded after selective bleaching of the α’3 MB compartment. n=5–7; multiple t-tests with Sidak-Bonferroni correction.
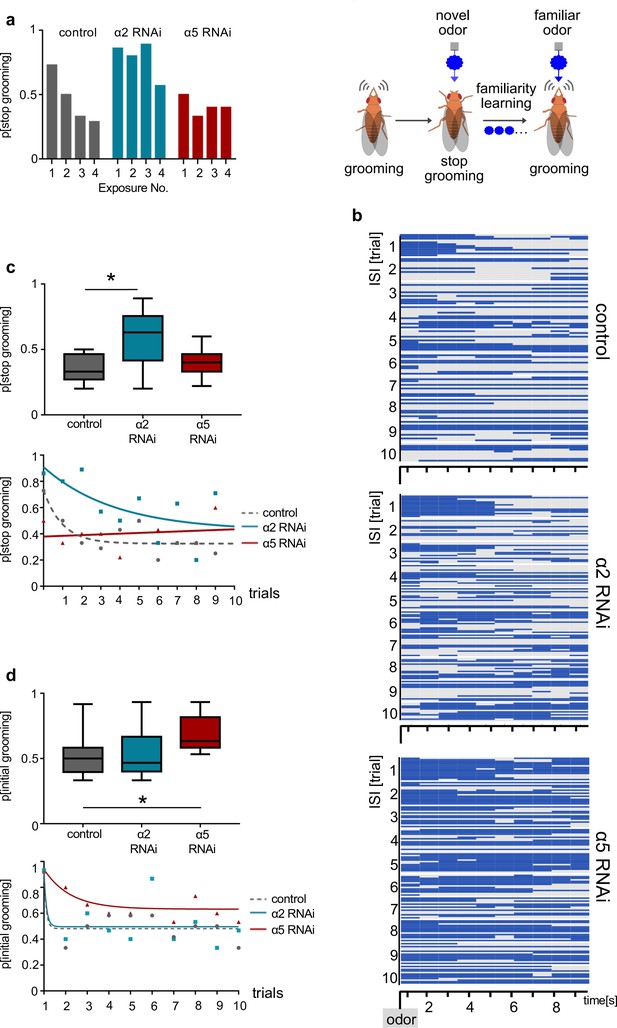
α2 and α5 nicotinic acetylcholine receptor (nAChR) subunits are required for non-associative familiarity learning at the level of α’3 mushroom body output neurons (MBONs).
(a) Scheme of behavioral responses to novel and familiar odors (right). (Left): Knockdown of α nAChR subunits at the level of α’3 MBONs alters odor familiarity learning and the probability to stop grooming. α2 RNAi knockdown delays familiarity learning effects to novel odors. α5 RNAi knockdown flies do not show a novelty response at all. (b) Grooming behavior response of dusted flies following the repeated presentations of a novel odor (MCH). Ethogram of grooming behavior (blue) during 10 intervals of odor exposures. Horizontal lines in each trial correspond to a single experimental fly within a trial group. Not grooming (gray) flies can further be categorized between pausing and wandering (see Figure 8—figure supplement 1). n=15. (c) Knockdown of α2 subunit in α’3 MBONs (driver line MB027B [Split-GAL4]) impairs odor familiarity learning significantly; animals show a higher probability to terminate grooming responses during the learning period. The learning period is defined as the odor exposure rounds following the first exposure. Bottom graph: Non-linear representation of grooming flies over 10 training trials. Note that α5 behavioral responses are best described by linear representation. Box plots are median and 75% quartiles; n=9, one-way ANOVA followed by Dunnett’s test (p<0.05) *p<0.05. (d) Knockdown of α5 subunits in α’3 MBONs (driver line MB027B [Split-GAL4]) leads to an increased probability to start grooming earlier. Bottom graph, non-linear representation of grooming flies over 10 training trials. Box plots are median and 75% quartiles; n=9, Kruskal-Wallis followed by Dunn’s test (p<0.05), *p<0.05. Also see Figure 8—figure supplement 1 for further information.
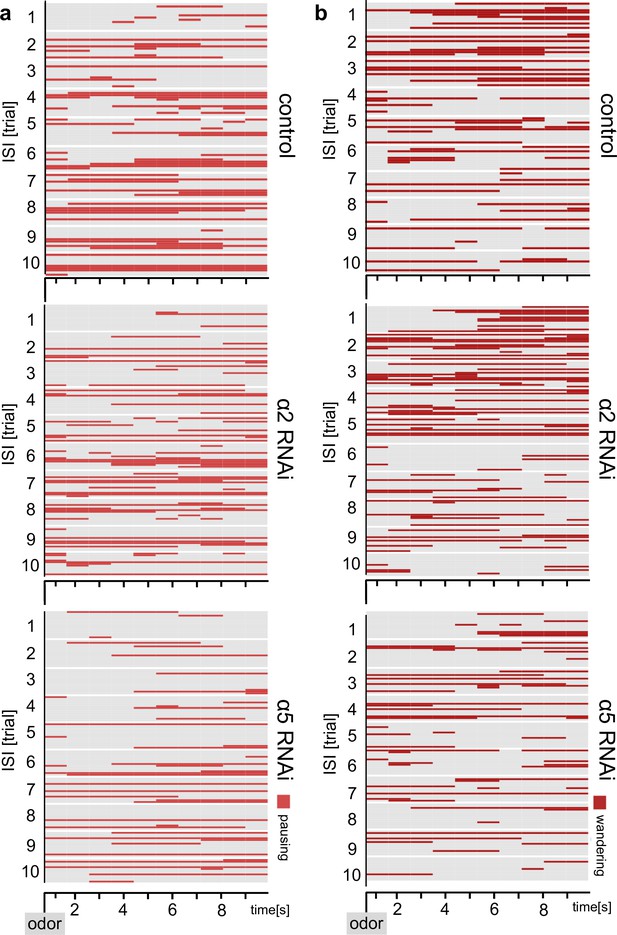
Additional ethograms, accompanying Figure 8.
(a, b) Ethograms of the behavioral responses of flies shown in Figure 8 with additional behavioral categories of pausing and wandering (when not grooming). Ethograms show pausing (red) (a), which is defined as not moving and not grooming or wandering (dark red) (b), which is defined as moving around in the chamber.
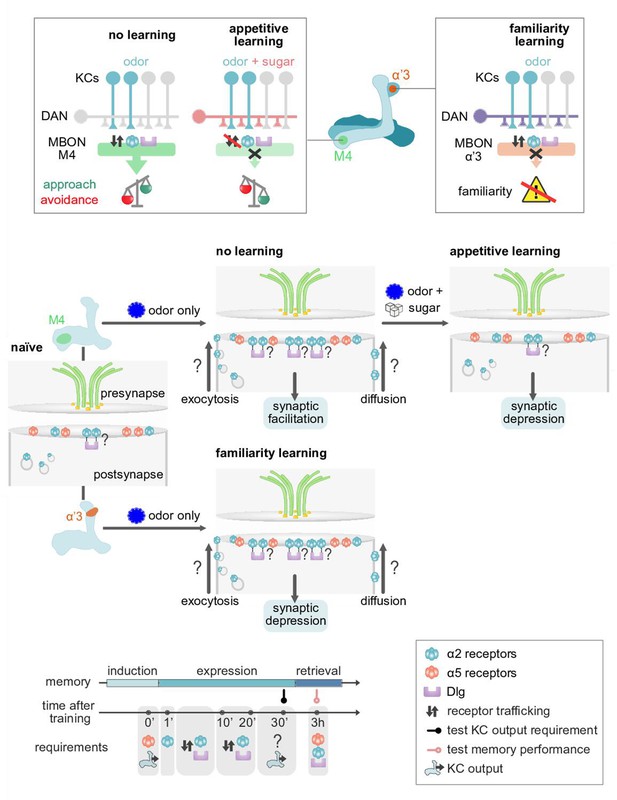
Model of postsynaptic plasticity sequence across compartments.
Top panels (circuit and behavior level): Mushroom body (MB) compartments investigated. Odors elicit high responses in M4 neurons inducing α2 receptor dynamics. High activity in M4 tilts the balance toward odor avoidance. Learning (or concurrent odor exposure with dopamine application) reduces receptor exchange. This results in reduced postsynaptic responses and tilts the balance toward approach behavior. Suppressed dynamics through learning could be reminiscent of a ‘dark current’ mechanism as found in the mammalian visual system (please see Discussion). At the level of the α’3 compartment repeated odor exposure triggers increased α2 receptor dynamics. In this compartment, the increased dynamics result in reduced odor-evoked activity in the MB output neurons (MBONs) resulting in familiarity learning (less behavioral responsiveness to familiar compared to novel odors). Middle panels (synaptic level): Our data are consistent with a model in which α5 subunit containing receptors (red) mediate the early phase of postsynaptic memory storage, potentially by leading to elevated calcium flux (not addressed in this study) at individual postsynaptic densities (see Discussion and Figure 10 showing separated PSDs (postsynaptic densities) and analyses concerning input specificity). Concurrent events see changed dynamics of the α2 receptor (blue). Changed dynamics likely reflect changed exocytosis of intracellular receptor populations or lateral diffusion across membranes. Nicotinic receptor subunits hereby potentially interact with adaptor proteins to bind to discs large (Dlg). Importantly, we identify elevated α2 subunit dynamics in the context of associative (M4; Kenyon cells [KC] and dopaminergic neuron [DAN] activation needed for memory formation) and non-associative (α’3 MBONs; odor activates both KCs and DANs) memory expression. Increased α2 subunit dynamics in both cases are triggered by odor application. At the level of M4, suppressed dynamics, would correspond to postsynaptic depression, while at the level of α’3 MBONs increased dynamics could result in postsynaptic depression. Therefore, different learning rules might govern the incorporation, exchange, or stabilization of receptors in or out of synapses. Please see Discussion for further details. Bottom panel: Proposed time line of molecular correlates and experimental read-outs of memory induction and expression (please see Discussion for details).
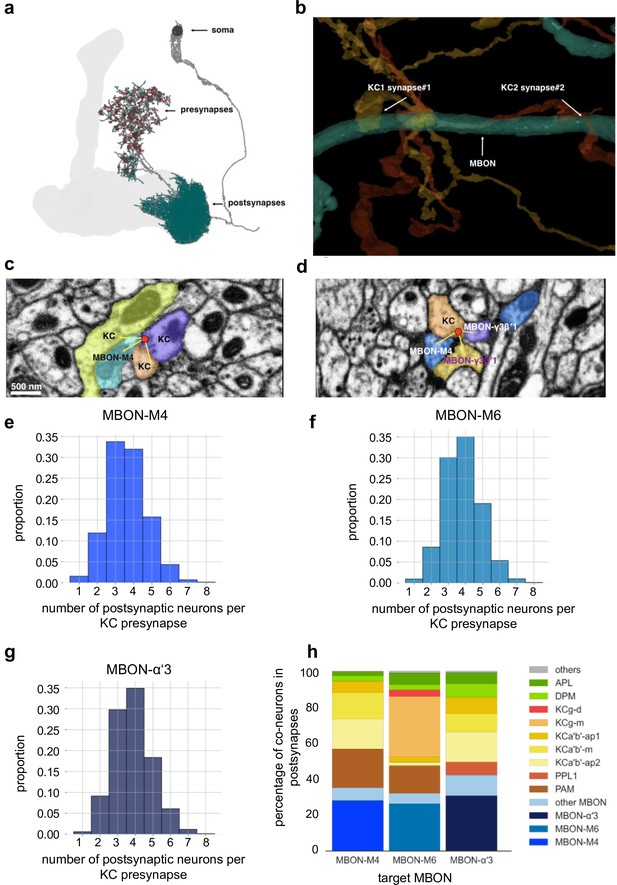
Mushroom body (MB) output connectomics (a) Example MB output neuron (MBON; here: M6) with pre- and postsynapses labeled.
(b) Reconstructed example synapses from electron microscopic (EM) volume (neuprint.org): two different Kenyon cells (KCs) connect to the same MBON on the postsynaptic side. (c) EM image (neuprint.org) showing a KC presynapse simultaneously innervating two other KCs and the M4 MBON. Scale bar: 500 nm. (d) EM image (neuprint.org) showing a KC presynapse simultaneously innervating the M4 MBON and two sites of another MBON. (e) Analysis of number of postsynaptic partners for each KC presynapse identified providing input to M4. The histogram shows the distribution of KC synapses to M4 relative to how many postsynaptic partners the KC presynapses have. (f) Analysis of number of postsynaptic partners for each KC presynapse identified providing input to M6. The histogram shows the distribution of KC synapses to M6 relative to how many postsynaptic partners the KC presynapses have. (g) Analysis of number of postsynaptic partners for each KC presynapse identified providing input to α’3 MBONs (pooled). The histogram shows the distribution of KC synapses to α’3 MBONs relative to how many postsynaptic partners the KC presynapses have. (h) Percentage of types of neurons that share a KC presynapse with a given MBON.
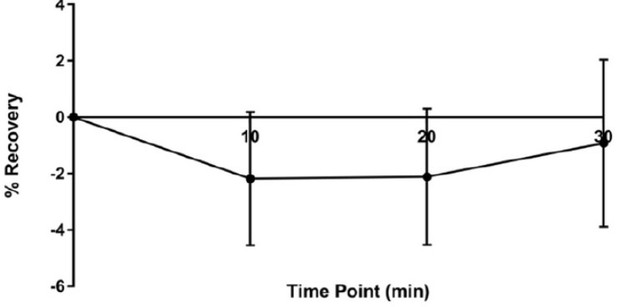
No recovery is observed after odor stimulation after bleaching of the full dendritic arbors.
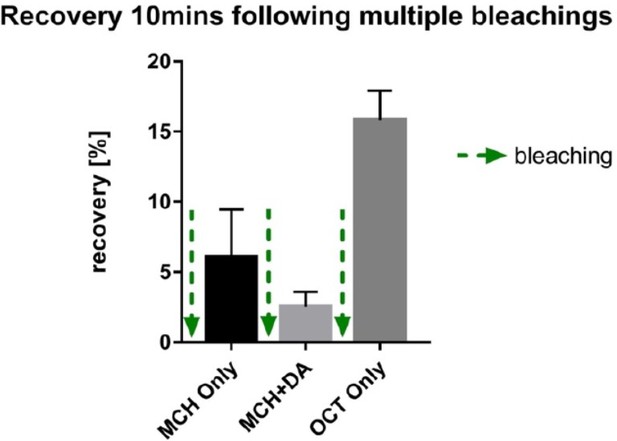
preliminary experiments demonstrating recovery of signal after three rounds of photo bleaching to a novel odor arguing against significant photo-toxic effects.
Tables
Used EGFP positions and gRNA sequences of this study.
Subunit | EGFP position between AA | gRNA sequence |
---|---|---|
nAChRalpha1/CG5610 | 438D and 439L | ACAGATCGTCGTCGGCGCCC[GGG] |
nAChRalpha2/CG6844 | 456G and 457L | CAGATTCAGCGGCTTGGTGG[GGG] |
nAChRalpha4/CG12414 | 426M and 427D | AATAGCCGCCGTCCCCGATA[TGG] |
AChRalpha5/CG32975 | 717G and 718S | CAGCACCCGAATGCCGGATG[CGG] |
nAChRalpha6/CG4128 | 403T and 404A | TTACGCCGACGAGCCAATGG[CGG] |
nAChRalpha7/CG32538 | 464G and 465S | GCAAGGGGATGACGGCAGCG[TGG] |
Additional files
-
MDAR checklist
- https://cdn.elifesciences.org/articles/80445/elife-80445-mdarchecklist1-v2.docx
-
Supplementary file 1
Supplementary statistics.
- https://cdn.elifesciences.org/articles/80445/elife-80445-supp1-v2.xlsx
-
Source data 1
Source data.
- https://cdn.elifesciences.org/articles/80445/elife-80445-data1-v2.zip