Analogue signaling of somatodendritic synaptic activity to axon enhances GABA release in young cerebellar molecular layer interneurons
Figures
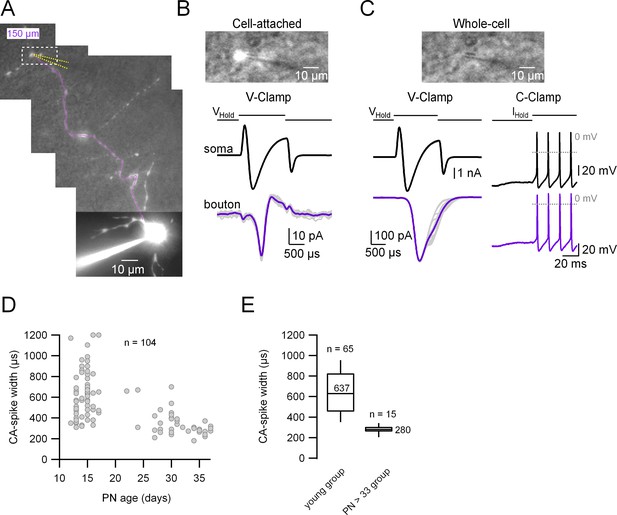
Simultaneous patch-clamp recordings from the soma and its corresponding axonal presynaptic terminal.
(A) Fluorescence image of the whole-cell recorded molecular layer interneuron (MLI). The patched presynaptic varicosity is located at 150 µm from the soma. The position of the presynaptic bouton patch pipette is represented with dotted yellow lines and the main axon in magenta. Top: Simultaneous transmitted and fluorescent light pictures of the area shown with the dotted rectangle in (A), which shows the recorded presynaptic varicosity before (B) and after (C) rupturing the seal. In (B), the fluorescence image is saturated and does not reflect the real size of the presynaptic varicosity. Bottom: Somatic (upper) and presynaptic bouton (lower), voltage- (left) or current-clamp recordings (right) of somatically induced Na+ current (or action potentials [APs]) and its propagation to the presynaptic varicosity. (D) Presynaptic AP width (measured from the cell-attached [CA] recordings) as a function of age (postnatal days 13–37). Total number of pairs = 104. (E) Box plots of the two age groups in which the recordings were categorized. Presynaptic CA-spike width of the immature age group (PN13–17): 639 ± 232 µs (median value 620 µs, n = 65). Presynaptic CA-spike width of the mature age group (PN33–37): 278 ± 44 μs (median value 280 µs, n = 15). Here, we set postnatal day 33 as the arbitrary limit between immature and mature animals.
-
Figure 1—source data 1
Data related to Figure 1D and E describing CA spike width.
- https://cdn.elifesciences.org/articles/85971/elife-85971-fig1-data1-v1.xlsx
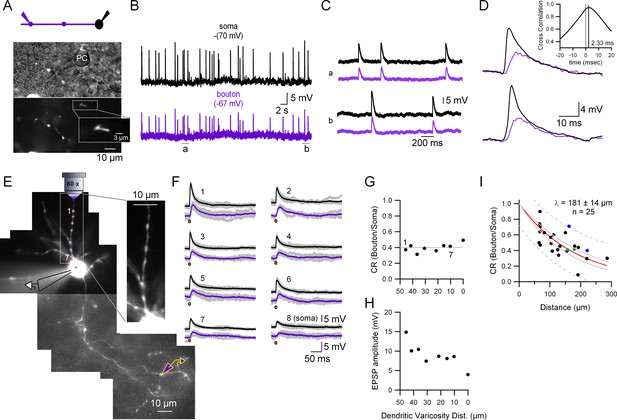
Quantification of the somato-axonal coupling for synaptic activity.
(A) Top: Recording configuration. Bottom: Pictures highlighting the position of the recorded presynaptic varicosity on top of a Purkinje cell somata during the cell-attached configuration. The inset shows an expanded view of the varicosity showing the fluorescence inside the patch pipette. (B) Current-clamp (CC) recordings of spontaneous synaptic activity in the soma and the presynaptic bouton of the molecular layer interneuron (MLI). Resting membrane potential is indicated on top of each trace. (C) Two selected time epochs are shown (a and b) in (B) with an expanded time-scale. (D) Two selected pairs of subthreshold responses are shown with a ms time-range to see the details of the responses. The inset shows the cross-correlogram of the traces shown in (B) in the −20 to +20 ms interval. (E) Fluorescent picture of an Alexa 594-filled MLI showing the experimental configuration. Glutamate was photolysed from MNI-glutamate at different dendritic varicosities (magenta spots 1–7 plus soma) while simultaneously recording from the soma and presynaptic bouton. The inset, which corresponds to a single picture, shows that all the dendritic varicosities tested are at the same depth, which ensures the same laser intensity. (F) Somatic and presynaptic bouton CC recordings of the depolarizations evoked by the photolysis of glutamate at the different dendritic varicosities (and soma) shown in (E). Brown dots indicate the timing of the laser pulse. Laser pulse duration and power are 100 µs and 2 mW, respectively. (G) Coupling ratio (CR) between the soma and presynaptic varicosities for each tested dendritic varicosity as a function of distance of the stimulated site to the soma. Dotted line is the average. (H) Somatically recorded evoked excitatory synaptic potential (eEPSP) amplitude as a function of distance. (I) EPSPs amplitude ratios as a function of distance between somatic and presynaptic bouton recording sites. The continuous red line shows the fit with an exponential function. Data include experiments with spontaneous excitatory synaptic potentials (sEPSPs) and eEPSPs. Individual points correspond to the average ratio calculated from the average of all the detected presynaptic bouton and somatic EPSPs in each cell. Circles presented with the same color (blue and green) represent data from two different varicosities on the same axon. Dotted gray lines correspond to the 95% prediction bands and solid gray lines to the 95% confidence bands. The length constant (λ) value calculated was 181 ± 14 µm (mean ± standard deviation [SD]).
-
Figure 2—source data 1
Data related to Figure 2 describing coupling between soma and axon.
- https://cdn.elifesciences.org/articles/85971/elife-85971-fig2-data1-v1.xlsx
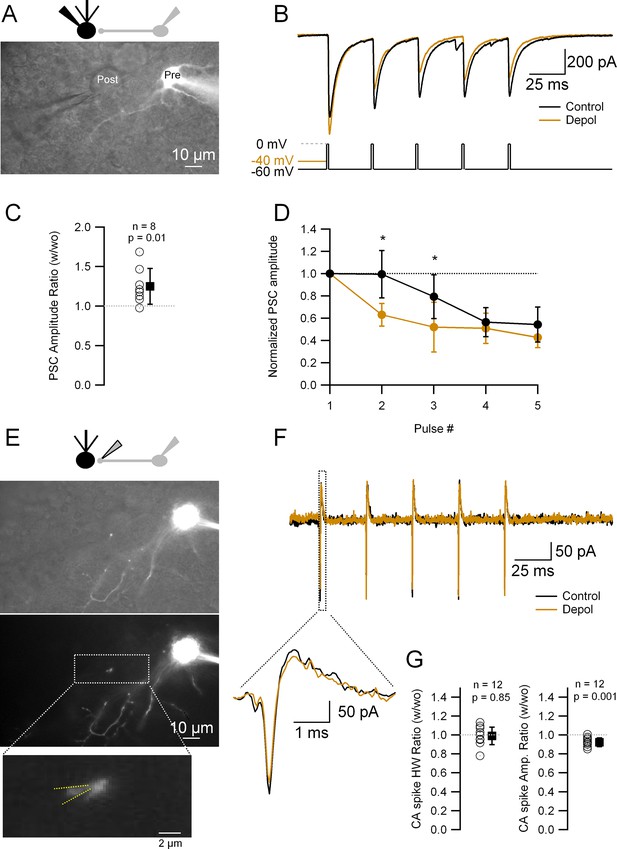
Short depolarizations increase postsynaptic current (PSC) and alter short-term plasticity but do not modify the presynaptic action potential (AP) width.
(A) Top: Recording configuration. Bottom: Superimposed transmitted and fluorescence light pictures of the synaptically connected molecular layer interneuron (MLI) and postsynaptic Purkinje cell. (B) PSCs recorded from the Purkinje cell under two different experimental conditions: control (five APs evoked in the MLI by depolarization to 0 mV from a HP of −60 mV at 33 Hz; black trace); test (same but with a 20- or 50-ms depolarization to −40 mV before the first AP; brown trace). (C) Ratio of the first PSC in test over control conditions: 1.25 ± 0.23 (mean ± standard deviation [SD], n = 8, p = 0.01). Each empty circle corresponds to a single MLI > Purkinje cell pair. Black symbol corresponds to the mean ± SD values. (D) Amplitudes of PSCs in a train normalized over the first PSC in control and test conditions. Data show the averages ± SD from seven MLI > Purkinje cell pairs. Asterisks indicate statistical significance: p value for the first interval is 0.015 and for the second interval 0.016. (E) Top: Recording configuration. Middle: Superimposed transmitted and fluorescence light pictures highlighting the MLI presynaptic varicosity on top of the Purkinje cell soma. Same pair as in A. Bottom: Fluorescence light picture of the MLI. The inset shows the details of the recorded presynaptic varicosity. Yellow dotted lines show the approximate position of the presynaptic recording pipette. (F) Presynaptic APs recorded in the cell-attached configuration from the varicosity shown in (E) under the same experimental condition as in (B). The inset shows an expanded view of the first presynaptic APs in the train. (G) Ratio of the first CA (cell-attached) recorded spikes half-widths (HW) (left) and amplitudes (right) in test over control conditions: mean width ± SD = 0.99 ± 0.09 (n = 12, p = 0.85); amplitude mean ± SD = 0.92 ± 0.06 (n = 12, p = 0.001). Each empty circle corresponds to a single presynaptic varicosity. Black symbol corresponds to the mean ± SD values.
-
Figure 3—source data 1
Data related to Figure 3 describing effects of pre-pulse depolarization on presynaptic APs and PSCs.
- https://cdn.elifesciences.org/articles/85971/elife-85971-fig3-data1-v1.xlsx
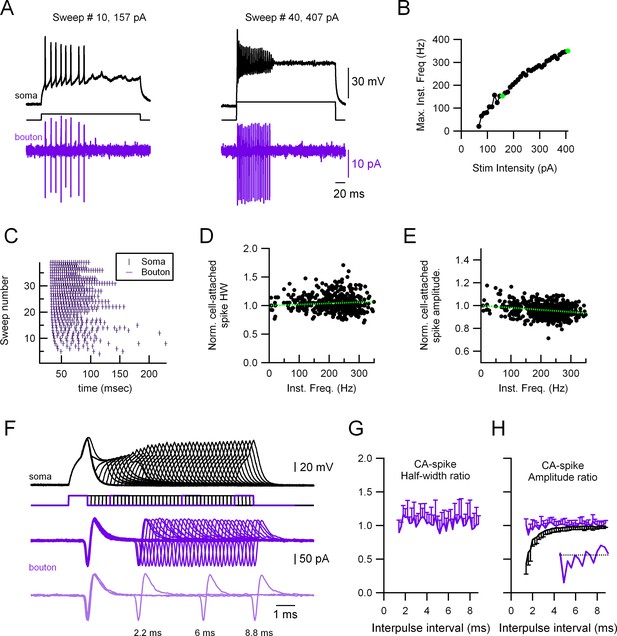
Highly reliable and stable presynaptic action potential (AP) in molecular layer interneuron (MLI) boutons.
(A) Representative experiment showing a paired recording between the soma (whole-cell current-clamp; black traces) and the presynaptic varicosity (cell-attached in voltage-clamp; magenta traces). Firing was induced by injecting current through the somatic electrode (40 trials, 67–407 pA in 10 pA increments, 300 ms duration). Left: Responses at 157 pA stimulation intensity. Right: Responses at 407 pA stimulation intensity. (B) Maximal firing frequency measured from the somatic recordings. The green dots correspond to the sweeps shown in (A). (C) Raster plots of the somatic and the presynaptic bouton spikes for the 40 different trials, showing no presynaptic AP propagation failure. Normalized presynaptic CA-spike width (D) and amplitude (E) as a function of instantaneous frequency. Green, dotted lines show linear fits to the data. Spearman rank correlation test shows no significant correlation between presynaptic AP width and firing frequency (D) and a significant negative correlation (correlation coefficient = −0.27) between the presynaptic AP amplitude and firing frequency (E). The presynaptic AP widths and amplitudes are shown normalized to the first AP in each train to avoid errors due to fluctuations between trials. (F) Representative experiment showing a paired recording between the soma (whole-cell current-clamp; black traces) and the presynaptic bouton (cell-attached in voltage-clamp; magenta traces). Firing was induced by injecting twin current pulses at varying intervals through the somatic electrode (each current pulse was 1 nA and 1 ms duration). Light magenta, lower traces, show a selection of three trials at different time intervals (8.8, 6, and 2.2 ms). Ratios (second over first axonal AP) of CA-spike half-width (G) and amplitude (H) as a function of interpulse interval. Magenta traces correspond to the presynaptic CA spikes and black trace to the somatically recorded APs (in current-clamp). Data correspond to the averages of 11 different soma–presynaptic bouton pairs. Only either the positive or negative standard deviation (SD) is shown for clarity. The inset in (H) shows the presynaptic CA spikes amplitude ratio for the intervals 1.2–4 ms.
-
Figure 4—source data 1
Excel file for data related to Figure 4 (panels B to E, G and H) describing presynaptic APs stability.
- https://cdn.elifesciences.org/articles/85971/elife-85971-fig4-data1-v1.xlsx
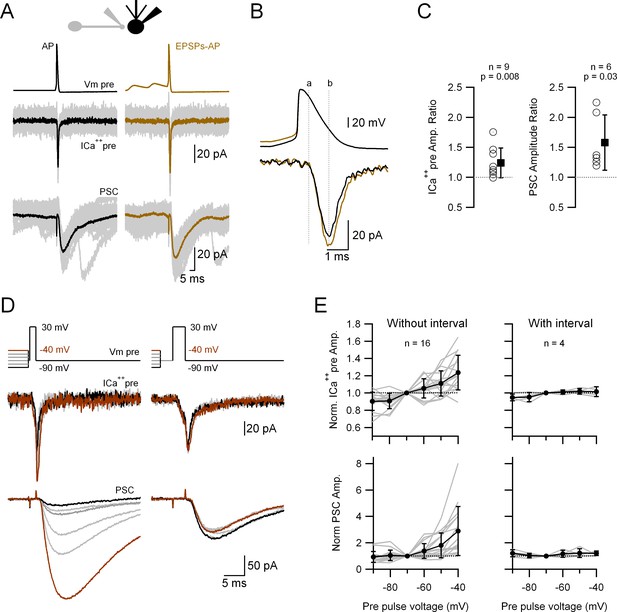
Passively propagated synaptic activity increases action potential (AP)-induced ICa++pre and postsynaptic current (PSC) in cerebellar primary cultures.
(A) Simultaneous, voltage-clamp (VC) recording of a presynaptic varicosity and the PSC. Upper traces show the voltage waveforms applied (Vm pre), middle traces the recorded ICa++pre and bottom traces the PSC. (B) Expanded AP waveforms (upper traces) and the resulting ICa++pre (bottom traces). The ‘a’ and ‘b’ dotted lines correspond to the onset and peak of the ICa++pre, respectively. (C) Relative increases (waveform with EPSPs over that without EPSPs) of the ICa++pre (left; 1.24 ± 0.25, n = 9, p = 0.008) and PSC (right; 1.58 ± 0.46, n = 6, p = 0.03) amplitudes. Each empty circle corresponds to a single varicosity (ICa++pre) or to a pair of varicosity > postsynaptic cell. Black symbol corresponds to the mean ± standard deviation (SD) values. (D) Simultaneous, VC recording of Ca++ influx into a presynaptic varicosity (middle traces) and the PSCs in its postsynaptic partner (lower traces), without (left) or with (right) a 3-ms interval between the small and large presynaptic voltage pulses. The upper traces show the voltage waveforms applied. The suprathreshold depolarizations lasted 1.5 ms (left) and 3 ms (right). (E) Normalized ICa++pre (upper graph) and PSC (lower graph) as a function of pre-pulse voltage for stimuli with (right) or without (left) a 3-ms interval between the pre-pulses and the suprathreshold depolarization. Gray lines correspond to individual varicosities and their corresponding postsynaptic cells. Black symbol corresponds to the mean ± SD values. For clarity, the complete statistical analysis of these results is presented in Figure 5—figure supplement 2.
-
Figure 5—source data 1
Data related to Figure 5A–C and E describing augmentation of ICa++ and PSCs by subthreshould depolarization.
- https://cdn.elifesciences.org/articles/85971/elife-85971-fig5-data1-v1.xlsx

Pre-pulse voltage does not influence release in the Granule cell axonal varicosities.
(A) Simultaneous, voltage-clamp (VC) recording of a presynaptic granule cell varicosity and its postsynaptic molecular layer interneuron (MLI). Upper traces show the voltage waveforms applied, middle traces the recorded ICa++pre, and bottom traces the postsynaptic current (PSC). (B) Simultaneous, VC recording of the same granule cell. Middle traces show the ICa++pre and the bottom traces the PSCs in the postsynaptic MLI. The upper traces show the voltage waveforms applied.
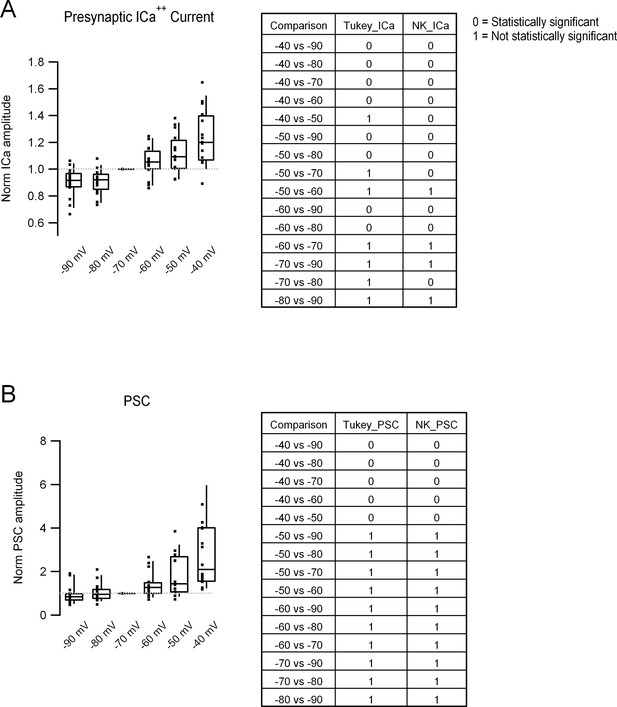
Complete statistical analysis of the data presented in Figure 5, D and E.
(A) Left: Box plots showing the ICa++pre amplitude obtained with the different pre-pulses. In each column, middle line corresponds to the median, upper, and lower horizontal lines to percentiles 75 and 25, respectively, and upper and lower extremes to percentiles 90 and 10, respectively. Filled squares correspond to individual measurements taken from single bouton > postsynaptic cell pairs. Right: Table showing the statistical comparison, performed with the pairwise comparisons Tukey, (column 2) and Newman–Keuls (column 3) tests. 0 corresponds to a statistically significant difference (rejection of the null hypothesis), and 1 to no difference (acceptance of the null hypothesis). We chose to present the results of both tests because there is no real consensus on whether to use one or the other. Please note that the results of both tests only differ in three comparisons in the case of the ICa++pre. (B) Left and right: Same analysis as in (A) but for PSC amplitude. Data correspond to the 16 different pairs shown in Figure 5D.
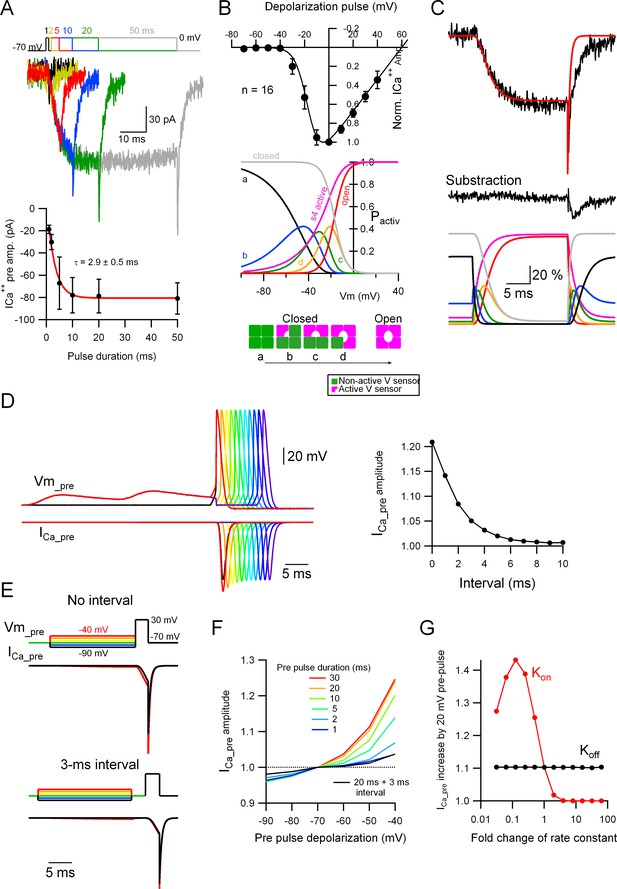
Subthreshold potential increases the probability of Cav voltage sensor activation.
(A) Top: Voltage-clamp (VC) recordings showing representative traces of ICa++pre upon depolarization to 0 mV for various durations (1, 2, 5, 10, 20, and 50 ms). Bottom: Average ICa++pre amplitude as a function of stimulus duration (mean ± SEM). Red line is the fit to the data with an exponential function. Number of presynaptic boutons analyzed: 1 ms = 4; 2 ms = 8; 5 ms = 12; 10 ms = 16; 20 ms = 16; 50 ms = 16. (B) Top: The black circles show the current to voltage (I/V) relationship of the ICa++pre (mean ± standard deviation [SD]; n = 16) and the continuous line the simulated I/V relationship, which yielded the following parameters (VCav50 = −17 mV [the half-maximal Cav voltage] and α = 0.2 [steepness of the voltage-dependent activation]). Middle: The probabilities of the different states of the Cav channels are shown as a function of voltage. Bottom: Different states of the Cav channels based on activation of independent four voltage sensors. (C) Top: Representative ICa++pre (black trace) upon a depolarization to 0 mV for 20 ms and the simulated data (red trace). Middle: Subtracted data, which indicates that the difference between the experimental and simulated data probably corresponding to a GABAA autoreceptor current. Bottom: The probabilities of the different states of the Cav channels upon the depolarizing pulse shown on the top, as a function of time. For the color code please see (B), middle and bottom schemes. (D) Left: Simulated data showing the presynaptic ICa++ induced by a single presynaptic action potential (AP) and the effect of EPSPs right before the AP. Right: Effect of the interval between the prior EPSPs and the presynaptic AP on the ICa++ pre augmentation. Please look at Figure 5A–C for a comparison with experimental data. (E) Simulated data showing the effect of a suprathreshold square depolarization on the presynaptic ICa++ pre and the effect of pre-pulse, subthreshold depolarizations to various potentials. Please look at Figure 5D, E for a comparison with experimental data. (F) Effect of the duration and interval of pre-pulse depolarizations on the presynaptic ICa++ pre augmentation. An interval between the subthreshold and suprathreshold pulses dramatically weakens the effect. (G) Effect of a change in the kon and koff rate constants on the presynaptic ICa++ enhancement induced by a 20-mV subthreshold depolarization.
-
Figure 6—source data 1
Presynaptic calcium current time constant vs recording series resistance, showing no correlation between both variables.
- https://cdn.elifesciences.org/articles/85971/elife-85971-fig6-data1-v1.xlsx
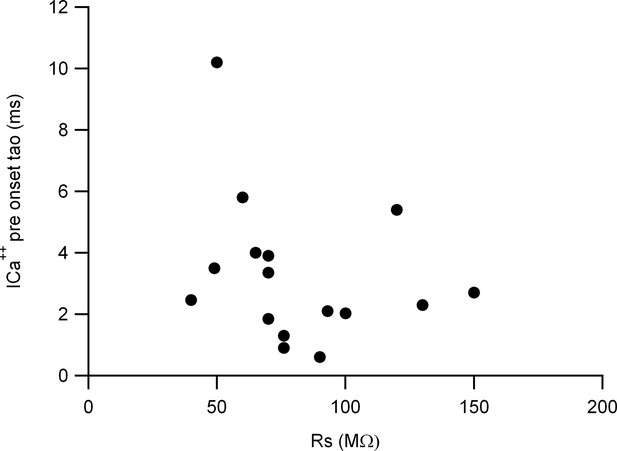
Plots for ICa++pre onset kinetics vs recording series resistance (Rs).
The ICa++pre onset time constant is shown as a function of the recording series resistance. The correlation analysis with the Spearman rank correlation test indicates no correlation between the two variables (Spearman coefficient -0.38), indicating that the Rs value does not have a significant influence on the voltage-clamp kinetics.
Tables
Reagent type (species) or resource | Designation | Source or reference | Identifiers | Additional information |
---|---|---|---|---|
Strain, strain background (Rattus norvegicus, either sex) | SD rat | Janvier Labs | RRID:RGD_38676310 | |
Strain, strain background (Rattus norvegicus, either sex) | Wistar rat | IIBCE animal facility or Japan SLC, Inc | Slc:Wistar | |
Transfected construct (adeno-associated virus) | Recombinant AAV2/9-eGFP | Kawaguchi and Sakaba, 2017 | doi: 10.1016/j.celrep.2017.11.072. | AAV vector 2/9 to transfect eGFP in neurons |
Chemical compound, drug | Tetrodotoxin Citrate | Tocris or WAKO chemical | Tocris: 1069/1 WAKO: 206-11071 | |
Chemical compound, drug | Tetraethylammonium chloride | Tocris | Tocris: 3068/50 | |
Chemical compound, drug | MNI-caged-glutamate | Tocris or HelloBio | Tocris: 1490/10 or HelloBio: HB0423 | |
Chemical compound, drug | Alexa Fluor 594 Hydrazide | Thermo Fisher | Thermo Fisher: A10438 | |
Software, algorithm | Fiji | Schindelin, J et al. https://doi.org/10.1038/nmeth.2019 | RRID:SCR_002285 | https://fiji.sc/ |
Software, algorithm | Igor Pro | WaveMetrics | RRID:SCR_000325 | https://www.wavemetrics.com/ |
Software, algorithm | Taro Tools | Labrigger, devloped by Dr. Taro Ishikawa | https://labrigger.com/blog/2011/07/21/taro-tools-and-ppt-for-igor-pro/ | https://sites.google.com/site/tarotoolsregister/ |
Software, algorithm | Python Programming Language | https://www.python.org | RRID:SCR_008394 |
Additional files
-
MDAR checklist
- https://cdn.elifesciences.org/articles/85971/elife-85971-mdarchecklist1-v1.docx
-
Source code 1
Python code for the simulation of Cav sensor activations presented in Figure 6.
- https://cdn.elifesciences.org/articles/85971/elife-85971-code1-v1.zip