A robust method for measuring aminoacylation through tRNA-Seq
Figures
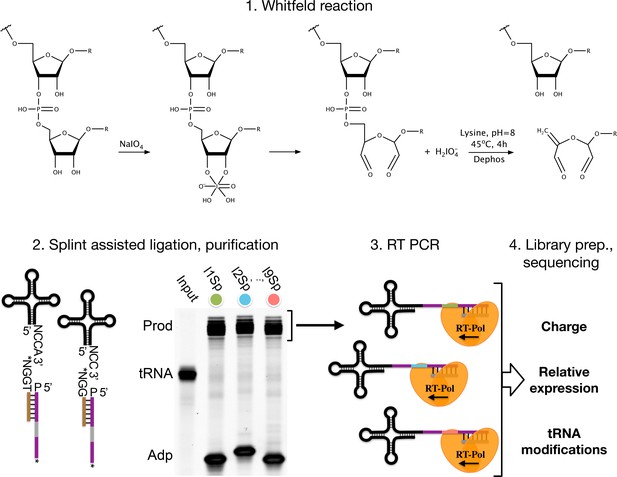
Summary illustrating the steps of the charge transfer RNA sequencing (tRNA-Seq) method we used to measure aminoacylation, relative expression, and tRNA modification levels.
First, the Whitfeld reaction (detailed in Figure 1—figure supplement 1) is used to discriminate between tRNAs with and without an aminoacylation by cleaving off the 3’ base of deacylated tRNA. Second, the tRNA secondary structure exposes the discriminator base (N) followed by the CCA/CC-end, creating a sticky end for splint-assisted ligation to a barcoded adapter. P at the 5’ end of adapter oligos indicates phosphorylation. Stars (*) on the 3’ end of splint and adapter oligos indicate modifications to block self-ligation. Third, using the purified ligation product, RT-PCR is used to generate cDNA. Fourth, the cDNA is converted into a dsDNA library and sequenced to determine tRNA charge, expression, and modifications.
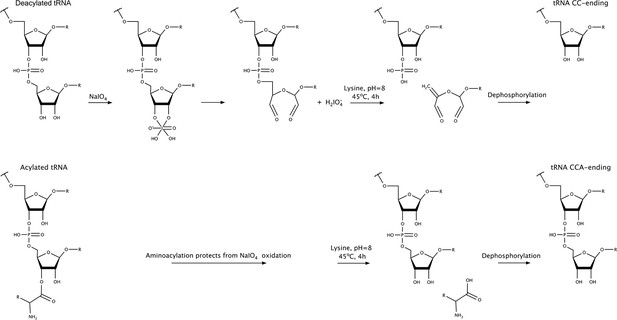
Schematic of the Whitfeld reaction with acylated and deacylated transfer RNA (tRNA) leading to generation of CCA and CC-ending tRNAs.
For deacylated tRNA, 3’ adenosine is oxidized by periodate and then cleaved off by lysine-induced β-elimination (Rammler, 1971; Uziel, 1973). Acylated tRNA is protected from periodate oxidation but will be deacylated in the subsequent incubation with lysine.
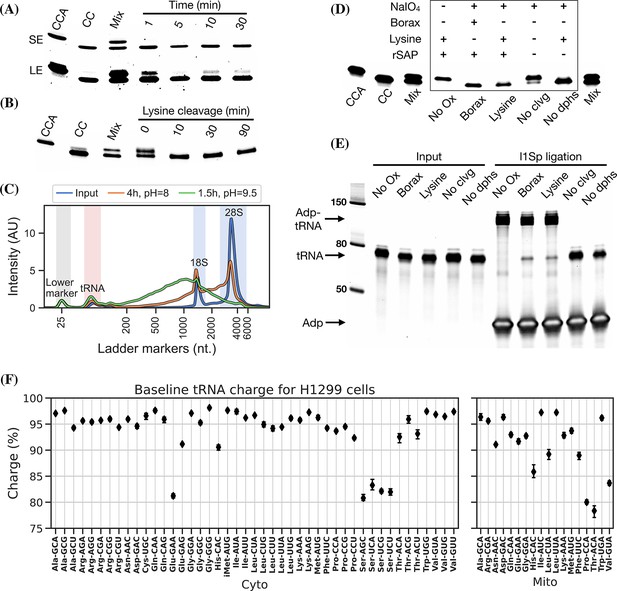
Optimizing the chemistry of charge transfer RNA sequencing (tRNA-Seq).
(A) Time required to complete periodate oxidation of the E. coli tRNA-Lys-CCA oligo on ice. Following oxidation, RNA was processed similar to Evans et al., 2017, to cleave off the 3’ adenosine. Successful cleavage produce E. coli tRNA-Lys-CC. CCA, input oligo. CC, product oligo. Mix, 50/50 mix of CCA and CC. SE, short exposure. LE, long exposure. (B) Time required to complete lysine cleavage of the E. coli tRNA-Lys-CCA oligo (CCA) at 45°C, pH = 8. Cleavage at time 0 is likely due to the heat denaturation step performed in RNA loading buffer prior to running the gel. (C) TapeStation electropherogram comparing stability of whole-cell RNA before and after 4 hr lysine cleavage at pH = 8 or 1.5 hr borax cleavage at pH = 9.5. tRNA range marked by red background, 18/28S by blue. See Figure 2—figure supplement 1, panel B for RNA stability timecourse as it occurs on a gel. (D) Effect of individual components on cleavage of the E. coli tRNA-Lys-CCA oligo (CCA). All samples were processed as a one-pot reaction, except the borax sample which was processed similar to Evans et al., 2017. rSAP, shrimp alkaline phosphatase; No Ox, no periodate oxidation; No clvg, no lysine cleavage; No dphs, no dephosphorylation. (E) Ligation test comparing the effect of RNA processing. Deacylated and gel-purified human tRNA was processed identically as in panel (D), then ligated to adapter l1Sp. Other adapters were tested with similar results (Figure 2—figure supplement 5, panel A). (F) Baseline tRNA aminoacylation charge in H1299 cells grown in Dulbecco’s Modified Eagle’s Medium (DMEM) (four replicates, bootstrapped 95% confidence interval of the mean). Only highly expressed codons are shown (more than 1000 reads per million). Charge on tRNAHis is possibly erroneously low because the discriminator base is shielded by base pairing (Heinemann et al., 2012), creating a steric hindrance for the splint-assisted ligation.
-
Figure 2—source data 1
Numeric source data for Figure 2.
- https://cdn.elifesciences.org/articles/91554/elife-91554-fig2-data1-v1.xlsx
-
Figure 2—source data 2
Original files for images in Figure 2.
- https://cdn.elifesciences.org/articles/91554/elife-91554-fig2-data2-v1.zip
-
Figure 2—source data 3
Annotated uncropped images used in Figure 2.
- https://cdn.elifesciences.org/articles/91554/elife-91554-fig2-data3-v1.docx
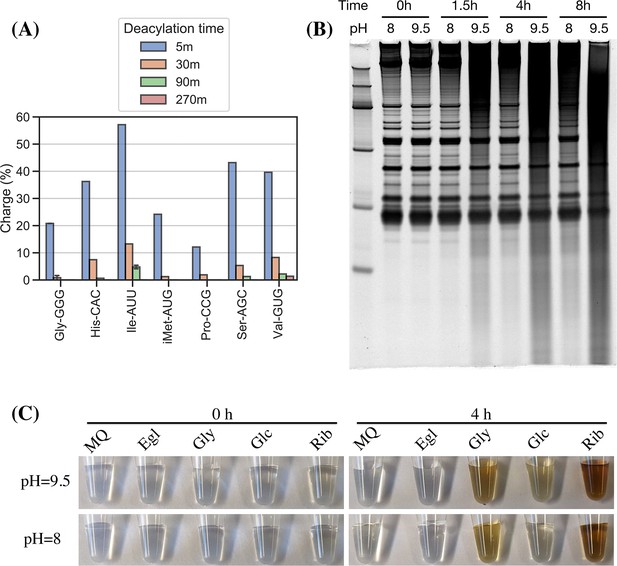
Optimizing lysine-induced cleavage for the charge transfer RNA sequencing (tRNA-Seq) method.
(A) Aminoacylation remaining after 5, 30, 90, and 270 min of deacylation in 1 M lysine pH = 8 at 45°C. After deacylation, RNA was purified and submitted to the Whitfeld reaction using lysine cleavage at pH = 9.5 for 90 min at 45°C to ensure complete deacylation. The RNA was then processed using the described charge tRNA-Seq method. (B) RNA stability over time for lysine cleavage at pH = 8 and borax cleavage at pH = 9.5. (C) Lysine reacts with dialdehydes forming from quencher oxidation. One-pot Whitfeld reactions were performed at pH = 8 and pH = 9.5 and quenched with either water (MQ), ethylene glycol (Egl), glycerol (Gly), glucose (Glc), or ribose (Rib). Pictures taken before (0 hr) and after (4 hr) the lysine cleavage step indicate side product formation consistent with lysine reacting with dialdehydes formed during the periodate quenching (Saraiva et al., 2006). This side product causes problems in the later purification step.
-
Figure 2—figure supplement 1—source data 1
Numeric source data for Figure 2—figure supplement 1.
- https://cdn.elifesciences.org/articles/91554/elife-91554-fig2-figsupp1-data1-v1.xlsx
-
Figure 2—figure supplement 1—source data 2
Original files for images in Figure 2—figure supplement 1.
- https://cdn.elifesciences.org/articles/91554/elife-91554-fig2-figsupp1-data2-v1.docx
-
Figure 2—figure supplement 1—source data 3
Annotated uncropped images used in Figure 2—figure supplement 1.
- https://cdn.elifesciences.org/articles/91554/elife-91554-fig2-figsupp1-data3-v1.zip
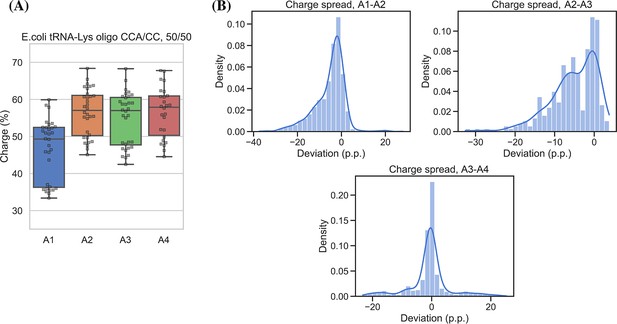
Measurement bias in charge transfer RNA sequencing (tRNA-Seq) using blunt-end ligation.
(A) Measured charge of a E. coli tRNA-Lys oligo control spiked into samples processed with four different pre-adenylated adapters. The control was made using a mix of 50% E. coli tRNA-Lys-CCA and 50% E. coli tRNA-Lys-CC and thus simulating 50% charge. Each dot represents a single charge tRNA-Seq sample. (B) Distribution of charge differences at the transcript level among samples with two barcode replicates, comparing adapters A1 vs. A2, A2 vs. A3, and A3 vs. A4. Deviation is reported as percentage point differences and the kernel density estimate (KDE) is overlaid.
-
Figure 2—figure supplement 2—source data 1
Numeric source data for Figure 2—figure supplement 2.
- https://cdn.elifesciences.org/articles/91554/elife-91554-fig2-figsupp2-data1-v1.xlsx
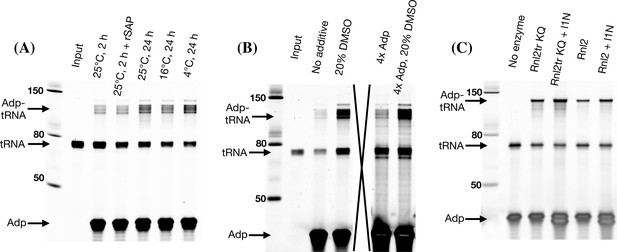
Despite optimization attempts, high ligation efficiency could not be achieved for blunt-end ligation.
(A) Effect of incubation temperature, time, and addition of a phosphatase (rSAP). Using deacylated and gel-purified human transfer RNA (tRNA) as substrate and pre-adenylated l3N as adapter, otherwise following the blunt-end ligation method described in the Materials and methods section. (B) Effect of additives and higher adapter concentration. Using deacylated and gel-purified human tRNA as substrate, pre-adenylated l2N as adapter and 4°C, 24 hr incubation. An irrelevant well has been crossed out to avoid image splicing. (C) Effect of ligase type. Using the E. coli tRNA-Lys-CCA oligo as substrate, pre-adenylated l1N as adapter and 4°C, 24 hr incubation with 20% DMSO. Wells with ‘+l1N’ were added additional none pre-adenylated adapter. Rnl2tr KQ (T4 RNA Ligase 2, truncated KQ) is the standard ligase used for pre-adenylated adapters whereas Rnl2 (T4 RNA Ligase 2) does not require pre-adenylation of adapters.
-
Figure 2—figure supplement 3—source data 1
Original files for images in Figure 2—figure supplement 3.
- https://cdn.elifesciences.org/articles/91554/elife-91554-fig2-figsupp3-data1-v1.zip
-
Figure 2—figure supplement 3—source data 2
Annotated uncropped images used in Figure 2—figure supplement 3.
- https://cdn.elifesciences.org/articles/91554/elife-91554-fig2-figsupp3-data2-v1.docx
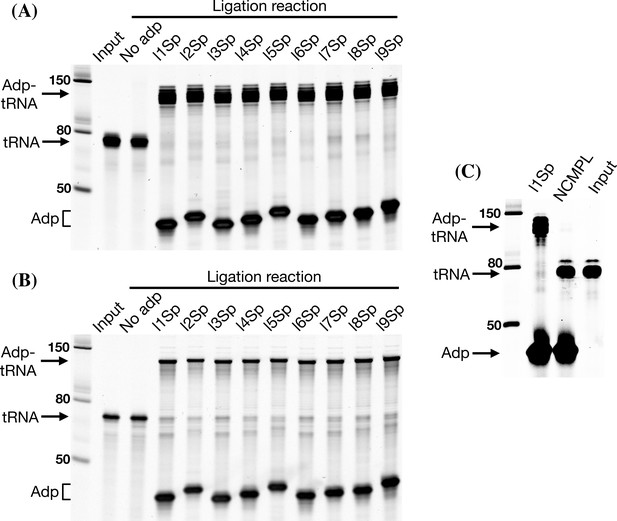
Ligation efficiency of all the barcoded adapters is high and depends on splint complementarity.
(A) Ligation reactions using deacylated purified human transfer RNA (tRNA) as substrate. (B) Ligation reactions using E. coli tRNA-Lys-CC oligo as substrate. (C) Comparing ligation using a tRNA-end complementary splint (l1Sp lane) vs. a non-complementary splint (NCMPL lane). For both ligations the l1Sp adapter was used. For the non-complementary splint ligation the two standard TGGN and GGN overhang generating splints were swapped by two splints generating CAAC and AAC overhangs.
-
Figure 2—figure supplement 4—source data 1
Original files for images in Figure 2—figure supplement 4.
- https://cdn.elifesciences.org/articles/91554/elife-91554-fig2-figsupp4-data1-v1.zip
-
Figure 2—figure supplement 4—source data 2
Annotated uncropped images used in Figure 2—figure supplement 4.
- https://cdn.elifesciences.org/articles/91554/elife-91554-fig2-figsupp4-data2-v1.docx
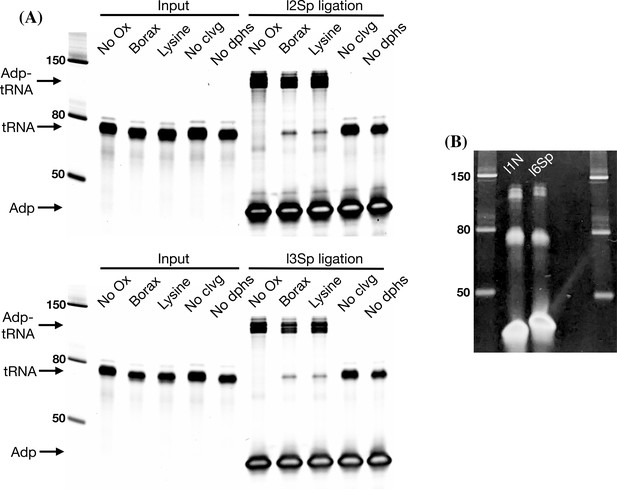
Ligation test comparing the effect of RNA processing.
(A) Ligation test comparing the effect of RNA processing. Similar to Figure 2, panel E but with two different adapters. (B) The unligated transfer RNA (tRNA) that appears after tRNA is oxidized with periodate in panel A is refractory to further ligation. The unligated tRNA was gel purified from enough ligation reactions as shown in panel A to set up two new ligation reactions using either l1N pre-adenylated adapter for blunt-end ligation or l6Sp for splint-assisted ligation. For l1N, ligation was set up with 35 ng tRNA, 20 pmol adapter, 17.5% PEG-8000, 20% DMSO, 1xT4 RNA ligase buffer, 1 μL T4 RNA ligase 2 (truncated KQ), and 1 μL SuperaseIn. For l6Sp, the ligation was set up as described in the charge tRNA sequencing (tRNA-Seq) protocol.
-
Figure 2—figure supplement 5—source data 1
Original files for images in Figure 2—figure supplement 5.
- https://cdn.elifesciences.org/articles/91554/elife-91554-fig2-figsupp5-data1-v1.zip
-
Figure 2—figure supplement 5—source data 2
Annotated uncropped images used in Figure 2—figure supplement 5.
- https://cdn.elifesciences.org/articles/91554/elife-91554-fig2-figsupp5-data2-v1.docx
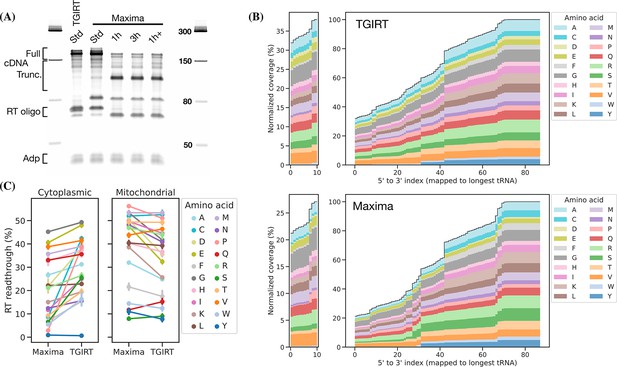
RT readthrough comparing TGIRT to Maxima.
(A) The Maxima reverse transcription (RT) polymerase produces similar levels of full-size cDNA as TGIRT-III under the standard (Std) transfer RNA sequencing (tRNA-Seq) RT-PCR conditions (42°C, 16 hr, suggested by Behrens et al., 2021). For Maxima, other incubation conditions tested are: 1 hr at 60°C (similar to Lucas et al., 2024), 3 hr at 60°C and 1 hr at 60°C followed by 15 hr at 42°C (1h+). After RT-PCR, the RNA template was removed by NaOH hydrolysis, liberating the DNA adapter annotated on the gel. (B) Coverage plots for cytoplasmic tRNA transcripts grouped by cognate amino acid, comparing samples prepared with TGIRT-III or Maxima using standard incubation (42°C, 16 hr). (C) Percentage of full-length transcripts grouped by cognate amino acid (i.e. left side of plots in panel B). Error bars are bootstrapped 95% confidence interval of the mean over the seven individual samples, barcoded, pooled, and used for RT-PCR template with both TGIRT-III and Maxima.
-
Figure 2—figure supplement 6—source data 1
Numeric source data for Figure 2—figure supplement 6.
- https://cdn.elifesciences.org/articles/91554/elife-91554-fig2-figsupp6-data1-v1.xlsx
-
Figure 2—figure supplement 6—source data 2
Original files for images in Figure 2—figure supplement 6.
- https://cdn.elifesciences.org/articles/91554/elife-91554-fig2-figsupp6-data2-v1.zip
-
Figure 2—figure supplement 6—source data 3
Annotated uncropped images used in Figure 2—figure supplement 6.
- https://cdn.elifesciences.org/articles/91554/elife-91554-fig2-figsupp6-data3-v1.docx
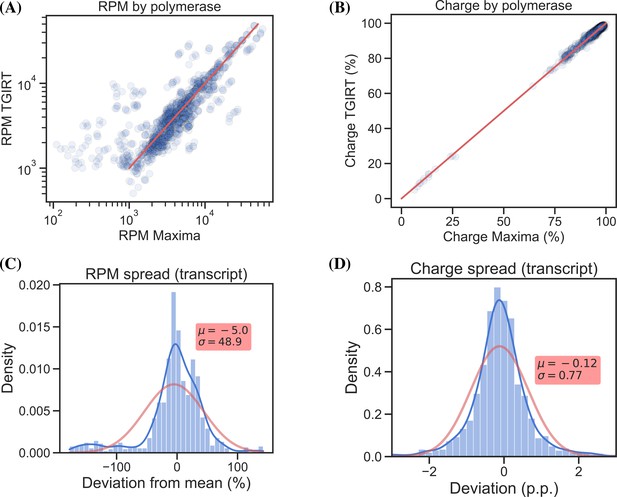
Transfer RNA (tRNA) expression level measurements are sensitive to the choice of reverse transcription (RT) polymerase while charge measurements are not.
(A) Comparing relative tRNA expression of TGIRT vs. Maxima. (B) Comparing tRNA charge of TGIRT vs. Maxima. (C) Density plot showing the percentage deviation of reads per million (RPM) measurements using TGIRT vs. Maxima, compared to the average of both. (D) Density plot showing the percentage point deviation of charge measurements using TGIRT vs. Maxima, compared to the average of both. Data is shown at transcript level for seven individual RNA samples, barcoded, pooled, and used for RT-PCR template with both TGIRT-III and Maxima. The red line in (A) and (B) is equality. Density plots are provided with kernel density estimate (KDE) in blue, normal distribution estimate in red and inserts with mean (μ) and standard deviation (σ). For density plots, a negative deviation is the result of RPM or charge readings being higher for TGIRT than for Maxima, and vice versa for positive deviations.
-
Figure 2—figure supplement 7—source data 1
Numeric source data for Figure 2—figure supplement 7.
- https://cdn.elifesciences.org/articles/91554/elife-91554-fig2-figsupp7-data1-v1.xlsx
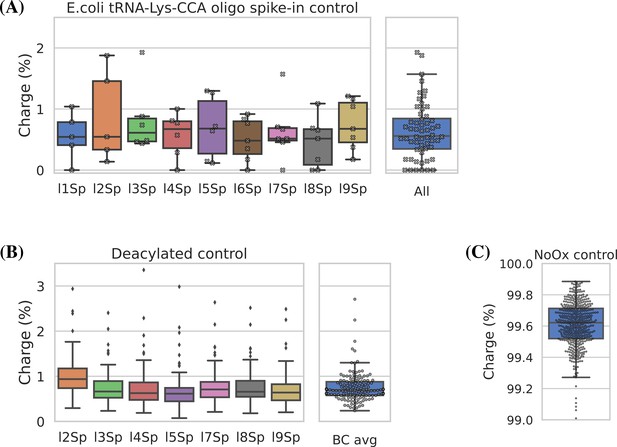
Charge transfer RNA sequencing (tRNA-Seq) control samples and spike-ins validate the method.
(A) Cleavage of the 3’ adenosine on spike-in oligo is near complete and similarly measured across adapters. Using the E. coli tRNA-Lys-CCA oligo as a spike-in control to monitor completion of the Whitfeld reaction. If complete, 100% E. coli tRNA-Lys-CC should be produced and thus appearing as 0% charged. Each dot represents one sample spiked with E. coli tRNA-Lys-CCA oligo before the Whitfeld reaction and processed using the charge tRNA-Seq processing described in the Materials and methods section. (B) Aminoacylation level of human tRNA transcripts after undergoing deacylation by incubation at 45°C for 4 hr in 1 M lysine (pH = 8). Mitochondrial tRNAfMet was excluded because formylated amino acids are known to be highly resistant toward deacylation (Schofield and Zamecnik, 1968). (C) Aminoacylation level of tRNA transcripts from four samples receiving sham oxidation (NaCl) during the Whitfeld reaction.
-
Figure 2—figure supplement 8—source data 1
Numeric source data for Figure 2—figure supplement 8.
- https://cdn.elifesciences.org/articles/91554/elife-91554-fig2-figsupp8-data1-v1.xlsx
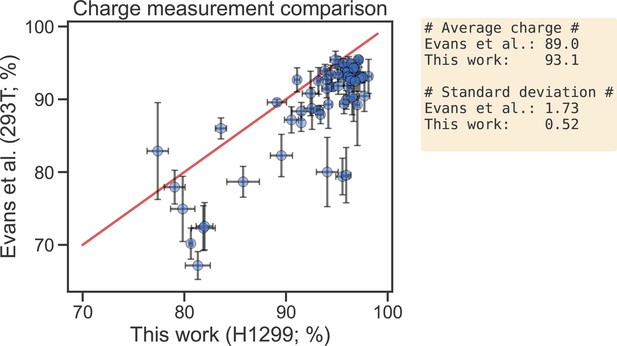
Charge measurement comparison between H1299 at baseline (this work) and 293T at baseline (Evans et al., 2017).
Each point is an isodecoder shared by both datasets, error bars are ±1 standard deviation of the sample replicates, and the red line is equality. H1299 charge measurements with four replicates also displayed in Figure 2, panel F. 293T charge measurement with six replicates, from NCBI Geo database (accession GSE97259, supplementary file). In right text box, the average charge and the average replicate standard deviation, across all isodecoders.
-
Figure 2—figure supplement 9—source data 1
Numeric source data for Figure 2—figure supplement 9.
- https://cdn.elifesciences.org/articles/91554/elife-91554-fig2-figsupp9-data1-v1.xlsx
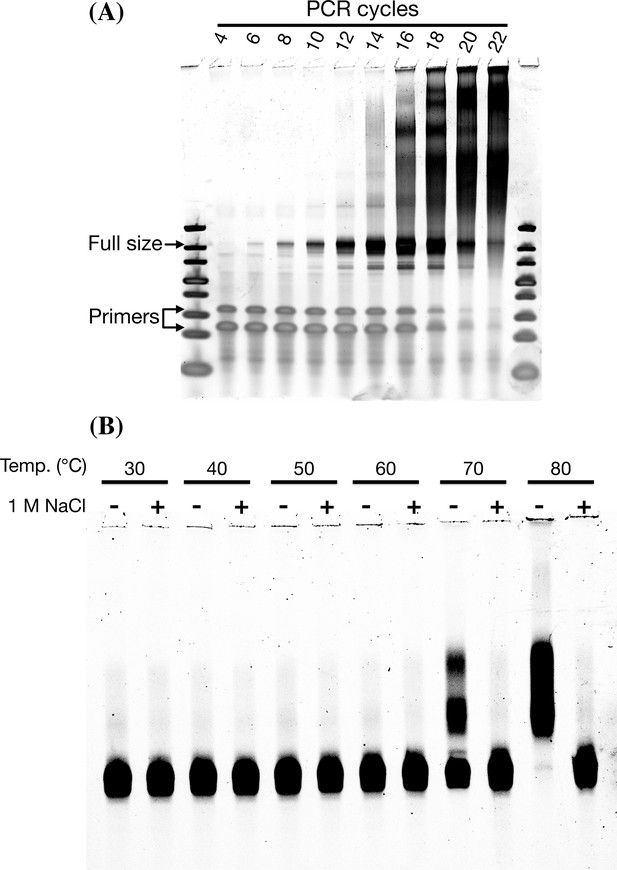
tRNA homology requires careful PCR conditions.
(A) The specificity of the final library PCR step (attaching Illumina P7 and P5 sequences) deteriorates with increasing product-to-primer ratios, probably due to high transfer RNA (tRNA) homology and PCR crossover (Holcomb et al., 2014). (B) tRNA sequencing (tRNA-Seq) DNA library reannealing is inhibited by high salt concentrations. A gel-purified charge tRNA-Seq DNA library was resuspended in TBE buffer and incubated 30 min at different temperatures with or without 1 M NaCl.
-
Figure 2—figure supplement 10—source data 1
Original files for images in Figure 2—figure supplement 10.
- https://cdn.elifesciences.org/articles/91554/elife-91554-fig2-figsupp10-data1-v1.zip
-
Figure 2—figure supplement 10—source data 2
Annotated uncropped images used in Figure 2—figure supplement 10.
- https://cdn.elifesciences.org/articles/91554/elife-91554-fig2-figsupp10-data2-v1.docx
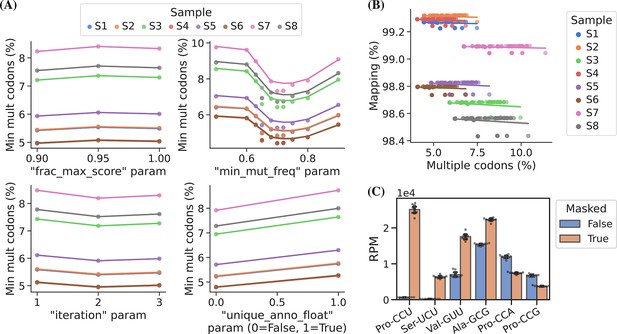
Masking of the reference sequences improves alignment performance.
(A) Grid search optimization of parameters determining the extent of reference masking (see Materials and methods section for details). Each subplot shows the mean effect of one tuning parameter when combined with the combination of all the other three. Parameters used for reference masking are chosen to minimize the percentage of reads assigned to transfer RNAs (tRNAs) with multiple codons. (B) There is no trade-off between sequence mapping success and minimizing multiple codon mapping. (C) Reference masking increases relative expression levels of select codons. Reads per million (RPM) levels of the codons shown were found before and after optimized reference masking. Error bars are bootstrapped 95% confidence interval of the mean over the nine barcode replicate samples.
-
Figure 3—source data 1
Numeric source data for Figure 3.
- https://cdn.elifesciences.org/articles/91554/elife-91554-fig3-data1-v1.xlsx
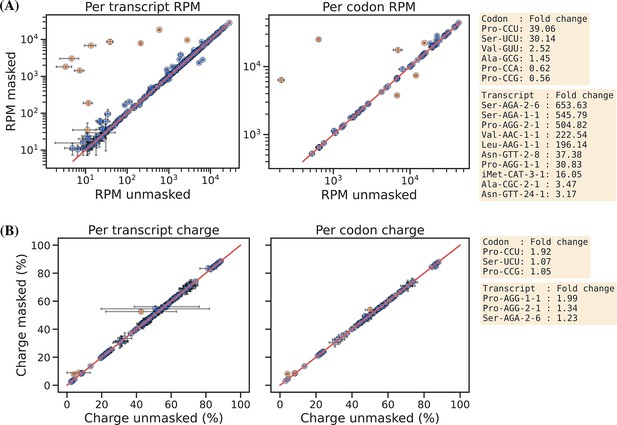
Reference masking effect on RPM and charge levels.
(A) Reference masking effect on reads per million (RPM) levels per transcript (left) and per codon (right). Transcripts showing >3, and codons showing >1.4, fold increase or decrease upon reference masking are colored orange and annotated on the right side of the plot. (B) Reference masking effect on charge levels per transcript (left) and per codon (right). Transcripts/codons showing >1.05-fold increase or decrease upon reference masking are colored orange and annotated on the right side of the plot.
-
Figure 3—figure supplement 1—source data 1
Numeric source data for Figure 3—figure supplement 1.
- https://cdn.elifesciences.org/articles/91554/elife-91554-fig3-figsupp1-data1-v1.xlsx
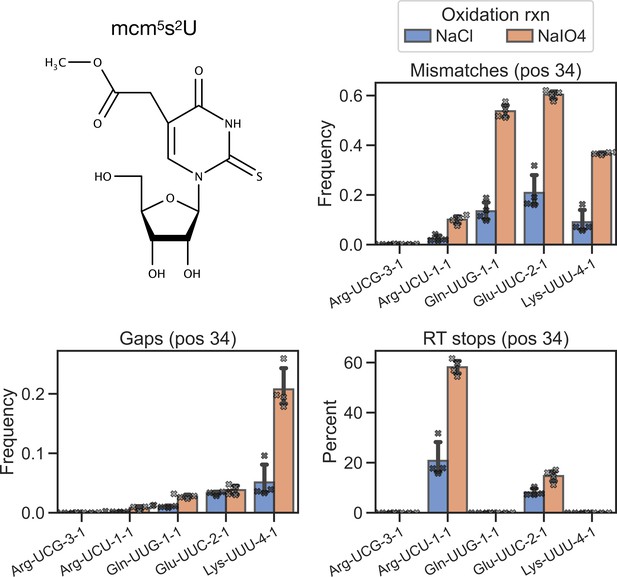
Mismatch frequency, gap frequency, and reverse transcription (RT) stop percentage is increased upon periodate oxidation for transcripts known to be 5-methoxycarbonylmethyl-2-thiouridine (mcm5s2U) modified.
The mcm5s2U modification has been shown to be present on the first anticodon nucleoside (position 34) in human transfer RNA (tRNA) Lys-UUU, Gln-UUG, Glu-UUC, and Arg-UCU, while absent in the similar tRNA Arg-UCG (Lentini et al., 2018).
-
Figure 3—figure supplement 2—source data 1
Numeric source data for Figure 3—figure supplement 2.
- https://cdn.elifesciences.org/articles/91554/elife-91554-fig3-figsupp2-data1-v1.xlsx
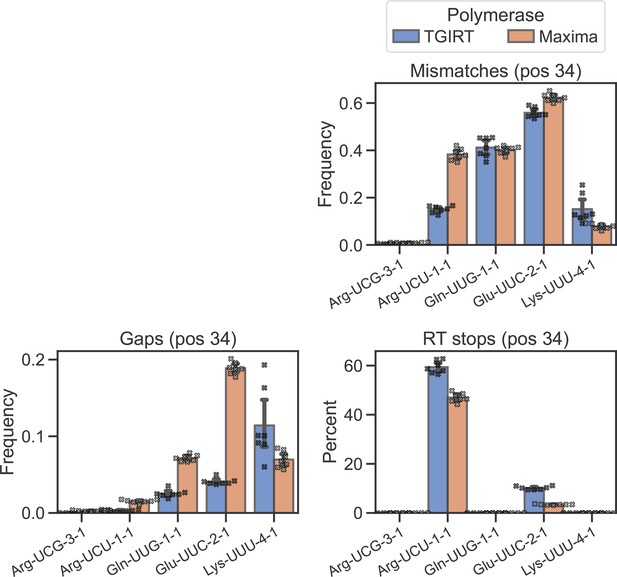
Polymerase-dependent mismatch frequency, gap frequency, and reverse transcription (RT) stop percentage for the 5-methoxycarbonylmethyl-2-thiouridine (mcm5s2U) modified position.
Comparing TGIRT vs. Maxima RT polymerases, otherwise similar to Figure 3—figure supplement 2. Data is shown for seven individual RNA samples that were submitted to the Whitfeld reaction, barcoded, pooled, and used for RT-PCR template with both TGIRT-III and Maxima.
-
Figure 3—figure supplement 3—source data 1
Numeric source data for Figure 3—figure supplement 3.
- https://cdn.elifesciences.org/articles/91554/elife-91554-fig3-figsupp3-data1-v1.xlsx
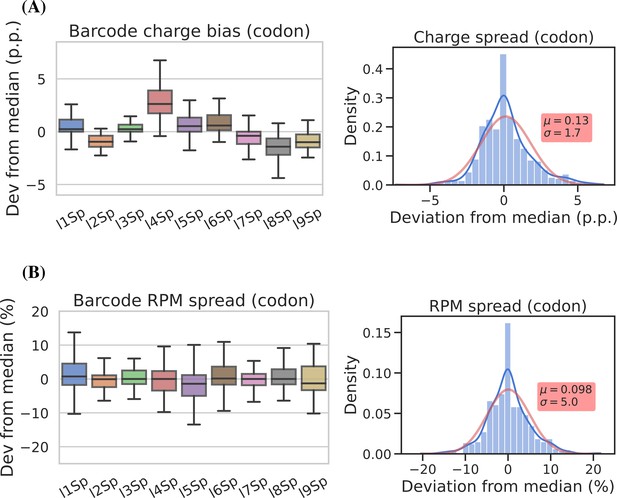
Barcode replicates show high precision and limited barcode bias.
Each of the nine adapters was ligated to the same sample containing a heterogeneous mix of CC and CCA-ending transfer RNAs (tRNAs). Ligations were then pooled and submitted to the remainder of the charge tRNA sequencing (tRNA-Seq) protocol. (A) The percentage point deviation from the median charge at the codon level, grouped by barcode identity (left) or shown summarized as a density plot (right). (B) The percentage deviation from the median reads per million (RPM) at the codon level, grouped by barcode identity (left) or shown summarized as a density plot (right). Density plots are provided with kernel density estimate (KDE) in blue, normal distribution estimate in red and inserts with mean (μ) and standard deviation (σ). For plots of transcript level data, see Figure 4—figure supplement 2.
-
Figure 4—source data 1
Numeric source data for Figure 4.
- https://cdn.elifesciences.org/articles/91554/elife-91554-fig4-data1-v1.xlsx
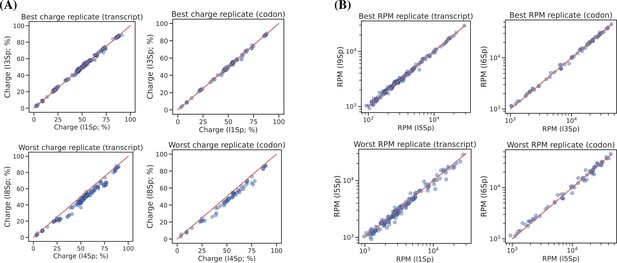
Best and worst pairwise comparisons between barcode replicates.
Sorting pairwise comparisons between barcode replicates according to the sum of squared differences and showing the best and worst either at the transcript or codon level. (A) For charge levels, adapter l4Sp tends to overestimate charge. (B) For reads per million (RPM) levels. For all plots the red line is equality.
-
Figure 4—figure supplement 1—source data 1
Numeric source data for Figure 4—figure supplement 1.
- https://cdn.elifesciences.org/articles/91554/elife-91554-fig4-figsupp1-data1-v1.xlsx
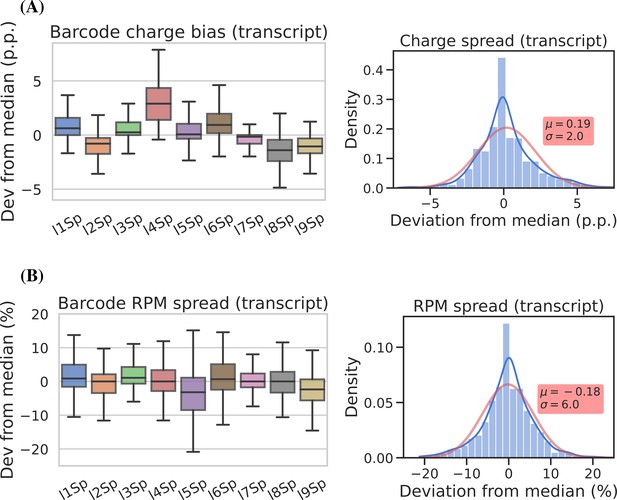
Similar to Figure 4, but at the transcript level.
-
Figure 4—figure supplement 2—source data 1
Numeric source data for Figure 4—figure supplement 2.
- https://cdn.elifesciences.org/articles/91554/elife-91554-fig4-figsupp2-data1-v1.xlsx
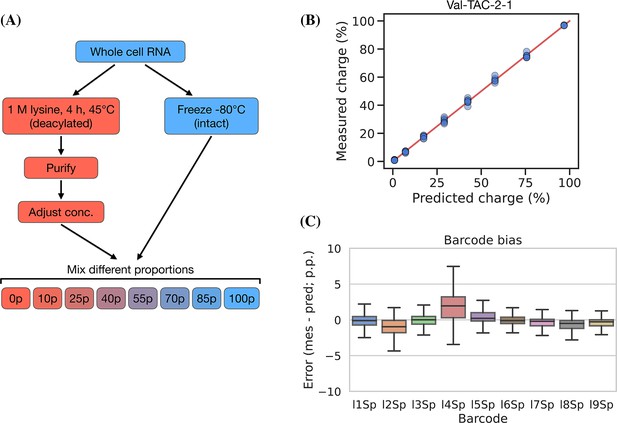
Charge titration shows linearity over the full range of charge measurements.
(A) Schematic illustration of the method to generate samples with predictable charge percentages. (B) Titration data for a representative transfer RNA (tRNA) transcript, Val-TAC-2-1, with the red line indicating equality between predicted and measured charge. For reference, the best and worst fitting tRNA transcripts are shown in Figure 5—figure supplement 1. (C) Error binned by adapter barcode. Error is the percentage point difference between the measured vs. predicted charge for all transcripts in the bin.
-
Figure 5—source data 1
Numeric source data for Figure 5.
- https://cdn.elifesciences.org/articles/91554/elife-91554-fig5-data1-v1.xlsx
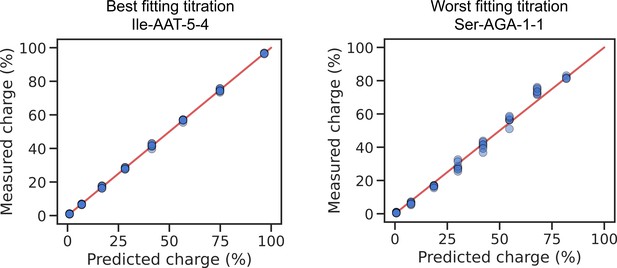
The best and worst transcript when ranked based on the sum of squared differences between the measured and predicted charge.
Related to the representative (i.e. ranked as the median) transcript shown in Figure 5, panel B.
-
Figure 5—figure supplement 1—source data 1
Numeric source data for Figure 5—figure supplement 1.
- https://cdn.elifesciences.org/articles/91554/elife-91554-fig5-figsupp1-data1-v1.xlsx
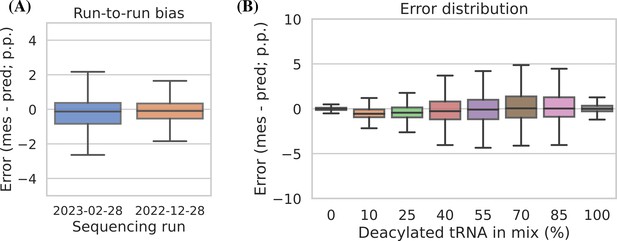
Charge titration prediction error binned by sequencing run and titration sample.
(A) Run-to-run bias of two sequencing libraries independently prepared and sequenced on different days. (B) Error distribution binned by titration sample. In both panels, error is the percentage point difference between the measured vs. predicted charge for all transcripts in the bin.
-
Figure 5—figure supplement 2—source data 1
Numeric source data for Figure 5—figure supplement 2.
- https://cdn.elifesciences.org/articles/91554/elife-91554-fig5-figsupp2-data1-v1.xlsx
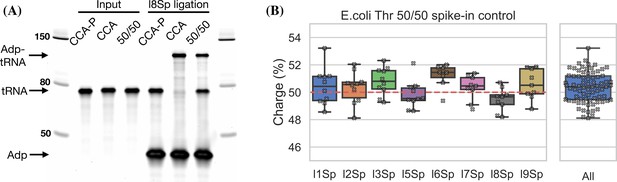
Spike-in control for 50% charge using the Escherichia coli tRNA-Thr-CGT oligo.
(A) Ligation between E. coli tRNA-Thr-CCA-Phos and l8Sp is completely blocked indicating ~100% 3’ phosphorylation. CCA-P, E. coli tRNA-Thr-CCA-Phos. CCA, E. coli tRNA-Thr-CCA. 50/50, equal mix of CCA-p and CCA. (B) E. coli tRNA-Thr spike-in charge measured for samples prepared with an equimolar mix of E. coli tRNA-Thr-CCA-Phos and E. coli tRNA-Thr-CCA. Each dot represents a single charge transfer RNA sequencing (tRNA-Seq) sample. The red dashed line indicates 50% charge.
-
Figure 5—figure supplement 3—source data 1
Numeric source data for Figure 5—figure supplement 3.
- https://cdn.elifesciences.org/articles/91554/elife-91554-fig5-figsupp3-data1-v1.xlsx
-
Figure 5—figure supplement 3—source data 2
Original files for images in Figure 5—figure supplement 3.
- https://cdn.elifesciences.org/articles/91554/elife-91554-fig5-figsupp3-data2-v1.zip
-
Figure 5—figure supplement 3—source data 3
Annotated uncropped images used in Figure 5—figure supplement 3.
- https://cdn.elifesciences.org/articles/91554/elife-91554-fig5-figsupp3-data3-v1.docx
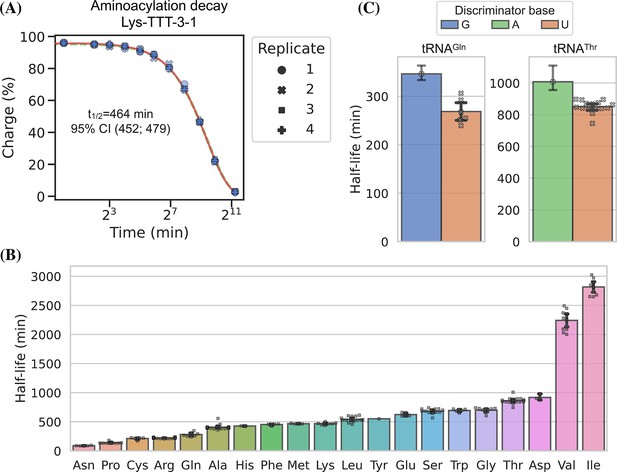
Measuring aminoacylation half-life using charge transfer RNA sequencing (tRNA-Seq).
(A) Aminoacylation decay for a representative tRNA transcript Lys-TTT-3-1 over the 11 timepoints sampled. For reference, the best and worst fitting tRNA transcripts are shown in Figure 6—figure supplement 2. The fitted first-order decay to estimate the aminoacylation half-life is shown as a red line. Similar dashed lines are plotted in green for the bootstrapped 95% confidence interval (these are hard to see). (B) Aminoacylation half-life estimates grouped by amino acid. Each marker represents one transcript, error bars are bootstrapped 95% confidence intervals of the mean. (C) Distribution of aminoacylation half-life estimates for tRNAGln and tRNAThr transcripts grouped by discriminator base identity. Error bars are bootstrapped 95% confidence intervals. For the single transcripts with G or A discriminator base the bootstrap is performed on measurement replicates while for the U discriminator base it is performed on the transcript observations.
-
Figure 6—source data 1
Numeric source data for Figure 6.
- https://cdn.elifesciences.org/articles/91554/elife-91554-fig6-data1-v1.xlsx
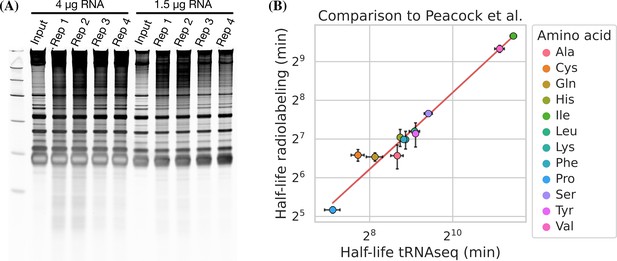
RNA integrity and comparison to previous half-life values.
(A) RNA integrity after the last sample was taken (40 hr) for the four replicates in the aminoacylation half-life experiment. (B) Comparison between aminoacylation half-life estimates grouped by amino acid from this study (transfer RNA sequencing [tRNA-Seq]) and measurements by Peacock et al., 2014 (radiolabeling). Error bars are ± standard deviations. A linear regression line is shown as a red line.
-
Figure 6—figure supplement 1—source data 1
Numeric source data for Figure 6—figure supplement 1.
- https://cdn.elifesciences.org/articles/91554/elife-91554-fig6-figsupp1-data1-v1.xlsx
-
Figure 6—figure supplement 1—source data 2
Original files for images in Figure 6—figure supplement 1.
- https://cdn.elifesciences.org/articles/91554/elife-91554-fig6-figsupp1-data2-v1.zip
-
Figure 6—figure supplement 1—source data 3
Annotated uncropped images used in Figure 6—figure supplement 1.
- https://cdn.elifesciences.org/articles/91554/elife-91554-fig6-figsupp1-data3-v1.docx
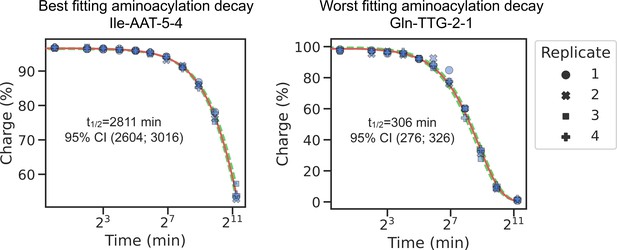
The best and worst transcript half-life estimates, ranked based on the sum of squared differences between the fitted decay function and the mean charge of the replicates.
Related to the representative (i.e. ranked as the median) transcript shown in Figure 6, panel A.
-
Figure 6—figure supplement 2—source data 1
Numeric source data for Figure 6—figure supplement 2.
- https://cdn.elifesciences.org/articles/91554/elife-91554-fig6-figsupp2-data1-v1.xlsx
Additional files
-
Supplementary file 1
Oligo and reagents list.
- https://cdn.elifesciences.org/articles/91554/elife-91554-supp1-v1.xlsx
-
Supplementary file 2
Step-by-step transfer RNA sequencing (tRNA-Seq) protocol.
- https://cdn.elifesciences.org/articles/91554/elife-91554-supp2-v1.docx
-
Supplementary file 3
Overview of the RNA/DNA manipulation steps.
- https://cdn.elifesciences.org/articles/91554/elife-91554-supp3-v1.xlsx
-
Supplementary file 4
TGIRT versus Maxima read mapping statistics.
- https://cdn.elifesciences.org/articles/91554/elife-91554-supp4-v1.xlsx
-
Supplementary file 5
Transfer RNA (tRNA) aminoacylation half-life fitting data.
- https://cdn.elifesciences.org/articles/91554/elife-91554-supp5-v1.xlsx
-
MDAR checklist
- https://cdn.elifesciences.org/articles/91554/elife-91554-mdarchecklist1-v1.pdf