Balance of activity during a critical period tunes a developing network
Figures
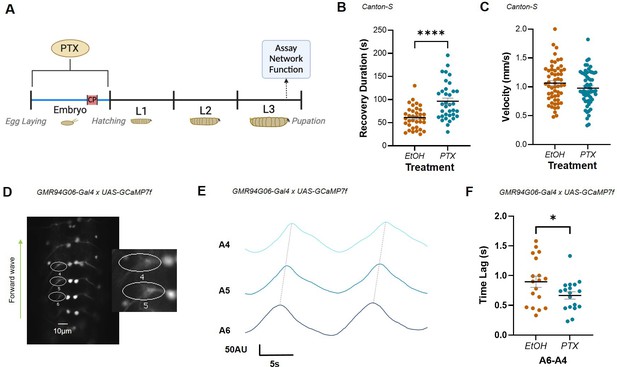
Increased excitation during the embryonic critical period (CP) alters network stability and motoneuron network synchrony.
(A) Schematic demonstrating timeline for picrotoxin (PTX) exposure and assay of network function. PTX-induced increase in activity during development increases third-instar larval duration of recovery from induced seizure (B) but does not change crawling velocity (C). (D) ROIs captured GCaMP signal generated by forward waves passing through ipsilateral anterior corner cell (aCC) motoneuron axons in the L3 ventral nerve cord, abdominal segments (A6-4). Inset: expanded view of ROIs 4 and 5. (E) Representative traces recorded in three adjacent abdominal segments (A6-A4), during two forward waves. Colours represent different segments. Synchrony was measured as the time lag (s) of activity passing between adjacent segments (i.e. between peaks, dotted lines). (F) Embryonic exposure to PTX caused a significant increase in synchronicity across segments A6-A4 vs. EtOH controls.

Increased excitation during the critical period (CP) preferentially increases the strength of excitatory inputs to anterior corner cell (aCC).
(A) Left: schematic demonstrating the use of optogenetics for testing strength of A18a or A31k synaptic drive on evoked action potential firing in aCC. Right: representative traces illustrating the effect of excitatory or inhibitory input on evoked action potential firing in aCC, respectively. (B, C) Current-clamp recordings wherein current (~10 pA) was injected to evoke action potential firing in aCC, prior to optogenetic stimulation of premotor input. Amplitude of inputs was quantified as change in frequency of action potential firing (ΔAP Freq. (Hz)) following optogenetic stimulation of A18a or A31k. Change in action potential firing frequency in A18a (p=0.04), but not A31k (p=0.15), was significantly potentiated by embryonic exposure to PTX vs. vehicle controls (EtOH).
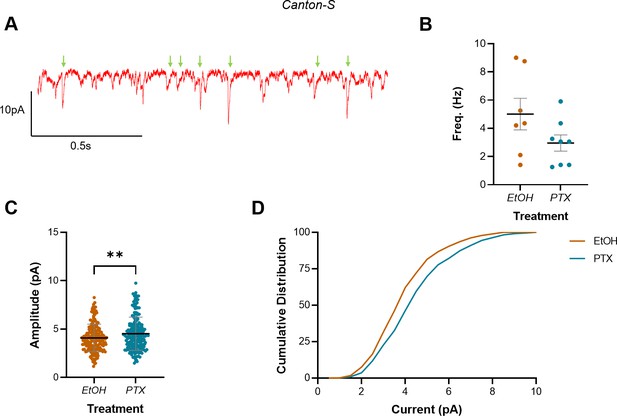
Increasing activity during the embryonic critical period (CP) increased mini amplitude but not frequency in L3 anterior corner cell (aCC).
(A) Representative trace showing minis (green arrows) in L3 aCC. Events not marked are not mini’s and probably represent single channel events (see Figure 4—figure supplement 1 for an expanded version of this same trace). (B) Mini frequency, as events observed in the first 20 s of a whole-cell voltage-clamp recording (Vm held at –60 mV), show no difference between vehicle controls (EtOH) and L3 exposed to picrotoxin (PTX) during embryogenesis. (C) Mini amplitude from the same recordings reveals significantly larger events in larvae that were exposed to PTX during embryogenesis vs. vehicle controls (EtOH). (D) Cumulative distribution (%) of mini amplitude demonstrates a rightward shift in current size, following activity manipulation.

Increasing activity during the embryonic critical period (CP) increased A18a-specific mini amplitude but not frequency in L3 anterior corner cell (aCC).
(A) Representative traces showing minis (mini excitatory postsynaptic potentials [mEPSPs], green arrows) in L3 aCC (Vm held at –60 mV), following optogenetic excitation of A18a. (B) Mini frequency, measured as events observed in the 3 s following optogenetic stimulation of A18a (50 ms light pulse), was not different between vehicle-only controls (EtOH) and L3 exposed to picrotoxin (PTX) during development. (C) Mini amplitude was significantly larger following exposure to PTX during embryogenesis, vs. controls (EtOH). (D) Cumulative distribution (%) of mini amplitudes demonstrates a clear rightward shift in current size, following activity manipulation.
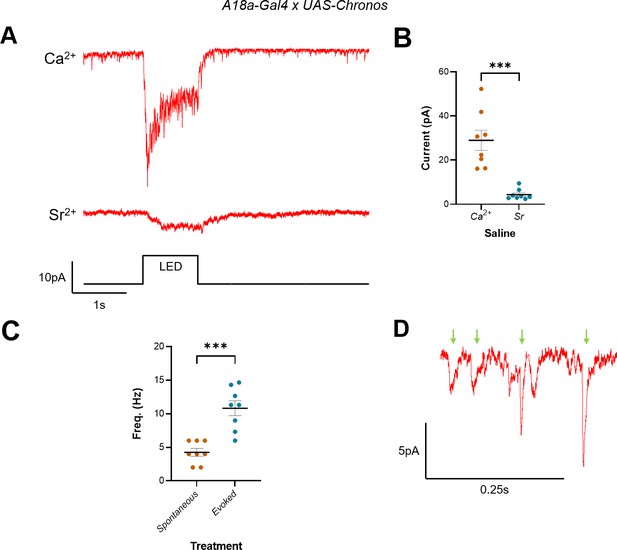
Optogenetic activation of A18a in 6 mM Sr2+ saline stimulates asynchronous release of minis.
(A) Representative traces show synaptic currents recorded in anterior corner cell (aCC) following optogenetic stimulation (1 s LED) of A18a in control (Ca2+) or 6 mM Sr2+ saline. Recordings made in control saline feature large synaptic events (~30 pA), indicative of normal vesicular release from the premotor. By contrast, the large current response to optogenetic stimulation is absent in 6 mM Sr2+. (B) Analysis of peak currents recorded in aCC, elicited by optogenetic stimulation of A18a are significantly larger in control (Ca2+) than 6 mM Sr2+ saline (28.96 ± 4.56 s, Ca2+; 4.4 ± 0.85 s, Sr2+; n = 8, 8; p<0.0001), thus, the changes we observed in current amplitude are consistent with the asynchronous mini release reported when using Sr2+ saline in mammals. (C) Similarly, there is a significant increase in the frequency of asynchronous synaptic events (minis) recorded in aCC in 6 mM Sr2+ saline, in the 3 s following stimulation of A18a (Evoked) vs. the 3 s prior (4.25 ± 0.59 Hz, Spontaneous; 10.83 ± 1.12 Hz, Evoked; n = 8, 8; p<0.0001). (D) Minis (green arrows) were defined as ≥2 pA events with a short rise time and slow decay. In combination, these results suggest recording current in aCC following optogenetic stimulation of A18a in 6 mM Sr2+ saline enables quantifying the frequency and amplitude of minis elicited by this premotor interneuron.

Increasing neural network activity by picrotoxin (PTX) exposure during development does not alter motoneuron dendritic arbor size or excitatory postsynaptic sites.
(A, B) Representative images of anterior corner cell (aCC) motoneurons from larval ventral nerve cords 72 hr after larval hatching (ALH), from control (A) or embryonic exposure to picrotoxin (B). aCC dendritic arbor structure is marked by membrane targeted myr::tdTomato (red), and postsynaptic excitatory sites are marked by a Drep2::YPet fusion protein (cyan). Visualisation of individual aCC motoneurons is achieved by an RN2-enhancer element driving a stochastic FLP-out LexA expression system. (C) tdTomato fluorescence was used for AI-supported 3D reconstruction of the whole aCC dendritic arbor, allowing quantification of total dendrite length (grey segments), branch points (blue spots), and terminals (red spots). (D) Following live imaging, nerve cords were fixed and stained using a fluorophore-tagged nanobody to detect the Drep2::YPet fusion protein (D), enabling high-resolution and photostable imaging of Drep2::YPet puncta for quantification of excitatory postsynaptic site number by semi-automated spot detection (D’). (E) The total dendrite length, number of branch points, and number of terminals are shown for both control and picrotoxin treatments. (F) The number of Drep2::YPet puncta for the larger ipsilateral arbor (‘arbor1’) the smaller contralateral arbor (‘arbor2’), and the combined sum across both arbors (‘aCC’) is shown for both control and picrotoxin treatments. (G) Synapse density, calculated as the total dendrite length divided by total Drep2::YPet positive postsynaptic sites. In (E–G), each point represents a single aCC, where the symbol shape denotes the particular cell.
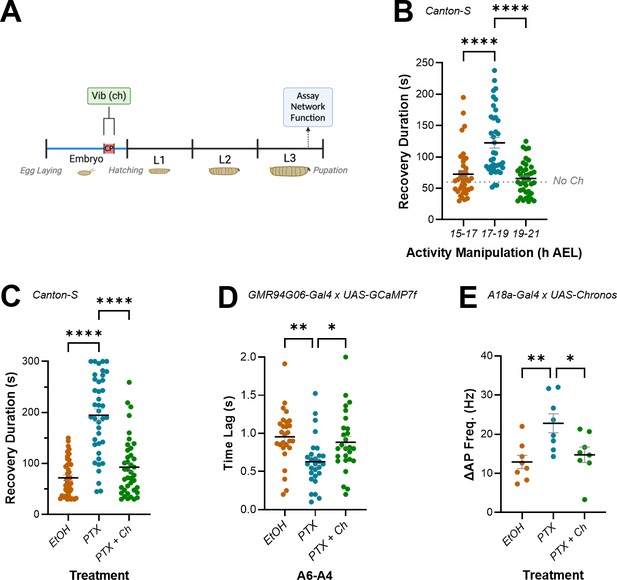
Activating mechanosensory neurons during the critical period (CP) rescues excitation:inhibition (E:I) balance due to increasing activity during embryogenesis.
(A) Schematic showing stimulation of mechanosensory chordotonal neurons (ch) by vibration during the CP (17–19 hr after egg laying [AEL]). (B) Stimulating ch neurons during the CP, but not during 15–17 hr AEL or 19–21 hr AEL, significantly increases mature larval (L3) recovery duration from electroshock-induced seizure. CS is unmanipulated wild-type (Canton-S) controls. (C–E) Exposure to picrotoxin (PTX) during embryogenesis significantly increases mature larval (L3) recovery duration from electroshock-induced seizure, increases network synchronicity, and increases the strength of excitatory (A18a) inputs to motor neurons (aCC). All these effects are rescued by co-activation of ch neurons during the CP (PTX + Ch).
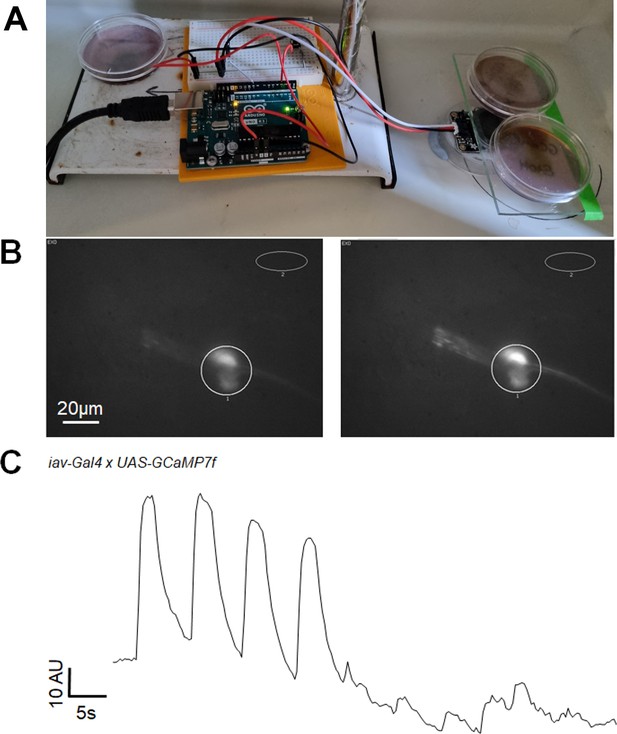
A custom-built device provides mechanical stimulation of chordotonal neurons.
(A) Image of the device, showing an Arduino Uno Rev 3 printed circuit board with microcontroller, which drives a speaker according to timings and frequency (i.e. 17–19 hr after egg laying [AEL] at 0.5 Hz) coded using C++ in Arduino IDE, and with an amplitude adjusted using a potentiometer. (B) GCaMP imaging demonstrating activity in the chordotonal neurons prior to (left) and following (right) stimulation by the device (response shown is to 1 s tone at 80 dB). (C) Representative trace of the activity shown in (B).
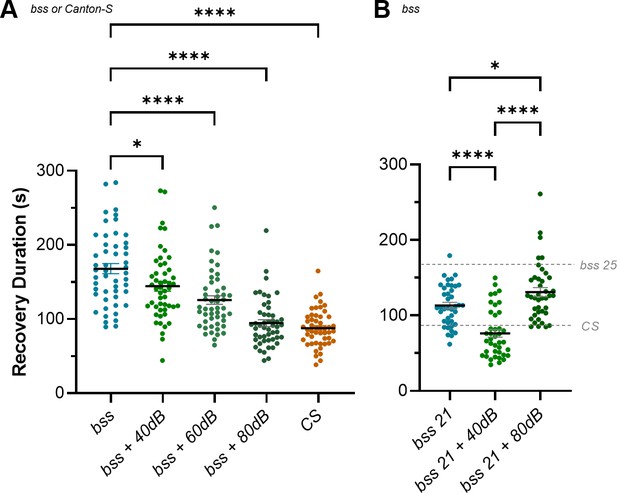
Restoring excitation:inhibition (E:I) balance during the critical period (CP) rescues seizure phenotype caused by a genetic mutation.
(A) The parabss (bss) mutation causes lifelong neural hyperactivity, which is evident in a long duration of recovery from seizure when compared to wild-type controls (Canton-S). This recovery can be rescued by balancing the hyperactivity present with inhibition provided by sufficiently strong stimulation of chordotonal (ch) neurons, during the CP (bss + 80 dB). Weaker stimulation of the ch neuron-mediated inhibition provides intermediate phenotypes (bss + 60 dB, bss + 40 dB) that suggest the function of the mature network reflects the sum of excitatory and inhibitory activity during the CP. (B) parabss raised at 21°C experience less excitation than those raised at 25°C (e.g. in A) during development, and demonstrate a reduced seizure phenotype compared to the latter. This phenotype is completely rescued by 40 dB stimulation of ch neurons (vs. 80 dB at 25°C (A)), which supports the idea that it is a balanced sum of excitatory and inhibitory activity during the CP, that enables normal mature network function. Similarly, exposing parabss raised at 21°C to 80 dB vibration during the CP results in a significant increase in seizure duration. We hypothesise that at this temperature inhibition provided by ch neuron activity is greater than the increase in excitation resulting from the parabss mutation.
Tables
Reagent type (species) or resource | Designation | Source or reference | Identifiers | Additional information |
---|---|---|---|---|
Genetic reagent (Drosophila melanogaster) | parabss1(bss) | Laboratory stocks | Nav channel hypermorph | |
Genetic reagent (D. melanogaster) | GMR94G06-Gal4 | Gift from Juan J. Pérez-Moreno Pérez-Moreno and O’Kane, 2019 | Expressed in aCC | |
Genetic reagent (D. melanogaster) | 15B07-Gal4 -Gal4 | Gift from Aref Zarin | Expressed in A18a | |
Genetic reagent (D. melanogaster) | A31k-Gal4-Gal4 | Gift from Akinao Nose | Expressed in A31k | |
Genetic reagent (D. melanogaster) | iav-GAL4 | Bloomington Drosophila Stock Center | BDSC #52273 | Expressed in chordotonal neurons |
Genetic reagent (D. melanogaster) | UAS-Chronos | Bloomington Drosophila Stock Center | BDSC #77115 | Green-shifted channelRhodopsin |
Genetic reagent (D. melanogaster) | UAS-GCaMP7f | Bloomington Drosophila Stock Center | BDSC #80906 | Calcium activity indicator |
Genetic reagent (D. melanogaster) | LexAop2-Flp2 | Gift from Jan Felix Evers Gärtig et al., 2019 | lexAoperon flip-out line | |
Genetic reagent (D. melanogaster) | 13xLexAop2-IVS-myr::tdTomato (su(Hw)attP5) | Generated for use in the present study | BDSC #56142 | Modified lexAoperon flip-out line |
Chemical compound, drug | Picrotoxin | Sigma-Aldrich, UK, or Merck, NJ | Sigma-Aldrich P1675 or Merck 528105 | Toxin that increases neural activity |
Software, algorithm | Arduino IDE | Arduino | Arduino 1.8.19 | Environment used for programming Arduino Uno |
Other | Arduino Uno Rev 3 | Arduino | Printed circuit board with ATmega 328P microcontroller | |
Other | Adafruit STEMMA Speaker | Adafruit | Plug and Play Audio Amplifier | 1 W, 8 Ohm speaker |