Plastic vasomotion entrainment
Figures
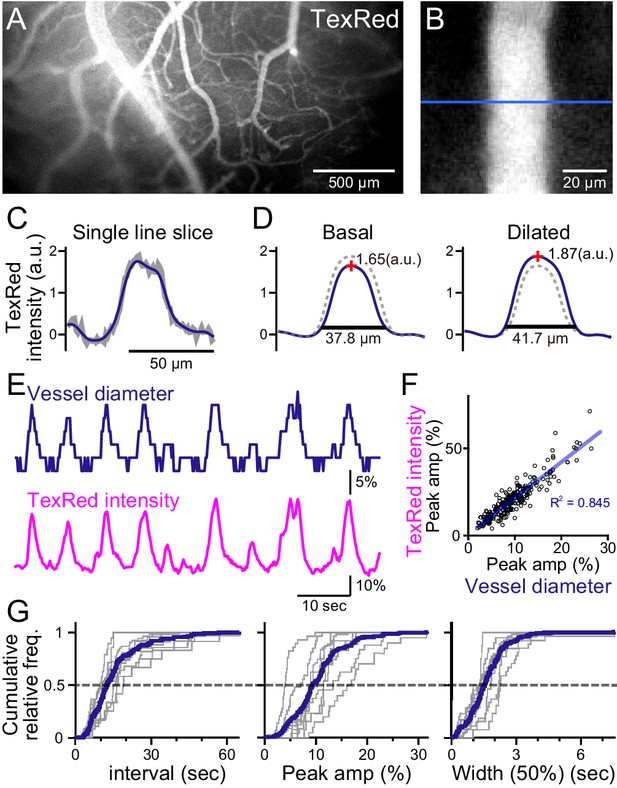
Spontaneous vasomotion detection.
(A) A fluorescence image of a mouse brain through the thinned-skull cranial window using a single-photon wide-field macro-zoom microscope. TexasRed was intravenously injected and the blood vessels on the brain surface (between bregma and lambda) could be readily resolved. (B) Magnified image of a single blood vessel. TexasRed fluorescence intensity profile was calculated at the cross-section indicated by the blue line. (C) An example of a single-line intensity profile. Raw traces (gray bold line) were filtered to derive the boundaries between the vessels and parenchyma. (D) TexasRed intensity profiles averaged for multiple lines. The profile during the basal, undilated phase (solid line shown on the left, the same profile shown as a dotted line on the right) and the dilated phase (solid line on the right, dotted line on the left). Peak amplitudes of the intensity profiles (red cross) and full width at 10% maximum (horizontal lines). (E) An example of a time-series data of the calculated vessel diameter and TexasRed intensity. (F) Correlation of the peak amplitude of the calculated vessel diameter with the TexasRed peak intensity (n = 214 peaks). (G) Characteristics of the vessel dilation events across multiple vessels were summarized. Inter-event interval (left), the peak amplitude of the vessel diameter (middle), and the half-width of the duration of individual events (right) are shown for individual sessions collected from multiple vessels (gray lines, n = 9 sessions from five vessels of three animals; duration of each session was from 1.5 to 11 min). Averaged cumulative histograms are shown as blue lines.
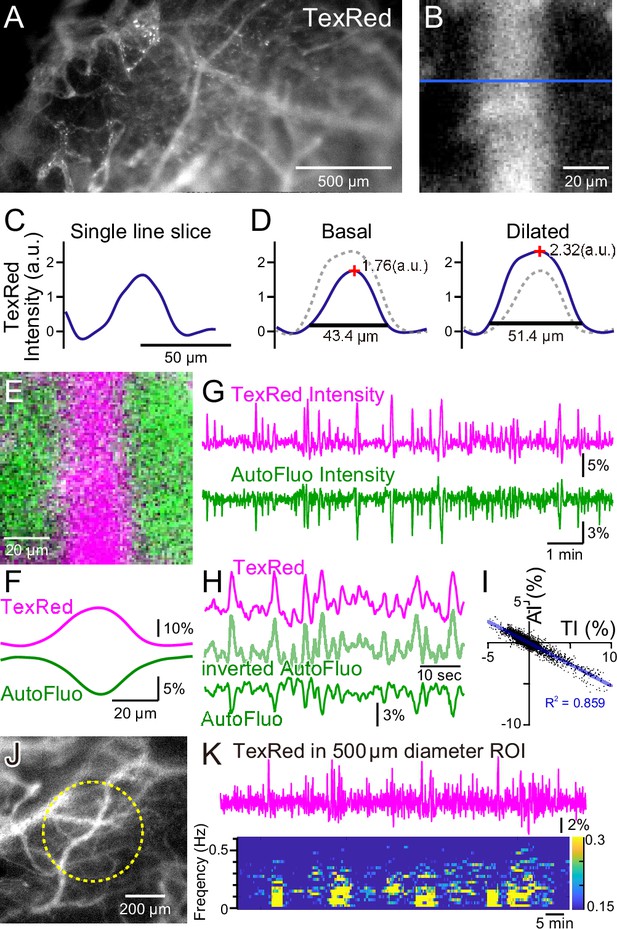
Autofluorescence signals could be used for vasomotion detection through the intact skull.
(A) A fluorescence image of a mouse brain through the intact skull (between bregma and lambda). Although with less resolution, individual blood vessels containing TexasRed could be visualized. (B) Magnified image of a single blood vessel. (C) A single-line intensity profile. (D) Averaged TexasRed intensity profile at the basal (left) and the dilated (right) states. The intensity profiles were shallower compared to the profiles analyzed with thinned-skull images. Therefore, rather than the vessel diameter estimated by the full width at 10% maximum of the intensity profile, the TexasRed intensity would likely better reflect the volume changes of the blood vessels. (E) A merged image of the TexasRed signal in the blood vessel (magenta) and the autofluorescence signal likely coming exclusively from the brain parenchyma (green). (F) Spatial intensity profile along the cross-section of TexasRed and autofluorescence signals in (E). (G) The temporal transitions of the TexasRed and the autofluorescence intensity signals at a region of interest (ROI) encompassing the vessel are shown in (E). The two signals mostly mirrored each other, suggesting that vessel dilation appears as the TexasRed intensity increase and the autofluorescence intensity decrease. (H) An enlarged segment of the traces is shown in (G). The inverted autofluorescence trace (light green) resembles the TexasRed intensity trace. (I) Autofluorescence intensity (AI) plotted against the TexasRed intensity (TI) for the duration of the traces shown in (G) (n = 3440 data points). (J) An image of the mouse brain through the intact skull. 500 diameter µm circular ROI is shown (dotted yellow line). This diameter corresponds closely to the diameter (400 µm) of the optical fiber used in fiber photometry experiments. (K) Spontaneous TexasRed fluctuation (top) was detected through the intact skull within the ROI shown in (J). The spectrum below shows that the low temporal frequency vasomotion does not continuously occur and short phases of vascular oscillation occur with frequency not locked to a narrow specific range.
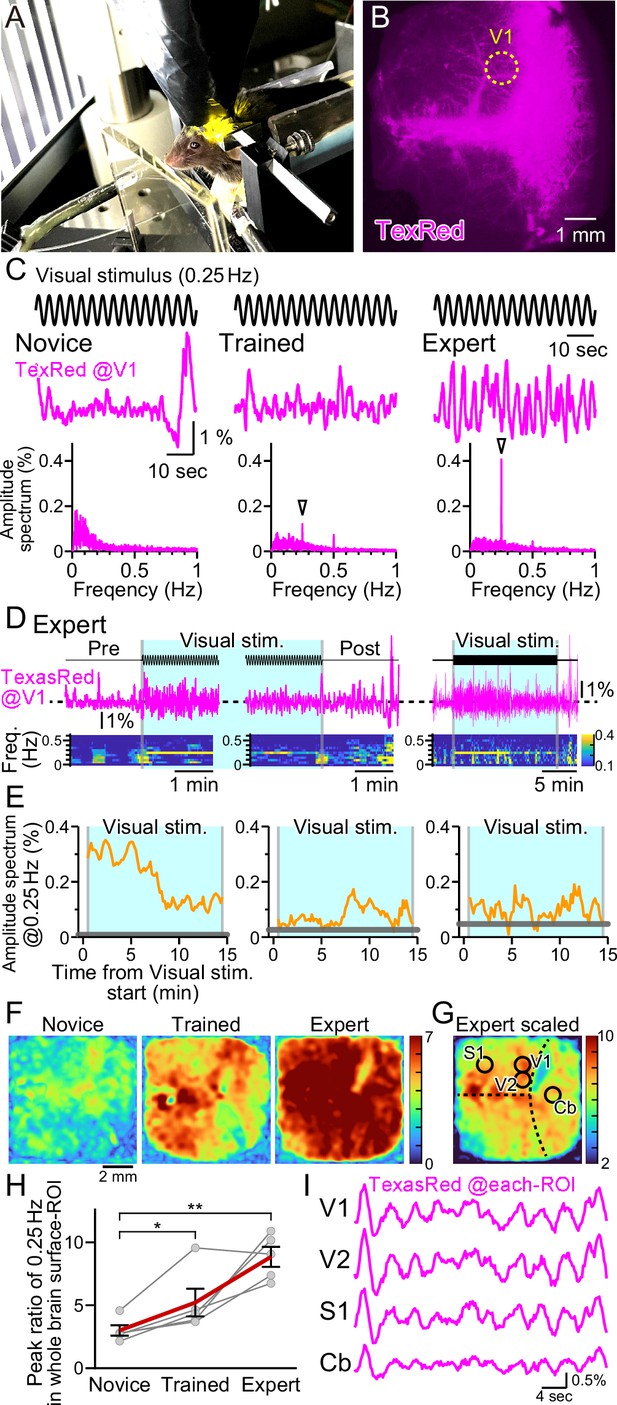
Vasomotion entrainment with the oscillating visual stimulus.
(A) Fluorescence images were taken from the whole brain surface through the intact skull using the macro-zoom microscope in awake mice. Horizontally oscillating (at 0.25 Hz temporal frequency) vertical stripes are shown as the visual stimuli on the two perpendicular monitors placed in front of the mouse. (B) Representative image of the near-whole brain surface after intravenous injection of TexasRed. The location of region of interest (ROI) in V1 is shown with the yellow dotted line. (C) TexasRed signals at V1-ROI during visual stimulation are shown. After repeated sessions, the vasomotion gradually became entrained to the frequency of the visual stimulus. At the first training session (Novice, left), no specific peak was observed in the amplitude spectrum (bottom) of the TexasRed signals (middle). After several trainings (Trained, at fourth training session, middle), a 0.25 Hz peak (arrowhead) in the amplitude spectrum appeared. After saturated training (Expert, at 8th–11th training session, right), the 0.25 Hz oscillation of the TexasRed signal was apparent in the raw traces (middle). (D) An example data from an Expert mouse. Immediately after the onset of a visual stimulation session, the 0.25 Hz band appeared in the TexasRed trace (left). The 0.25 Hz band dissipated immediately upon the cessation of the visual stimulation (middle). The 0.25 Hz band lasted throughout the 15 minute session in this particular mouse, albeit the amplitude became smaller toward the end of the session (right). (E) The amplitude values at 0.25 Hz in the spectrogram were plotted against time for three different animals. The left graph is from the same Expert animal and the same session as shown in (D). The two other graphs (middle, right) are from different sessions. The amplitudes at 0.25 Hz during the visual stimulation were mostly higher than those before the stimulation (horizontal gray line); however, the amplitude did not stay constant in most cases. The vasomotion appears to come in and out of the frequency lock to the visual stimulus. (F) The peak amplitude at 0.25 Hz over the mean amplitude in the 0.1–1 Hz range (PR0.25) was calculated for each pixel in the whole brain during the visual stimulation for Novice, Trained, and Expert mice. PR0.25 increase with repeated sessions was not restricted to the visual cortex but a near-uniform increase was observed throughout the whole brain surface. The color bar indicates PR0.25. (G) The color scale for the Expert example shown in (F) is saturated. Therefore, a different color scale (right) was applied for the same data. (H) The averaged PR0.25 in the whole brain surface ROI gradually increased as the training progressed (n = 5 animals). Novice vs. Trained, p=0.039, *p<0.05; Novice vs. Expert, p=0.0030, **p<0.005; paired t-test with Holm correction for multiple test. Data from individual animals are shown in gray, and the averaged data across all animals are shown in red. Data are presented as mean ± SEM, as error bars. (I) TexasRed signals in ROIs at V1, V2, S1, and cerebellum (Cb). Signals in different locations fluctuated with similar frequency with similar phases.
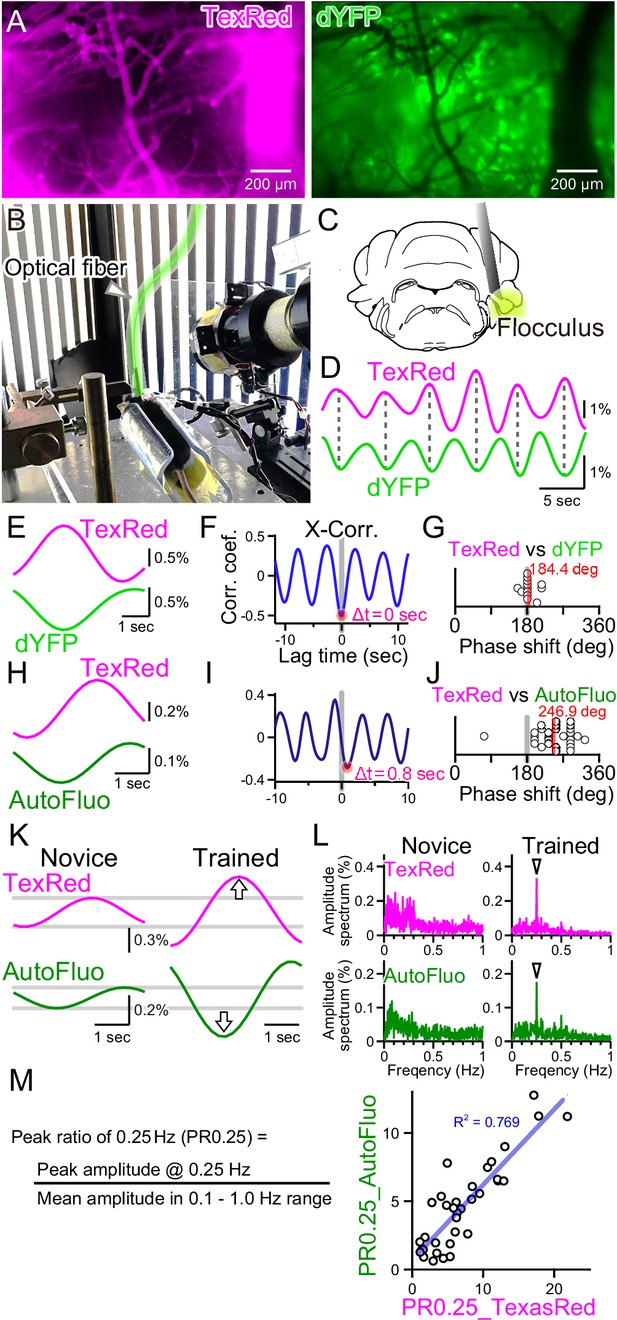
Autofluorescence signals could be used to quantify the vasomotion entrainment.
(A) Images of the TexasRed fluorescence in the blood vessels and the direct YFP fluorescence expressed in astrocytes of a transgenic mouse. The blood vessels appear as dark ‘shadows’ in the dYFP fluorescence image. (B) An optical fiber was implanted in the cerebellum close to the flocculus and another optical fiber (highlighted in green) from the fiber photometry apparatus was connected to this implanted fiber. Horizontally oscillating visual stimulation was presented on the monitors. A hot mirror reflected the IR image of the eye and the eye location was monitored using a camera. (C) Location of the optical fiber implantation relative to the cerebellar flocculus. (D) Simultaneously recording of the TexasRed and the dYFP signals from the optical fiber implanted in the flocculus during the horizontal optokinetic response (HOKR) visual stimulation session. The dYFP mirrored the TexasRed signal fluctuations. Fluorescence signal data were filtered at 150–350 mHz. (E) 20 5 s segments of the TexasRed and the dYFP signal traces were extracted and averaged. The two traces nearly completely mirrored each other. The temporal frequency of the visual stimulation presented to the transgenic mouse was 0.20 Hz. (F) Cross-correlation between the two traces had a negative peak with a lag time of Δt of 0 s. (G) The phase shift between TexasRed and dYFP signals was calculated for n = 13 segments with 5 min durations each. (H) In a separate set of experiments, the TexasRed signal and the endogenous autofluorescence signal were simultaneously recorded in wild-type mice. 20 4 s segments were extracted and averaged. The traces were not completely inverted and a certain amount of phase shift was observed. The temporal frequency of the visual stimulation presented to the wild-type mouse was 0.25 Hz. (I) Cross-correlation of TexasRed and Autofluorescence signals. Δt of 0.8 s was observed in this particular example. (J) Phase shifts in TexasRed and autofluorescence signals were calculated for n = 28 segments. (K) Averaged data of 35 traces of the extracted 4 s segments of the TexasRed and the autofluorescence signals during the first training (Novice) and the fourth training (Trained). Data was filtered with 150–350 mHz. An increase in the amplitudes was observed from both signals. (L) Amplitude spectrums for TexasRed and autofluorescence during the 5 min of the first training (Novice) and that of the fourth training (Trained). Signal data were high-passed filtered at 50 mHz. (M) PR0.25 of the TexasRed signals and the autofluorescence signals in the 5 min during the visual stimulation were plotted against each other. A clear correlation was observed indicating that the autofluorescence signal can be used to estimate the magnitude of the vasomotion frequency-locked to the visual stimulus.
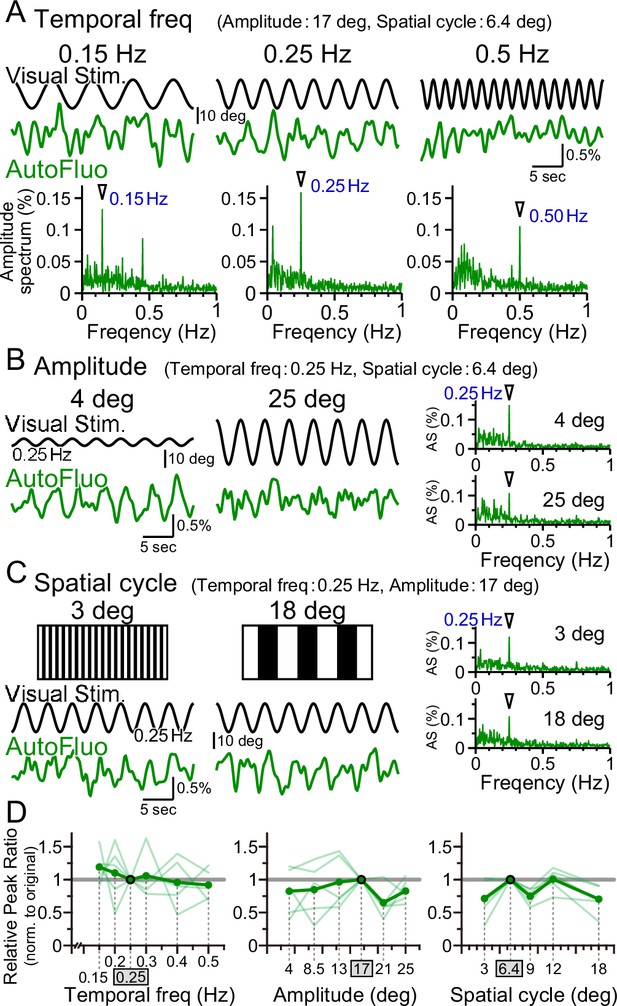
Vasomotion entrainment over a wide range of visual stimulation parameters.
(A–C) Autofluorescence signals at the flocculus in response to visual stimulation with various parameters. In the original condition, the temporal freq (the temporal frequency of horizontal oscillation) was 0.25 Hz, the amplitude (the spatial amplitude of the horizontal oscillation) was 17° in the visual angle of the mouse, and the spatial cycle (the horizontal spatial cycle of the vertical stripes) was 6.4°. All the fluorescence signal data were filtered with 50–1000 mHz. (A) The temporal frequency of visual oscillation was varied. The location of the peaks in the autofluorescence signal amplitude spectrogram exactly matched the frequency of the visual stimulus in almost all cases (arrowhead). (B) The amplitude of the visual oscillation was varied. (C) The spatial cycle of the stripe patterns was varied. (D) The relative peak ratio of the autofluorescence signal at the temporal frequency matching that of the presented visual stimulus was calculated. This relative peak ratio was normalized to that of the original condition. Varying the temporal freq, the amplitude, or the spatial cycle almost had no effect on the relative peak ratio. This suggests that vasomotion can tune in to any visual stimulation within the parameter range that we tested. Significant differences were shown only when the spatial cycle was varied (spatial cycle, 6.4° [original] vs. 9°, p=0.0028, *p<0.0125; 6.4° [original] vs. 18°, p=0.015, *p<0.017; one-sample t-test with Holm correction for multiple tests). Individual mice’s data (as an average from the three sessions of the 5 min test) are shown in pale green, and the average data from the examined mice (n = 5 mice) are shown in dark green.
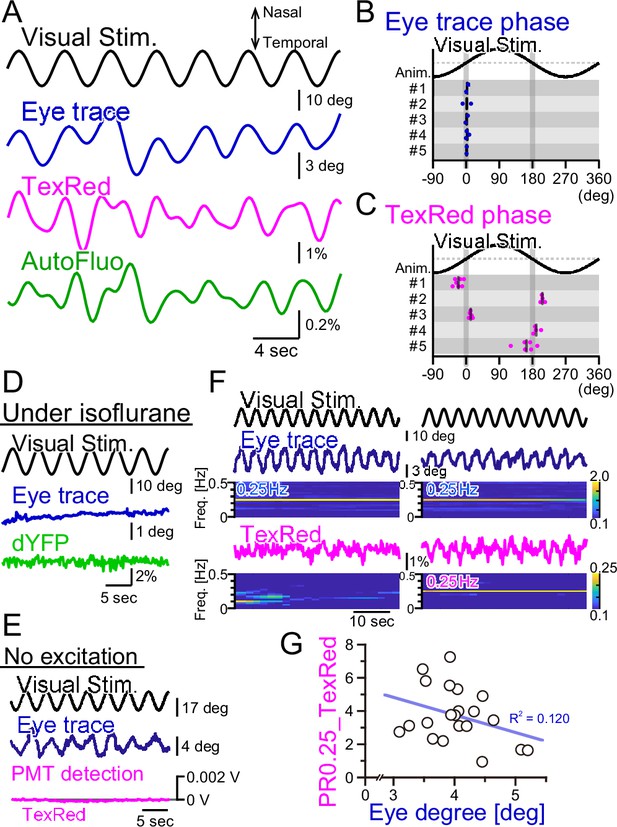
Fluorescence fluctuations are not the results of eye movement artifact.
(A) An example of the visual stimulation time course, the eye trace, the TexasRed signal, and the autofluorescence signal fluctuations are shown. The data was filtered with 50–500 mHz. (B) The phase of the eye trace relative to the visual stimulus. The visual stimulus was presented with a 4 s cycle (0.25 Hz), thus, the eye trace data was segmented with 4 s durations during the first 5 min of each training. The segmented data were aligned, averaged, and fitted with a sine curve. The phase difference between the visual stimulus and the fit to the averaged eye trace was calculated. The mean of the phase in each animal across multiple sessions is shown as a vertical black bar. The average phase lag was 2.3 ± 1.0° in five animals, indicating that the eye trace synchronize nearly completely with the visual stimulus. (C) The phase of the TexasRed signal fluctuations relative to the visual stimulus. The average TexasRed signal fluctuation with a 4 s cycle was calculated from segments extracted from several sessions, each with 1–6 min in duration. The phase lag of the TexasRed signal relative to the visual stimulus was clustered either at 0° or 180°. This shows that the local brain blood volume in the right flocculus becomes the largest when the right eye is either in the most nasal position or the temporal position. (D) Under anesthesia with isoflurane, the eye movement was not induced. The dYFP signal from the YCnano50 expressed in astrocytes also did not oscillate. (E) With no excitation light sent to the optical fiber, the PMT detected no signal (0 V). The presented visual stimulus did not reach the fiber optics to directly induce oscillatory signals. (F) The amplitude of the eye movement was normally nearly constant during a single session; however, slightly strong (left) and weak (right) eye movements were observed. The TexasRed signal fluctuation at the frequency locked to the visual stimulus was not constant during the session and PR0.25 of the TexasRed signal could be seen in some (right) but not the other (left) segments of the session. (G) PR0.25 of TexasRed signal was plotted against the oscillatory eye movement amplitude in 40 s segments within a single visual stimulation session (15 min). The first segment was excluded (n = 21 segments total). No correlation was found between these two factors.
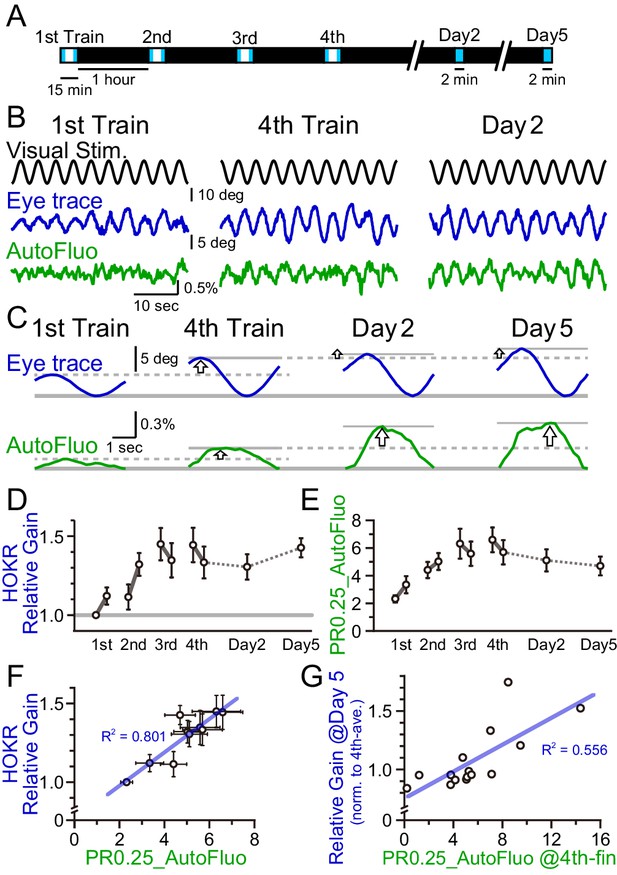
A concomitant increase in the horizontal optokinetic response (HOKR) performance and vasomotion entrainment.
(A) Schematic schedule of the HOKR spaced training paradigm. The 15 min training sessions were repeated at 1 hr intervals for four times on day 1 (the training day). The average amplitudes of the eye movement during the first and last 3 min of each training session were analyzed (during the period indicated by the blue box in the schematics). The 2 min tests measuring long-term memory were done on days 2 and 5. (B) Representative traces of the visual stimulus, the eye trace, and the autofluorescence during the first and the fourth training on day 1 and the test done on day 2. The amplitude of the eye movement and the vasomotion gradually increased in amplitude with repeated sessions. (C) The eye traces and the autofluorescence traces were segmented in 4 s durations, corresponding to the visual stimulation frequency of 0.25 Hz, and aligned and averaged. (D) The amplitude of the eye movement relative to that of the start of the first training session on day 1 was refined as the HOKR relative gain. Average HOKR relative gain across n = 15 animals is plotted (mean ± SEM). (E) PR0.25 of the autofluorescence signals was averaged across n = 15 animals and plotted against sessions. (F) Averaged HOKR relative gain was plotted against the averaged PR0.25 of autofluorescence signals. Error bars indicated S.D. (n = 15 animals). (G) HOKR relative gain on day 5 normalized to that on the fourth training on day 1 was plotted against the PR0.25 of autofluorescence signals at the end of the fourth training on day 1 (n = 15 animals).