Greatwall-phosphorylated Endosulfine is both an inhibitor and a substrate of PP2A-B55 heterotrimers
Figures
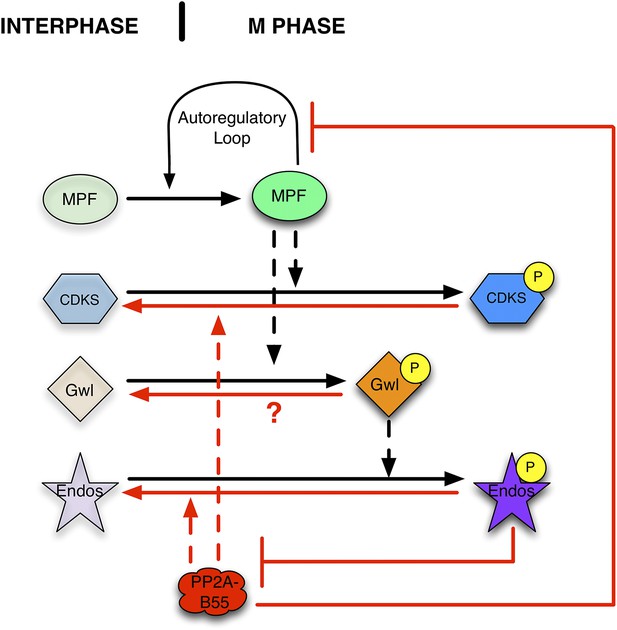
Function of the Gwl → pEndos ⊣ PP2A-B55 module in cell cycle transitions.
The major driver for transitions between interphase and M phase is the cyclic activation and degradation of MPF (Cdk1-Cyclin B). When activated (in part through a feed-forward autoregulatory loop involving the kinases Myt1 and Wee1 and the phosphatase Cdc25; not shown), MPF phosphorylates many substrates (CDKSs) that play key roles in M phase events. One such MPF substrate is the kinase Greatwall (Gwl), which in its active form phosphorylates Endosulfine (Endos). Phosphorylated Endos binds to and inhibits the phosphatase PP2A-B55. This inhibition protects MPF substrates, including components of the autoregulatory loop, from premature dephosphorylation during M phase entry. During M phase exit, MPF is inactivated by degradation of its Cyclin B component (not shown), and PP2A-B55 becomes reactivated to dephosphorylate many MPF substrates. M phase exit also requires the dephosphorylation and inactivation of both Gwl and Endos. Here, we show that PP2A-B55 catalyzes Endos dephosphorylation. The activities responsible for the inactivation of Gwl currently remain unknown.
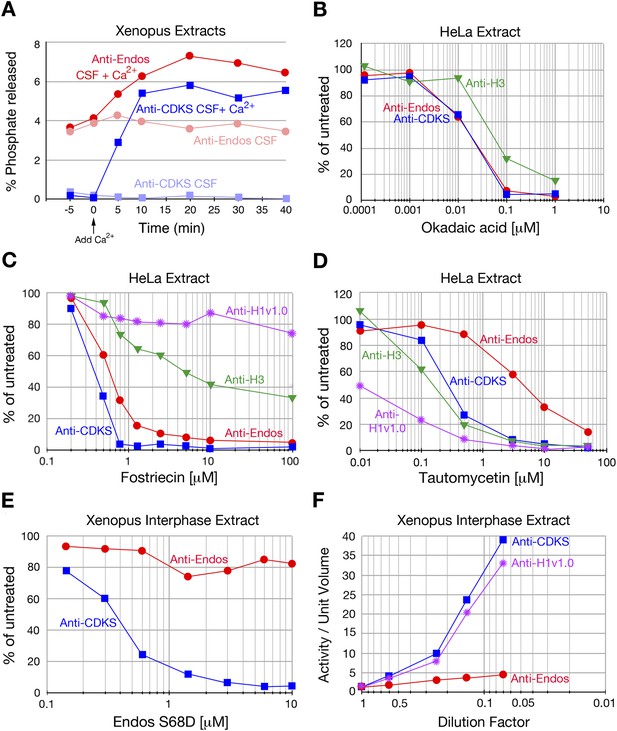
Characterization of anti-Endos in extracts.
In all parts of this figure, red circles depict anti-Endos, whereas blue squares represent anti-CDKS. (A) Anti-Endos is present during M phase. Xenopus CSF (M phase) extracts were incubated at 22°C. At time t = 0, Ca2+ was added to half of the extract to induce M phase exit; control extract without Ca2+ remained in M phase. At the indicated times, aliquots were assayed for anti-Endos and anti-CDKS as described in ‘Materials and methods’. During M phase, anti-CDKS (light blue squares) is undetectable, whereas anti-Endos (light red circles) is active. As the extracts exit M phase (interphase is achieved within 15–20 min of Ca2+ addition; [Yu et al., 2006; Zhao et al., 2008; Castilho et al., 2009]), anti-CDKS activity (dark blue squares) is strongly induced, while anti-Endos (dark red circles) increases about twofold. (B–E) Drug sensitivities of phosphatase activities. Y-axis values represent the percentage of the phosphatase activity for the given combination of extract and substrate measured in the absence of the inhibitor. Anti-Endos and anti-CDKS have similar sensitivities to okadaic acid and fostriecin, but anti-Endos is substantially more resistant than anti-CDKS to tautomycetin and phosphomimetic Endos S68D. In B and C, green triangles represent dephosphorylation activity against CDK-phosphorylated Histone H3; in C, purple stars are activity against CDK-phosphorylated Histone H1v1.0. In part C, the fostriecin resistant portions of the H3 phosphatase (about 40% of the total) and the H1v1.0 phosphatase (about 80% of the total) likely represent PP1 activity. The HeLa extracts examined in panels B–D were from asynchronous cells, the vast majority of which are in interphase. (F) The specific activities of anti-CDKS and anti-H3 increase upon dilution of the extract, presumably because weakly binding inhibitors are titrated away, but the specific activity of anti-Endos increases at most only marginally upon dilution. The y-axis shows the phosphatase activity on the indicated substrates, normalized to the original volume of undiluted extract. In all panels, n = 1; biological and evolutionary replicates of the experiments in panels B–D are presented in Figure 2 figure supplements 1–5.
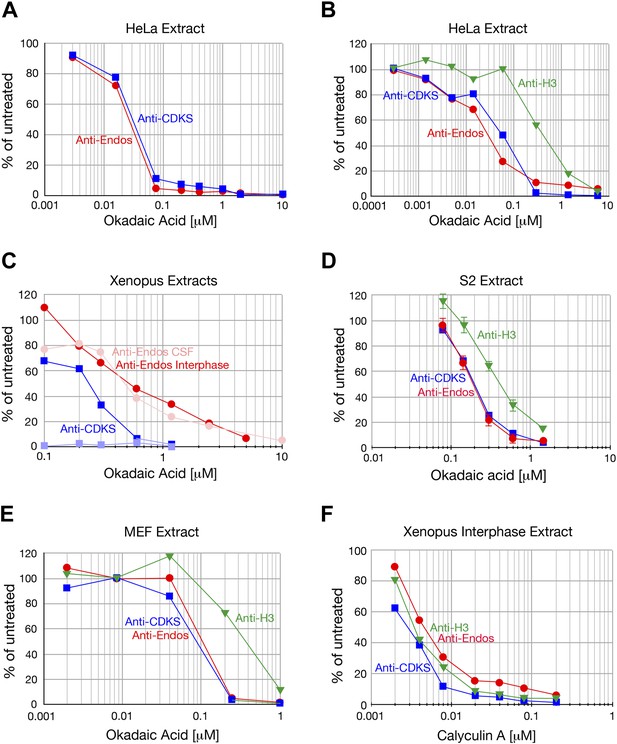
Anti-Endos is completely inhibited by okadaic acid and calyculin.
A In all parts of this figure, red circles depict anti-Endos, and blue squares are anti-CDKS; in B and C green triangles represent dephosphorylation activity against Histone H3. In all panels except part D, each symbol represents a single assay. (A and B) Biological replicates of the experiment shown in Figure 2B. (C) Xenopus CSF extracts were untreated (M phase) or treated with Ca2+ for 30 min (interphase) and then assayed for phosphatase activity. As in Figure 2A, anti-CDKS is undetectable in CSF extracts. The sensitivity of anti-Endos to okadaic acid is similar in M phase and interphase extracts; in both cases, the IC50 for anti-Endos is about threefold higher than that for anti-CDKS in interphase. We presume this difference reflects the substantial fraction of anti-Endos in Xenopus extracts due to PP1 (Figure 2—figure supplement 2). (D) In asynchronous S2 (Drosophila) cell extracts, anti-CDKS and anti-Endos have nearly identical dose-response curves to okadaic acid; these activities are slightly more sensitive to okadaic acid than is the phosphatase activity directed at Histone H3 substrate. In this panel, each symbol represents the average of four technical replicates; error bars are not shown for the anti-CDKS activity to aid readability, but their magnitude is consistent with those of the other assays. (E) Evolutionary replicate of panels A–D performed with an extract made from asynchronous mouse embryo fibroblast (MEF) cells. (F) Phosphatase activities against all three substrates are completely suppressed by calyculin A; reported IC50 values for this drug with respect to PP1, PP2A, PP4, PP5, and PP6 are all similar (Swingle et al., 2007).
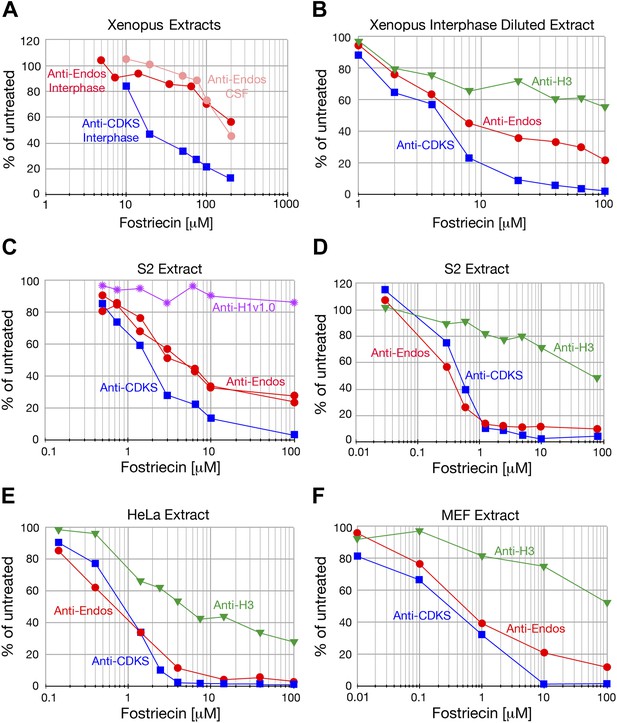
Anti-Endos is mostly fostriecin-sensitive.
In all parts of this figure, red circles depict anti-Endos, blue squares are anti-CDKS, green triangles are activity against Histone H3, and purple stars are anti-H1v1.0. Each symbol represents a single assay. (A) The fostriecin sensitivities of anti-Endos in M phase (CSF extracts) and interphase Xenopus egg extracts are similar. A proportion of anti-Endos in these concentrated egg extracts is more fostriecin-resistant than is the anti-CDKS in the same extracts; the exact proportion is difficult to estimate because the maximal amount of fostriecin that could be added was insufficient even to inhibit anti-CDKS completely. (B–F) In extracts of Xenopus eggs diluted 1:4 in phosphatase buffer (B), of Drosophila S2 cells (C and D are biological replicates), or of mouse MEF cells (F), anti-Endos activity is more resistant to fostriecin than is anti-CDKS, but is less resistant than are the phosphatase activities against Histone H1v1.0 or Histone H3. In panel C, two technical replicates of the anti-Endos assay are shown. These data suggest that PP1-like enzymes account for most of the activity against Histone H1v1.0 and about half of that against Histone H3. From the amount of anti-Endos activity remaining at fostriecin concentrations of 100 μM sufficient to inhibit anti-CDKS completely, we estimate that 70–90% of anti-Endos is fostriecin-sensitive (depending on the extract) and is therefore likely to be PP2A, PP4, or PP6. The minor fostriecin-resistant component is labile and is lost from certain extracts such as D and E (see also Figure 2C).
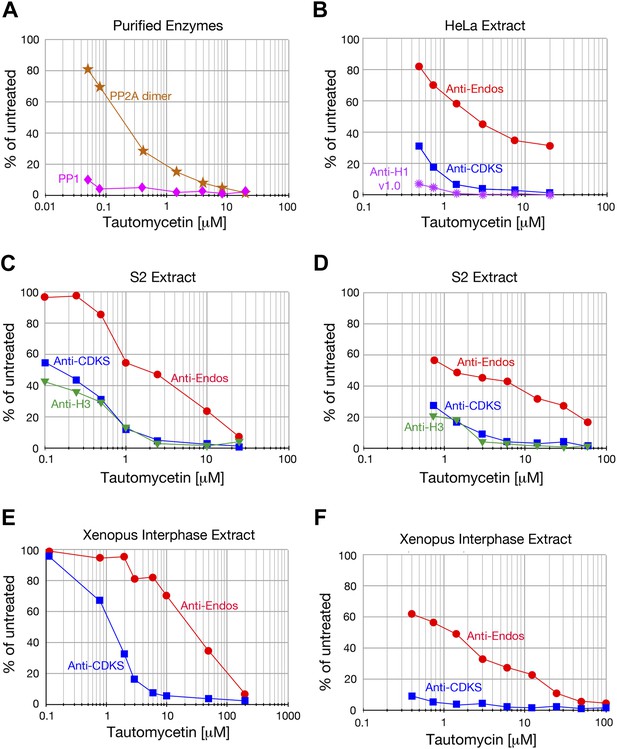
Anti-Endos is more tautomycetin/tautomycin-resistant than anti-CDKS.
(A) As expected from the literature (Mitsuhashi et al., 2001), purified PP1 (orange stars) is more sensitive to tautomycetin than is an equal molar amount of purified PP2A A-C dimer (pink diamonds) measured with myelin basic protein phosphorylated with PKA kinase as substrate. (B–F) The IC50 for tautomycetin (B–E) or tautomycin (F) is consistently ∼10-fold higher for anti-Endos than for anti-CDKS when measured in the same extract. The activity against CDK-phosphorylated Histone H1v1.0 is more sensitive than either to tautomycetin, consistent with the argument that the major phosphatase targeting this substrate is PP1. Each data point represents a single assay; C and D are biological replicates using different extracts of S2 cells.
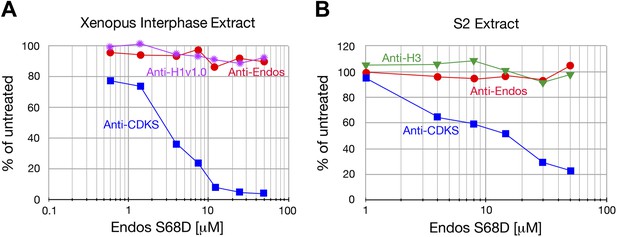
Anti-Endos is resistant to phosphomimetic Endos.
Although anti-CDKS activity is strongly inhibited by phosphomimetic Endos (S68D), anti-Endos activity is insensitive to addition of this molecule. The dephosphorylations of Histone H1v1.0 or Histone H3 substrates are also resistant to Endos S68D, as would be expected of substrates that are targeted by PP1 or any phosphatases other than PP2A-B55. Each data point represents a single assay; panel A is a biological replicate of the results shown in Figure 2E.
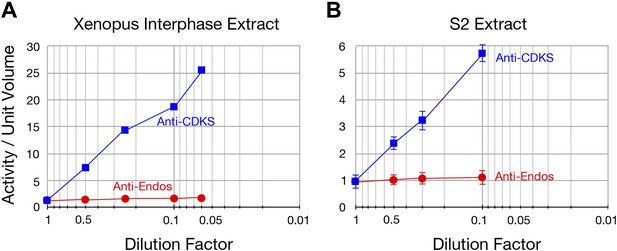
The specific activity of anti-Endos does not increase upon substrate dilution.
The y-axis shows the phosphatase activity on the indicated substrates, normalized to the original volume of undiluted extract. Panel A is a biological replicate of the experiment shown in Figure 2F, n = 1; in panel B, the data points each represent the average of three technical replicates with error bars shown. The reason that the dilution effect is less pronounced for the extract in part A likely reflects the fact that the original concentration of this extract (prior to dilution) was about fourfold lower than that in panel A.
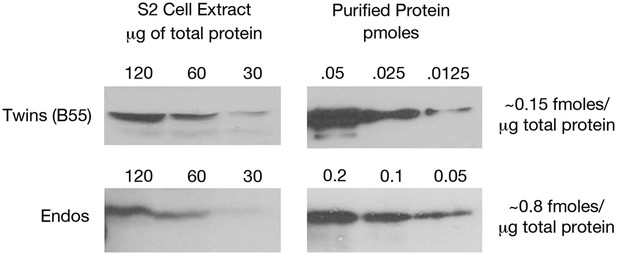
Estimating relative levels of Twins (B55) and Endos proteins in Drosophila S2 cells.
Sequential dilutions of purified recombinant proteins and of total S2 cell extracts were compared on Western blots using antibodies against these two proteins. Total protein amounts in extracts were determined by nanodrop spectrophotometry.
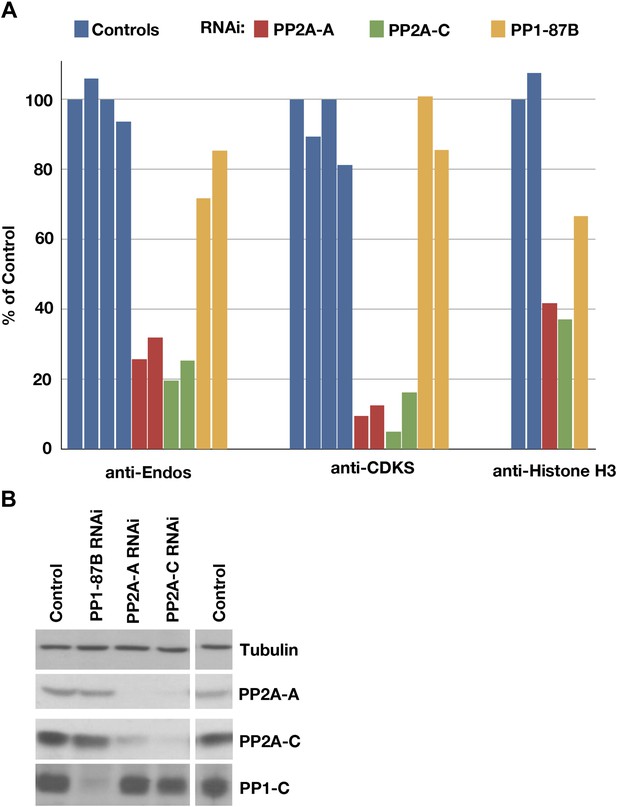
Depletion of PP2A by RNAi disrupts a major component of anti-Endos activity.
(A) Phosphatase assays for anti-Endos, anti-CDKS, and anti-Histone H3 activities in mock-treated control Drosophila S2 tissue culture cells (blue), and S2 cells treated with dsRNAs for the PP2A-A subunit (red; the gene is PP2A-29B), the PP2A-C subunit (green; the gene is mts), and the major PP1-C subunit (yellow; the gene is PP1-87B, which accounts for more than 80% of PP1-related activity against generic substrates [Dombradi et al., 1990]). Each column represents a separate biological replicate (n = 4 for the controls and n = 2 for each of the three RNAi treatments); values were normalized for total protein concentrations in the extracts. One of the four replicates of the control assay was arbitrarily chosen as the 100% reference. The data indicate that a form of PP2A is responsible for about 90% of anti-CDKS, ∼70 to 80% of anti-Endos, and ∼60% of anti-H3; the residual activities are likely due mostly to forms of PP1, although PP5 may also contribute in the case of anti-H3 (Figure 4). (B) Western blots showing effectiveness of the RNAi treatments. As reported elsewhere (Kamibayashi et al., 1992), the stabilities of the PP2A-A and PP2A-C subunits are mutually interdependent.
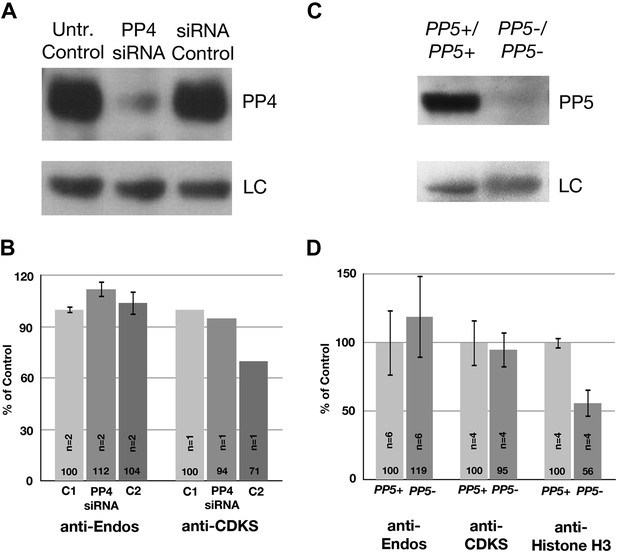
Neither PP4 nor PP5 is a major contributor to anti-Endos.
(A and B) Treatment of HeLa cells with siRNA to the PP4 catalytic subunit strongly reduces the amount of PP4 but does not obviously affect anti-Endos levels. C1 represents control cells that are not treated with siRNA; C2 cells were treated with a control-scrambled siRNA. (C and D) No obvious changes in anti-Endos levels are seen in the mutant mouse MEF cells homozygous for a null mutation in the gene encoding the PP5 catalytic subunit (Yong et al., 2007) relative to control MEF cells. PP5 may be a minor contributor to Histone H3 dephosphorylation. In panels A and C, LC are background bands used as loading controls for the Western blots. In panels B and D, the number of technical replicates is indicated in each bar.
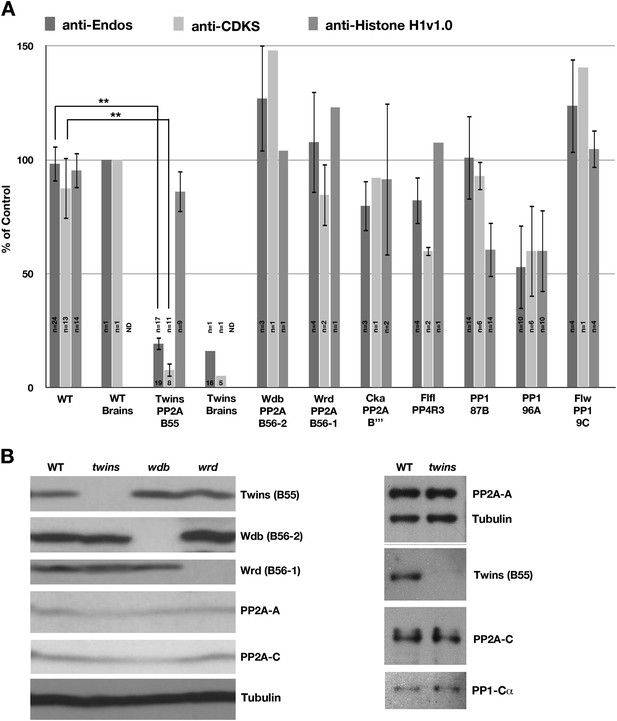
Drosophila larvae lacking B55 are deficient in anti-Endos.
(A) Extracts were prepared from larvae with null or strong loss-of-function alleles of the indicated genes, and assayed for anti-Endos, anti-CDKS, and anti-Histone H1v1.0 activities. Values were normalized for the total protein concentrations in the extracts; one replicate of the control assay (on a wild-type larva) was arbitrarily chosen as the 100% reference. Details about the genotypes involved are given in ‘Materials and methods’. The number of biological replicates for each genotype is presented inside the corresponding bar, with standard deviations shown. Levels of anti-Endos are much lower in twins (encoding the sole B55 subunit in Drosophila) larvae than in wild-type (WT) controls (p<10−20; Student’s two-tailed t test); this is also the case as expected for anti-CDKS (p<1010). As a control, phosphatase activity against Histone H1v1.10 is relatively unaffected in twins mutant larvae. Anti-Endos and Anti-CDKS activities were also severely compromised in extracts made from brains isolated from twins mutant animals. No consistent effects were observed in extracts made from larvae mutant for genes encoding the other phosphatase subunits indicated, with two exceptions. First, extracts from larvae mutant for PP1-96A displayed only about half the level of phosphatase activities measured with all three substrates. This is probably a systematic error caused by the ebony mutation in these animals, producing a dark pigment that caused an overestimation of the amount of total protein concentration. Second, the lower level of activity against Histone H1v1.0 in animals mutant for PP1-87B (the predominant PP1 catalytic subunit in Drosophila; Dombradi et al., 1990) is likely caused by the targeting of this substrate by the PP1-87B phosphatase. (B) Western blots of extracts from larvae mutant for genes encoding B55 (twins) and B56 (widerborst [wdb] and well-rounded [wrd]) regulatory subunits of PP2A. The blot at the right verifies that in twins mutants, the B55 protein is missing, while the PP2A-A and PP2A-C subunits are present in normal amounts.
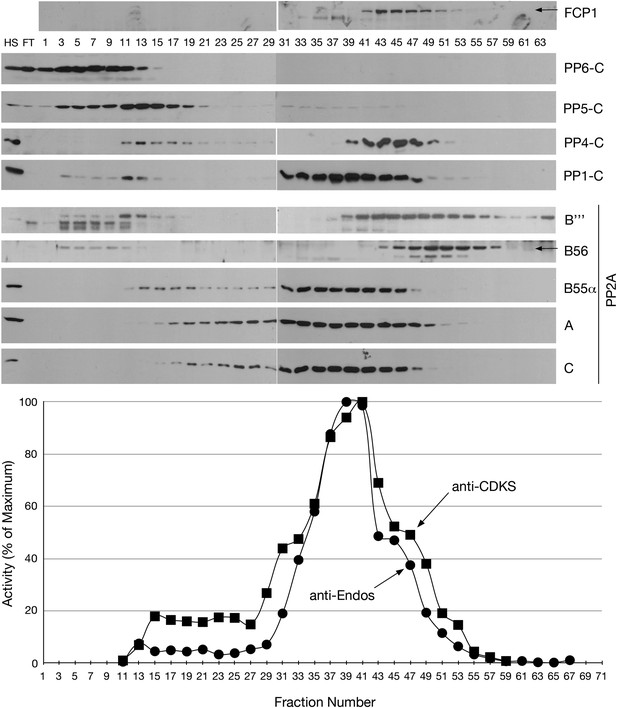
Mono-Q chromatography of HeLa extracts.
Total extract from asynchronous HeLa cells (high speed supernatant; HS) was applied to the column; FT is the flow-through. Proteins were eluted with linear gradient from 150 mM (Fraction 1) to 500 mM (Fraction 75) of NaCl. Individual fractions were assayed (n = 1 assay per data point) for anti-Endos (black circles) and anti-CDKS (black squares), and were also examined for the indicated phosphatase subunits by Western blot.
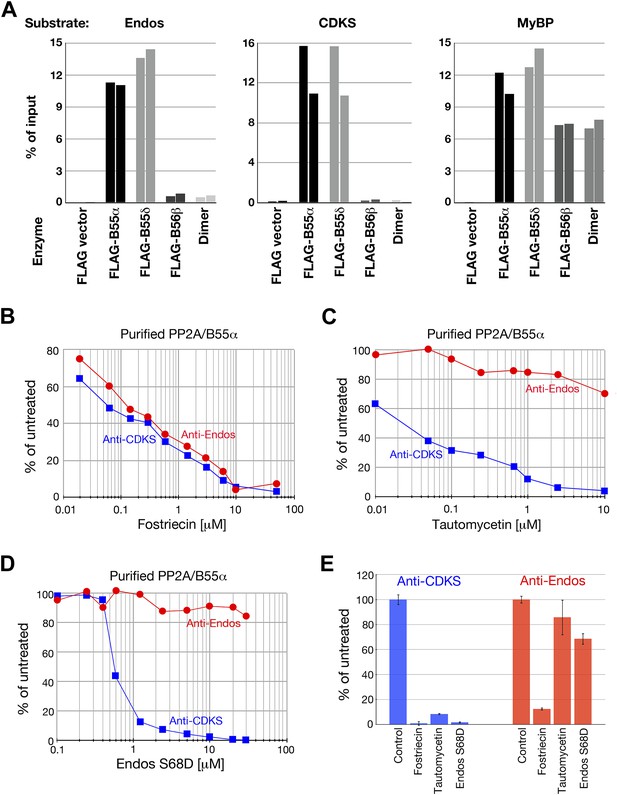
Anti-Endos activity of purified PP2A-B55.
(A) PP2A-B55 heterotrimers efficiently dephosphorylate Gwl-phosphorylated Endos. Each column represents an individual experiment (a biological replicate) in which the indicated enzyme preparations shown in Figure 6—figure supplement 1 (normalized for the amount of PP2A-C subunit where possible, or for the volume of transfected cells in the case of the vector-only control preparation) were assayed for anti-Endos activity, anti-CDKS activity, or generic activity against myelin basic protein (MyBP) phosphorylated with PKA. The y-axis indicates the percentage of radioactivity in the input substrate that was freed by enzyme treatment. The control preparation had no activity against any of these substrates, whereas PP2A-B55α and PP2A-B55δ heterotrimers showed similar strong levels of activity against all three substrates. PP2A-B56β heterotrimers and PP2A-A/C heterodimers, both of which dephosphorylated the generic MyBP substrate, failed to dephosphorylate either Gwl-phosphorylated pEndos or the pCDKS substrate. (B–D) Dose-response curves to phosphatase inhibitors (fostriecin, tautomycetin, and phosphomimetic Endos S68D as indicated) for the anti-Endos (red circles) and anti-CDKS (blue squares) activities of purified PP2A-B55α heterotrimers. Each point represents a single assay (n = 1). The anti-Endos activity of purified PP2A-B55α heterotrimers has the same characteristics as the predominant anti-Endos activity in whole cell extracts. In D, the fostriecin used has lost potency during the ∼6 months of storage after the experiments shown in Figure 2 were performed; fostriecin is well known to be somewhat labile in this time frame (Weiser et al., 2003). It is nonetheless clear that both the anti-Endos and anti-CDKS functions of PP2A-B555α display similar dose responses to this drug. (E) Technical replicates (n = 3) of untreated purified PP2A-B55 and enzyme treated with the maximal doses of the inhibitors shown in panels B–D (20 μM fostriecin, 10 μM tautomycetin, and 20 μM Endos S68D) were performed to assess reproducibility; symbols indicate average values and bars representing one standard deviation are shown.
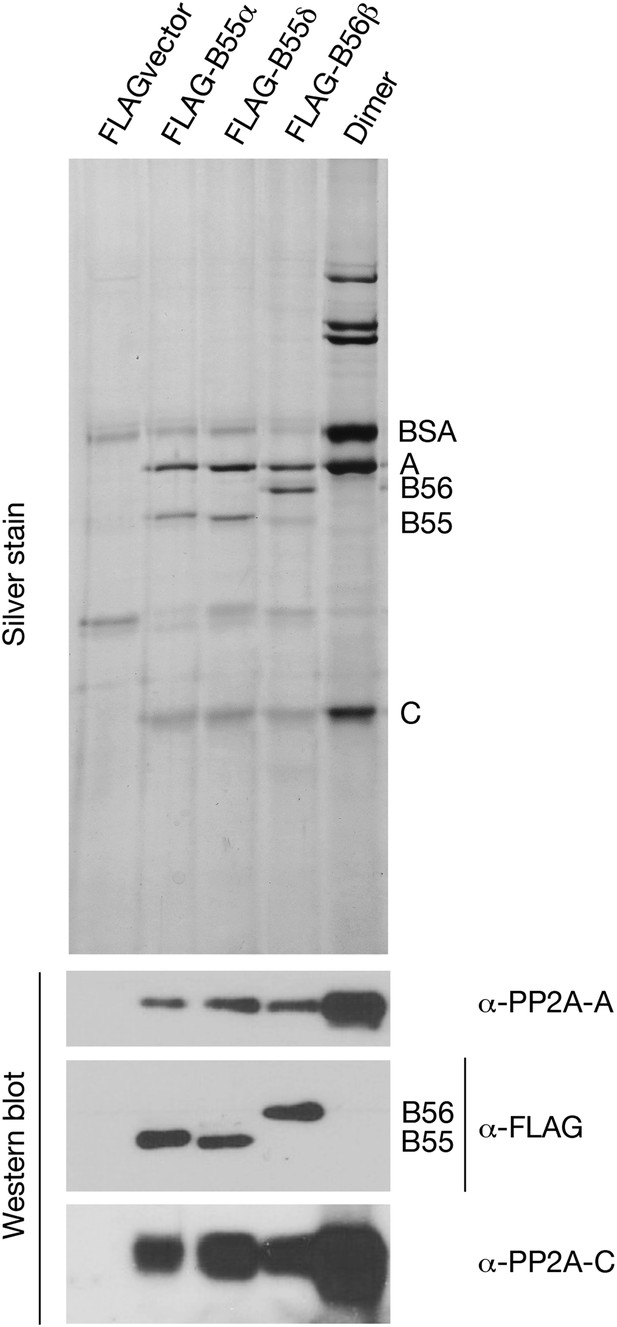
Preparations of PP2A heterodimer and heterotrimers.
In the first four lanes, HEK cells were transfected with the indicated constructs, and FLAG-tagged components were affinity purified as described in ‘Materials and methods’. Final preparations were fractionated by SDS-PAGE and analyzed by silver staining (top panel) and by Western blot (bottom panels). The major visible components in the silver staining are the A and C subunits of PP2A, the FLAG-tagged regulatory subunit whose expression construct was originally transfected (B55α, B55δ, or B56β), and bovine serum albumin (BSA) added to stabilize the purified enzymes. An additional band of variable intensity located between the B55 and C bands, which is also seen in the vector alone control lane, was identified by mass spectrometry as keratin; yet another weak band seen in some preparations that migrates just faster than B55α/δ appears to be immunoglobulin heavy chain from the anti-FLAG column. At the right is a sample of commercially obtained A–C heterodimer (Millipore 14-111).
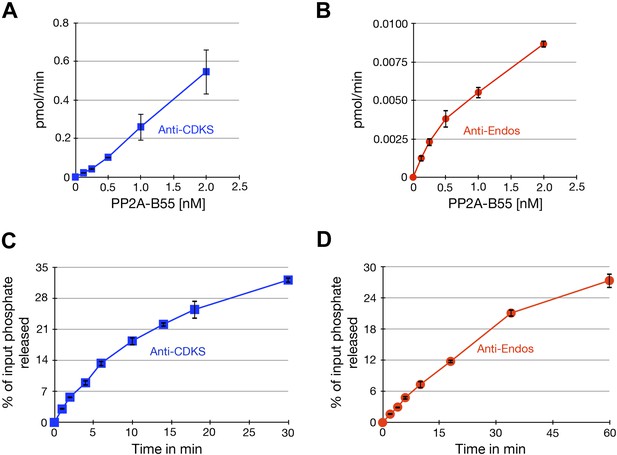
Characterization of phosphatase assays using purified PP2A-B55.
Panels A and B show the rate at which phosphate is released from labeled substrates as a function of the concentration of PP2A-B55. The relationships are approximately linear, although the rate of the anti-Endos reaction slows slightly at higher enzyme concentrations; for this reason, the concentration of PP2A-B55 was held to 0.5 nM or below in most experiments. Panels C and D show time courses of the same reactions; the concentration of PP2A-B55 was 0.5 nM. As expected, the reactions slow down as more than 20% of either substrate is depleted and as the reaction time becomes long enough for the PP2A-B55 to lose activity due to instability or degradation. For these reasons, kinetic constants were measured in experiments with incubations sufficiently short to ensure that the maximal conversion of substrate stayed below this level and to minimize loss of enzyme function. In all panels, data points represent the average of three technical replicates with error bars shown.
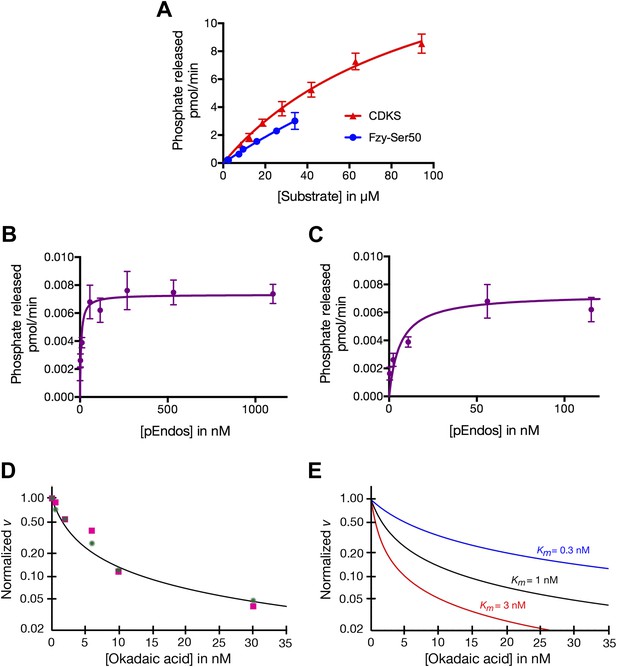
Determinations of the kinetic parameters shown in Table 1.
(A) Determination of kinetic parameters for the dephosphorylation of CDKS and Fzy-Ser50 substrates. One representative experiment of each is shown. Initial rates of dephosphosphorylation were determined with increasing substrate concentrations; the concentration of purified PP2A-B55 was 1 nM. The amount of 32P phosphate released was in all samples less than 20% of the input. For pCDKS substrate, n = 4; for pFzy-Ser50 substrate, n = 3 (technical replicates). The data were analyzed by non-linear regression analysis (using Prism 6 software) to determine Km and kcat values incorporated into the data shown in Table 1. Because we could not obtain sufficiently concentrated preparations of the Fzy-Ser50 substrate, the uncertainty in these parameters is very high, but the graphs illustrate that the behaviors of these two substrates are nonetheless much more similar to each other than either is to pEndos. (B and C) Determination of kinetic parameters for the dephosphorylation of pEndos from the initial reaction velocity as a function of substrate concentration. One representative experiment is shown. Initial rates of dephosphosphorylation were determined with increasing substrate concentrations. The concentration of purified PP2A-B55 was 0.5 nM; n = 4 technical replicates at each substrate concentration point. The amount of 32P phosphate released was in all samples less than 20% of the input. The data were analyzed by non-linear regression analysis as detailed in the ‘Materials and methods’ to determine Km and kcat values shown in Table 1. B and C graph the same data at different ranges of pEndos concentration. Note that in B, the rate of pEndos dephosphorylation remains constant near Vmax over a 20-fold range from 50 to 1100 nM, indicating that pEndos does not inhibit its own dephosphorylation. (D and E) Determination of kinetic parameters for the dephosphorylation of pEndos from competition with okadaic acid. Note the logarithmic scale on the y-axes. (D) Initial rates of pEndos dephosphorylation were determined in the presence of increasing concentrations of okadaic acid; one representative experiment is shown. The amount of 32P phosphate released was in all samples less than 20% of the input. Two independent experiments (technical replicates) are indicated with pink squares and green stars. The concentration of pEndos in this reaction was 50 nM, the concentration of purified PP2A-B55 was 0.25 nM, and the concentration of okadaic acid varied as shown. The data were fit by non-linear regression analysis to the mathematical model described in ‘Materials and methods’; calibration curves based on this model are shown in (E).
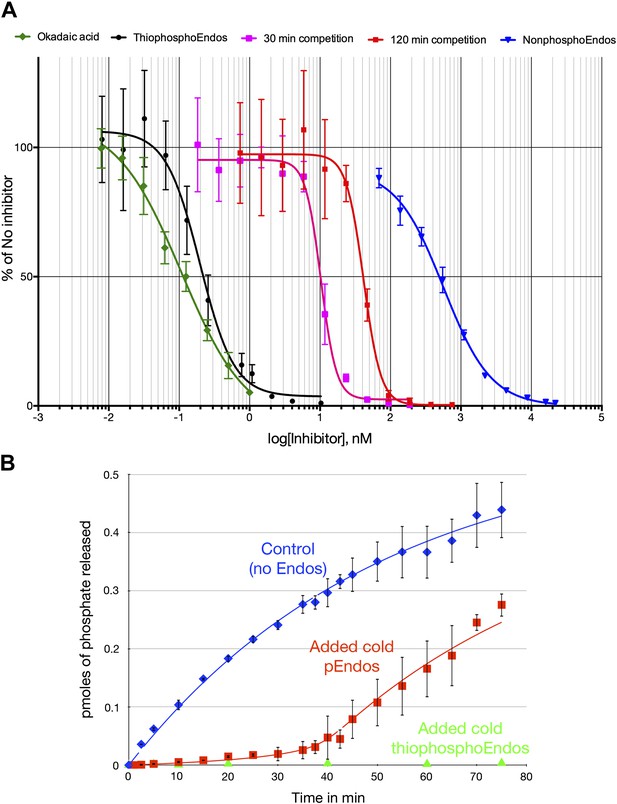
Dose-response curves for PP2A-B55 inhibitors.
(A) The ability of PP2A-B55 (at 0.125 nM) to dephosphorylate radioactive CDKS substrate (at 5 μM) was measured in the presence of varying amounts of the following inhibitors: okadaic acid (green), thiophosphorylated Endos (black), non-radioactive pEndos (during a 30-min incubation [pink] and a 120-min incubation [red]), and unphospohorylated Endos (blue). Thiophosphorylation by Gwl kinase makes Endos into a much stronger inhibitor of PP2A-B55 than unphosphorylated Endos; the affinity of thiophosphorylated Endos is only about twofold lower than that of okadaic acid. Non-radioactive pEndos is a less efficient inhibitor of PP2A-B55 than is thiophosphorylated Endos because the enzyme dephosphorylates pEndos during the course of incubation; the longer pEndos is exposed to the enzyme, the higher is the concentration of pEndos required to achieve the same degree of inhibition. For all points shown, n = 3 technical replicates. (B) Direct demonstration of the automatic reset mechanism that allows PP2A-B55 reactivation upon anaphase onset. PP2A-B55 at 0.25 nM was mixed together on ice with buffer in the absence of Endos (control) or in the presence of 16 nM unlabeled Gwl-phosphorylated Endos or Gwl-thiophosphorylated Endos and incubated on ice for 5 min; radioactive pCDKS substrate was then added to a final concentration of 0.47 μM; and the reaction was then transferred to 30°C at t = 0 and aliquots assayed for the release of 32P from the pCDKS substrate. Each point represents the average of three technical replicates (n = 3) with standard deviations shown. The dephosphorylation of pCDKS is suppressed until the majority of pEndos is inactivated by PP2A-B55-mediated dephosphorylation. (The gradual decrease in the slopes of the control and pEndos-added curves is probably due to instability and/or degradation of the PP2A-B55 during the extended time-course.) The control and cold pEndos data were fitted to curves calculated as described in ‘Materials and methods’; the best fit gave Km = 0.47 ± 0.14 nM and kcat = 0.03 s−1. The stronger inhibition caused by the thiophosphorylated pEndos (green triangles) is expected because its Kd (∼0.12 nM) is lower than the Km of pEndos.
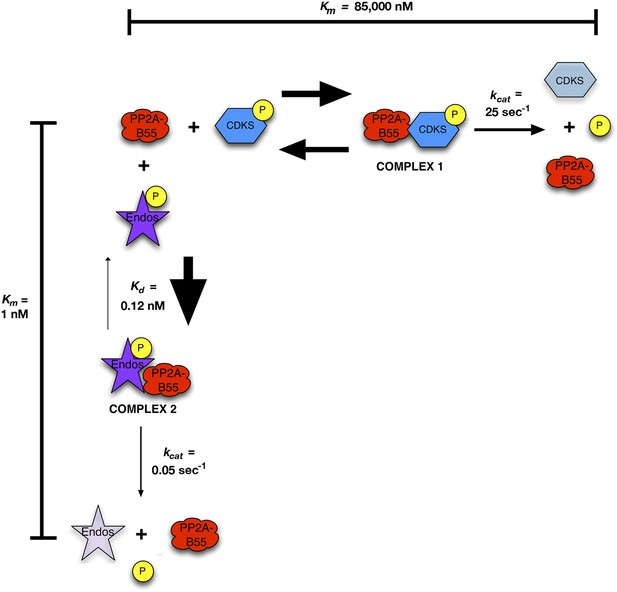
Inhibition by unfair competition.
In this model, pEndos and CDK-phosphorylated substrates compete for the active site of PP2A-B55. During M phase, pEndos is in stoichiometric excess of PP2A-B55, so due to the disparities in the kinetic constants of these substrates, almost all of the enzyme will accumulate as a tight-binding complex with pEndos. Although PP2A-B55 can slowly dephosphorylate pEndos, the inhibitor is replenished by action of Gwl until this kinase is inactivated at anaphase onset (not shown).
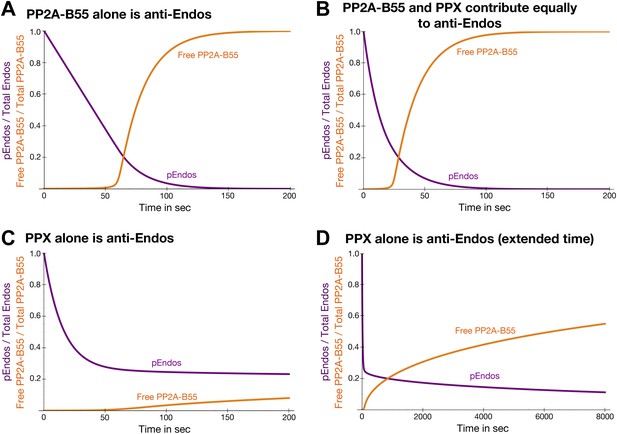
Theoretical time-course of pEndos dephosphorylation and PP2A/B55 activation at the end of M-phase.
These calculations make three assumptions: First, consistent with published data (Gharbi-Ayachi et al., 2010; Mochida et al., 2010), Endos was completely phosphorylated during M phase. Second, because the Km of PP2A-B55 for pEndos (∼1 nM, Table 1) is much smaller than the pEndos concentration (1 μM), essentially all the PP2A-B55 was bound to pEndos during M phase. Third, the Gwl phosphorylation of Endos stopped at the end of M-phase (t = 0) (Castilho et al., 2009; Mochida et al., 2009; Gharbi-Ayachi et al., 2010; Mochida et al., 2010). The curves show the fraction of Endos that remains phosphorylated (purple) and the fraction of PP2A-B55 that is released from pEndos sequestration (orange) as a function of time t in sec. (A) Scenario: PP2A-B55 is the only enzyme that can dephosphorylate pEndos. Parameter values estimated from the experimental data were used: Total PP2A/B55 concentration, 250 nM; initial total pEndos concentration, 1 μM; Km, 1 nM; and kcat, 0.05 s−1. (B) Scenario: pEndos is a target of both PP2A-B55 and equal amounts of PPX, a second phosphatase with parameters Km = 85 μM and kcat = 22.5 s−1 (based on the dephosphorylation of pCDKS by PP2A-B55 in Table 1). (C and D) Scenario: pEndos is only an inhibitor and not a substrate of PP2A-B55, and only PPX dephosphorylates pEndos. In this case, the Kd = 0.12 nM of thiophosphorylated Endos was used; this value was calculated from the IC50 for thiophosphorylated Endos from the dose-response curve in Figure 7 according to the equation described in Sasaki et al. (1994) and Takai et al. (1995). The time frame in D is an extension of that in C, showing that the time required for desequestration of 50% of PP2A-B55 activity would be ∼6200 s under this last scenario.
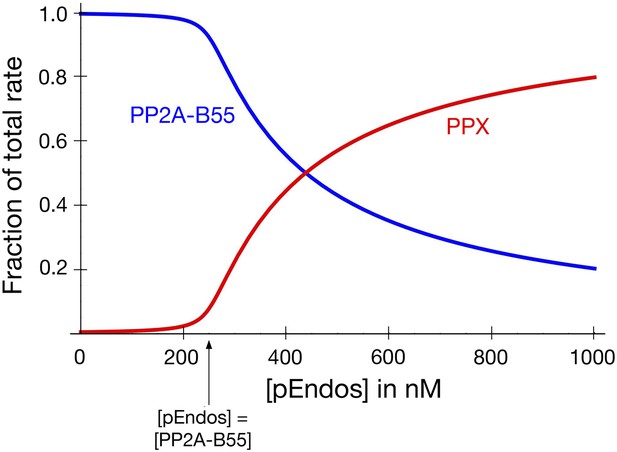
PP2A-B55 sequesters pEndos from the action of other phosphatases.
The graph models the scenario that cells contain an additional phosphatase PPX, present at the same concentration as PP2A-B55 (250 nM), which targets pEndos. The y-axis shows the fraction of the total initial rate of pEndos dephosphorylation contributed by the indicated enzyme (red for PPX; blue for PP2A-B55). The kinetic parameters used for PP2A-B55 and PPX were identical to those in Figure 9. When the concentration of pEndos is below the intracellular concentration of PP2A-B55, the contribution of PPX to pEndos dephosphorylation is negligible.
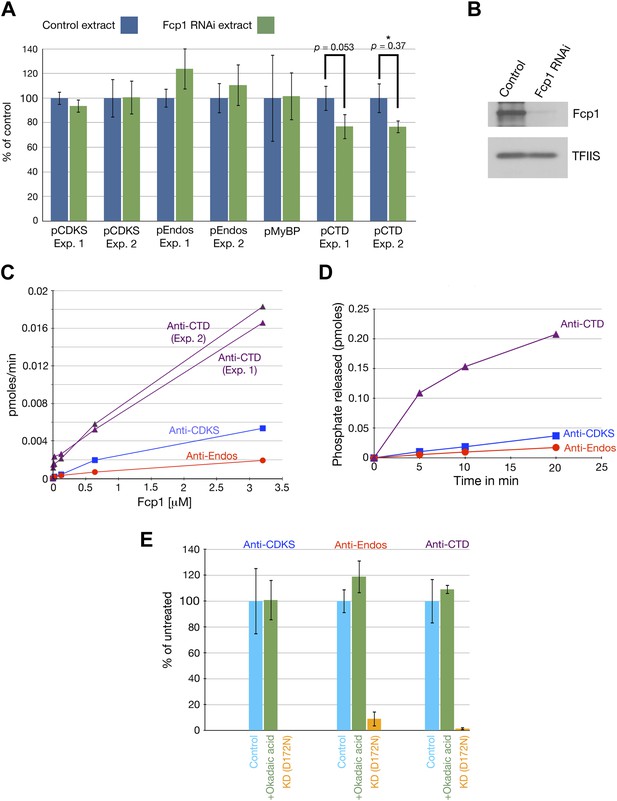
Fcp1 is not the anti-Endos phosphatase.
(A and B). Depletion of Fcp1 does not decrease anti-Endos activity. (A) Control extracts (made from S2 cells treated with a mock double-stranded RNA) or Fcp1 RNAi extracts (made from S2 cells treated with double-stranded RNA corresponding to the Drosophila Fcp1 gene [CG12252] were assayed for phosphatase activities using pCDKS, pEndos, and pCTD (the C-terminal domain of RNA polymerase II phosphorylated in vitro by mitogen-activated kinase 2 [MAPK2]) substrates. No significant differences were observed in terms of anti-CDKS or anti-Endos activities. Fcp1 depletion slightly decreases activity against the CTD substrate, consistent with the fact that Fcp1 is only one out of several known phosphatases known to target the CTD (Hsin and Manley, 2012). Each bar represents the average of three technical replicates with the standard deviation shown. (B) Western blot of the control and Fcp1 RNAi extracts assayed in part A, showing that more than 90% of the Fcp1 was removed by the treatment with Fcp1 double-stranded RNA. The loading control shows the signal revealed by antibody against the RNA polymerase II elongation factor TFIIS. (C and D). Purified S. pombe Fcp1 enzyme has minimal anti-Endos activity. Activities of purified Fcp1 against labeled pEndos, pCDKS, and pCTD. Each point represents a single assay. Note that the concentrations of Fcp1 employed in these experiments are in the micromolar range, as opposed to the subnanomolar-to-nanomolar concentrations of purified PP2A-B55 used in other figures. Consistent with previous reports for this enzyme (Hausmann and Shuman, 2002), the specific activity of Fcp1 against the CTD substrate is low (but much higher than that against pEndos). (E) The activities of purified wild-type Fcp1 against all three substrates are, as expected, resistant to high concentrations of okadaic acid (10 μM). A preparation of kinase-dead (KD) S. pombe Fcp1 with the D172N mutation (Suh et al., 2005) made in parallel has no activity against any of these three substrates.
Tables
Kinetic parameters of dephosphorylation by PP2A-B55
Substrate | Km (μM) | kcat (sec−1) | n* | Method† | Representative experiment |
---|---|---|---|---|---|
CDKS | 71–99 | 21–25 | 2 | v vs [S] | Figure 7A |
Fzy Ser50 | >100‡ | >15‡ | 1 | v vs [S] | Figure 7A |
pEndos | 0.0009–0.0017 | 0.021–0.035 | 2 | v vs [S] | Figure 7B,C |
0.018–0.066§ | 16§ | ||||
0.0004–0.0011 | 0.005–0.037 | 3 | OA competition | Figure 7D,E |
-
*
Number of independent experiments; each point in each experiment included 3–4 measurements.
-
†
v vs [S] experiments measured the initial rate of reaction as a function of substrate concentration; OA competition experiments involved measurements of pEndos dephosphorylation as a function of okadaic acid competition.
-
‡
Because sufficiently high concentrations of the Fzy Ser50 substrate were not obtained, non-linear regression analysis was inherently inaccurate in determining kinetic parameters. The values given are conservative lower limits.
-
§
The specific activities of multiple independent preparations of purified PP2A-B55 were measured at high pEndos substrate concentrations (5 μM) approximately 1000-fold in excess of the Km.