Kinetic regulation of kinesin’s two motor domains coordinates its stepping along microtubules
Figures
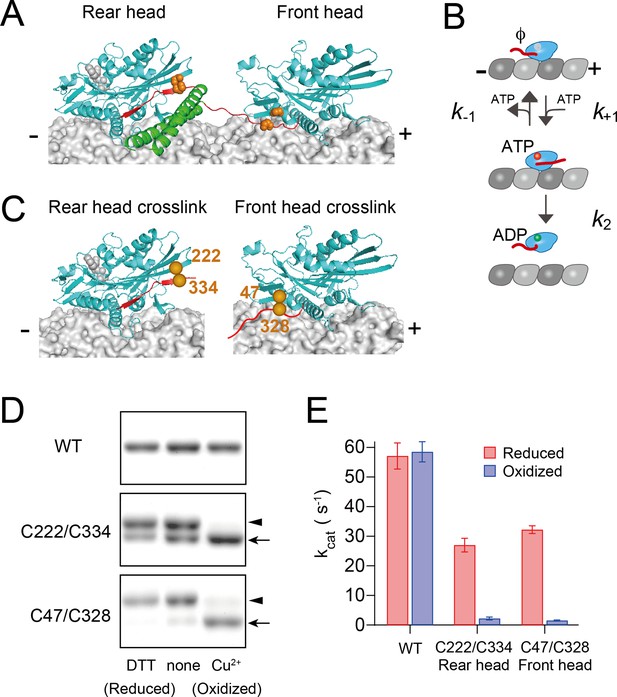
Disulfide-crosslinking to constraint the neck linker in either the forward- or backward-pointing configuration.
(A) Three-dimensional structure of kinesin dimer on the microtubule in the two-head-bound intermediate state (modeled based on PDB# 4HNA and 4LNU). The motor domain, neck linker, and neck coiled-coil are shown in cyan, red, and green. Orange spheres represent the positions of cysteine residues as described in panel (C). (B) Michaelis–Menten kinetics scheme for ATP-promoted dissociation of kinesin head from the microtubule, which includes ATP-binding (k+1), ATP dissociation (k−1), and ATP-induced microtubule dissociation (k2) steps. (C) Positions of the cysteine residues for disulfide-crosslinking of monomeric kinesin. Cys222 and Cys334 were introduced to constrain the neck linker in the forward, docked conformation (left), while Cys47 and Cys328 were introduced to constrain the neck linker in the backward-pointing configuration (right). (D) Disulfide-crosslinking after oxidative treatment was analyzed by non-reducing SDS–PAGE. WT (without Cys introduction), C222/C334, and C47/C328 constructs were treated with a reducing reagent (DTT), none, or oxidizing reagents (Cu2+). Arrowheads and arrows indicate un- and intramolecular-crosslinked kinesins, respectively. The whole image of the gels is shown in Figure 1—figure supplement 1. (E) Microtubule-activated ATPase under reduced and oxidized conditions. kcat and Km(MT) were determined from the fit to a Michaelis–Menten equation of ATP turnover rates at various microtubule concentrations (Figure 1—figure supplement 2). Mean ± SEM values were obtained from three independent assays.
-
Figure 1—source data 1
JPG files containing original gels for Figure 1D, indicating the relevant bands and treatments.
- https://cdn.elifesciences.org/articles/106228/elife-106228-fig1-data1-v1.zip
-
Figure 1—source data 2
Original files for SDS–PAGE displayed in Figure 1D.
- https://cdn.elifesciences.org/articles/106228/elife-106228-fig1-data2-v1.zip
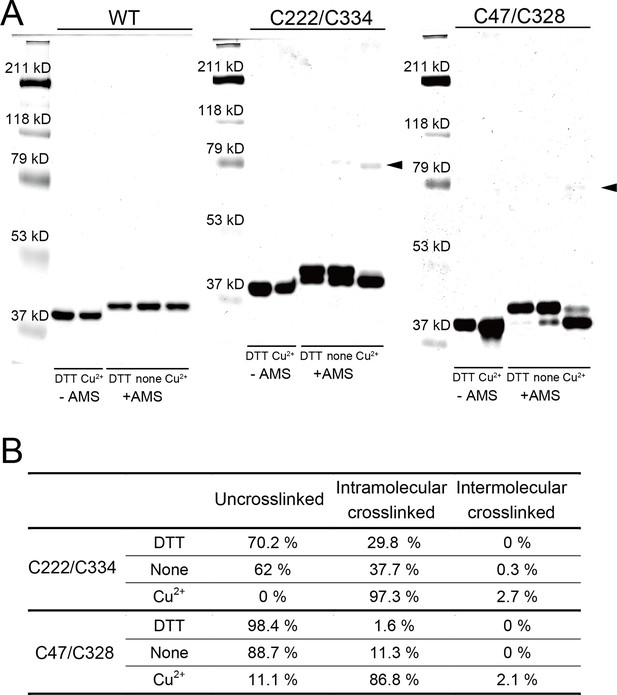
Estimation of disulfide-crosslinking efficiency.
(A) The whole gel image of the SDS–PAGE under non-reducing conditions with enhanced contrast to highlight the minor bands (close up of these gels are shown in Figure 1D). Cys-light K339 construct with no cysteine introduction (WT), with Cys222/Cys334 and with Cys47/Cys328 were treated with either reducing (10 mM DTT), oxidative (10 µM Cu2+ and 20 µM o-phenanthroline) conditions, or none (neither DTT nor Cu2+). 20 mM AMS, which covalently modifies free thiol residues, was added to enhance the band shift after crosslinking. Arrowheads indicate higher molecular weight bands (double the molecular weight of a monomer), corresponding to intermolecular disulfide crosslinked molecules. Cys-light K339 kinesin without cysteine introduction (WT) showed no band shift after oxidative treatment, confirming that crosslinking occurred only between substituted residues. (B) Ratio of the un-crosslinked, disulfide intramolecular-crosslinked, and disulfide intermolecular-crosslinked bands, as estimated from the intensities of the bands. Under oxidative conditions, un- and intermolecular-crosslinked species were minor and would not significantly affect the rate constants of the bulk measurements.
-
Figure 1—figure supplement 1—source data 1
JPG files containing original gels for Figure 1—figure supplement 1A, indicating the relevant bands and treatments.
- https://cdn.elifesciences.org/articles/106228/elife-106228-fig1-figsupp1-data1-v1.zip
-
Figure 1—figure supplement 1—source data 2
Original files for SDS–PAGE displayed in Figure 1—figure supplement 1A.
- https://cdn.elifesciences.org/articles/106228/elife-106228-fig1-figsupp1-data2-v1.zip
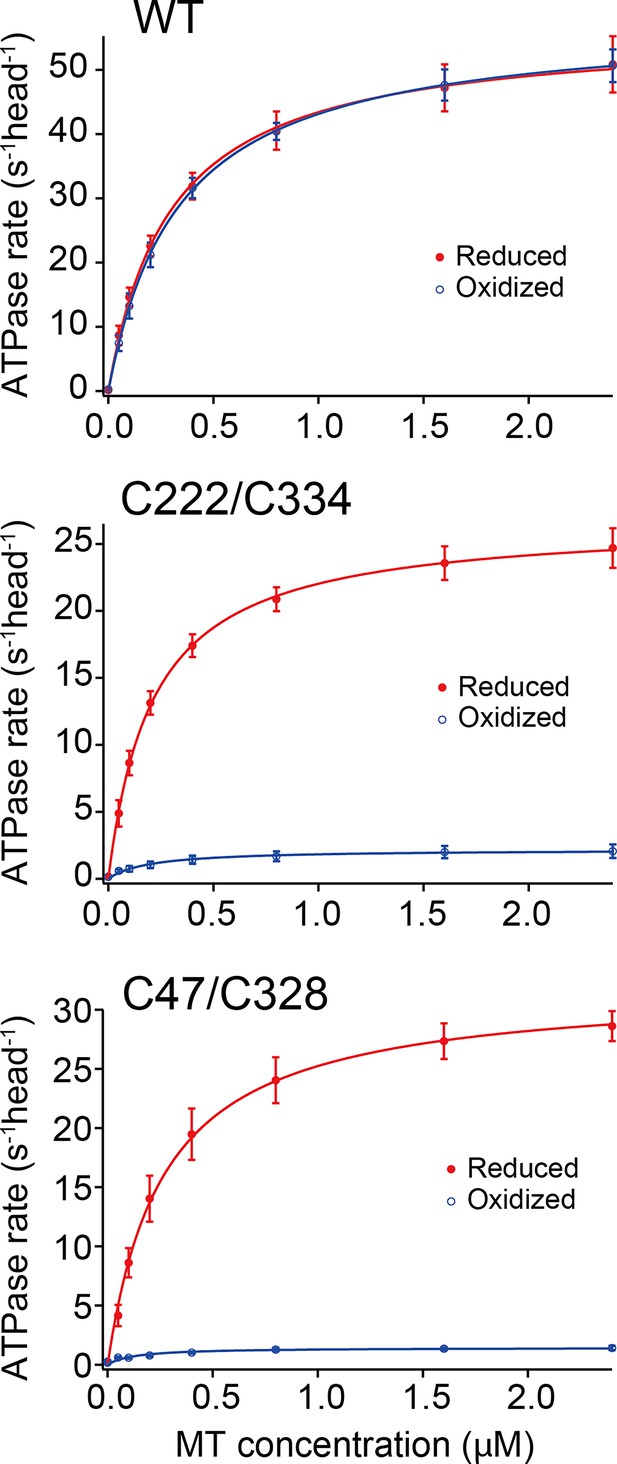
Steady-state microtubule-activated ATPase rates of monomeric kinesins before and after oxidative crosslinking.
These rates, measured under reducing and oxidizing conditions, were plotted against microtubule (MT) concentrations for monomeric kinesins with and without cysteine substitutions (N = 3; average ± SEM). The solid lines show fit with the Michaelis–Menten equation, and the fit parameters (kcat and Km(MT)) are summarized in Table 1.
-
Figure 1—figure supplement 2—source data 1
Excel file containing ATPase measurement data for Figure 1—figure supplement 2 and Figure 3—figure supplement 4A.
- https://cdn.elifesciences.org/articles/106228/elife-106228-fig1-figsupp2-data1-v1.xlsx
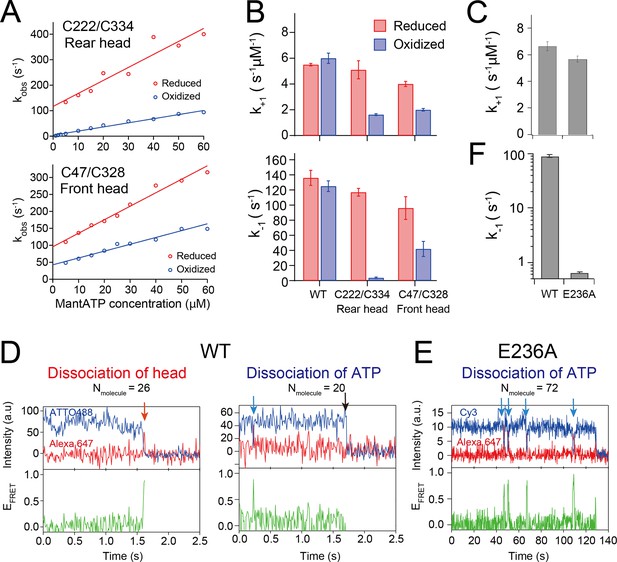
ATP-binding/dissociation kinetics of disulfide-crosslinked monomers.
(A) The rate of initial enhancement of the fluorescence after rapid mixing of kinesin–microtubule complex with mant-ATP (kobs; Figure 2—figure supplement 1) was plotted as a function of mant-ATP concentration. Solid lines represent a linear fit; the slope provides the on-rate of mant-ATP (k+1) and the off-set provides the off-rate of mant-ATP (k−1). (B) ATP-binding rate k+1 and ATP dissociation rate k−1 of monomers before and after crosslinking obtained from the fit shown in pane (A). Error bar shows the fit error. (C) Mant-dATP-binding rate k+1 to wild-type (WT) and E236A monomers (kobs plots are shown in Figure 2—figure supplement 2). (D) Two typical traces of single-molecule fluorescence resonance energy transfer (smFRET) between the donor-labeled wild-type monomer and Alexa-ATP (500 nM). Fluorescence intensities of donor (blue) and acceptor (red), and the calculated FRET efficiency (green) recorded at 100 fps are shown. Left trace: the FRET efficiency transiently increases just before both dyes disappear (indicated by the red arrow), suggesting that the nucleotide-bound head detached from the microtubule (Figure 2—figure supplement 4A). Right trace: the FRET efficiency transiently increases, followed by the recovery of donor fluorescent (shown by the blue arrow), indicating the reversible dissociation of ATP. The black arrow indicates the photobleaching of the donor dye. The numbers of molecules observed for each type is shown at the top of the traces. (E) Typical trace of smFRET between the E236A monomer and Alexa-ATP (200 nM) recorded at 5 fps, demonstrating repetitive ATP binding and dissociation. (F) The dissociation rate k−1 of Alexa-ATP from wild-type and E236A monomers (histograms are shown in Figure 2—figure supplement 4B). The value for the wild-type may be underestimated due to the limited temporal resolution (10 ms).
-
Figure 2—source data 1
Excel file containing kobs plot data of mant-ATP binding for Figure 2A and Figure 3—figure supplement 4B.
- https://cdn.elifesciences.org/articles/106228/elife-106228-fig2-data1-v1.xlsx
-
Figure 2—source data 2
Excel file containing fluorescent intensity time traces of donor and acceptor for Figure 2D, E.
- https://cdn.elifesciences.org/articles/106228/elife-106228-fig2-data2-v1.xlsx
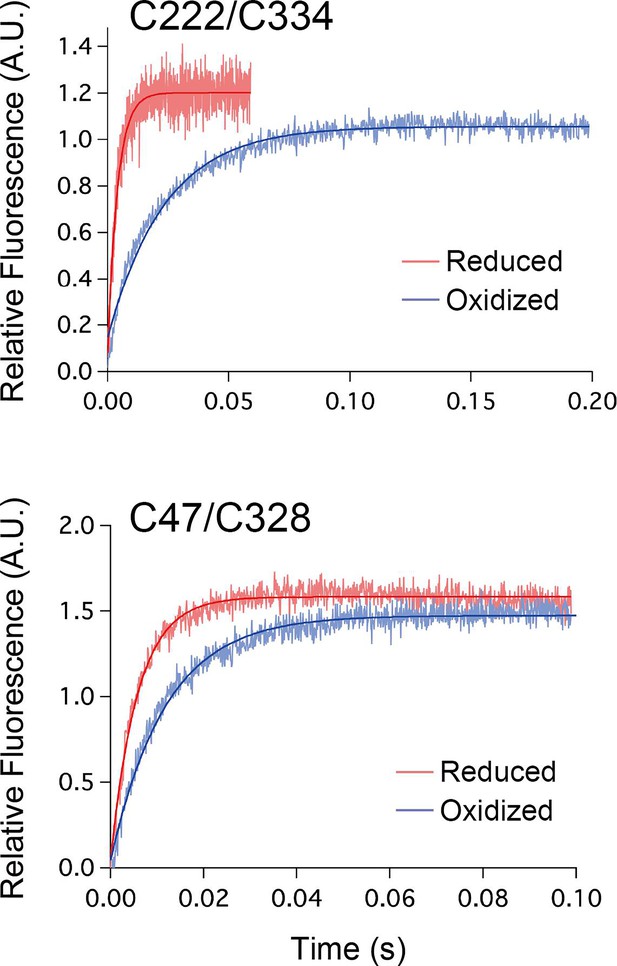
Pre-steady-state kinetics of ATP binding to monomeric kinesin–microtubule complex before and after crosslinking.
Examples of fluorescence signal time trace for C222/C334 and C47/C328 monomers bound to the microtubule under reduced (red) and oxidized (blue) conditions after rapid mixing with 20 µM mant-ATP. The solid lines represent the fit with a single exponential to determine the observed rate constants (kobs).
-
Figure 2—figure supplement 1—source data 1
Excel file containing mant-ATP-binding trace data for Figure 2—figure supplement 1.
- https://cdn.elifesciences.org/articles/106228/elife-106228-fig2-figsupp1-data1-v1.xlsx
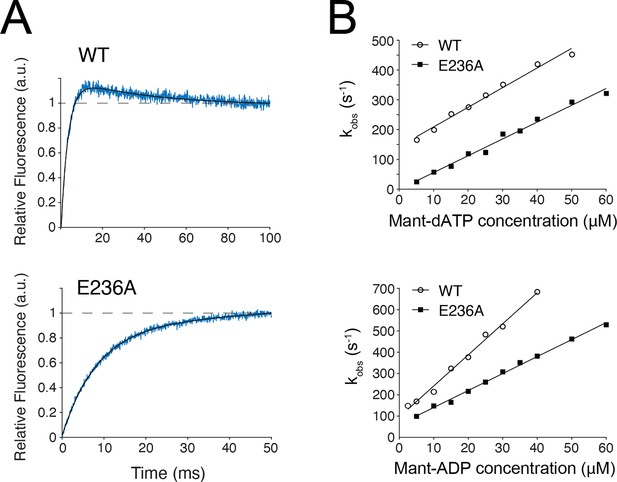
ATP-binding kinetics for E236A mutant monomer on microtubule.
(A) Examples of fluorescence signal time trace for microtubule-bound wild-type (WT) and E236A monomers (K349 without Cys-substitution) after rapid mixing with 20 µM mant-dATP. The WT monomer traces were fitted to a double exponential due to the presence of a decrease phase, previously reported by Ma and Taylor, 1997a. The E236A monomer traces were fitted to a burst equation (Equation 2 in Materials and Methods). (B) The kobs plot as a function of mant-dATP or mant-ADP concentrations. Solid lines represent a linear fit. The fit parameters for mant-dATP binding were: k+1 = 6.6 ± 0.3 μM-1s-1 and k-1 = 143 ± 9 s-1 for wild-type monomer, and k+1 = 5.6 ± 0.2 μM-1s-1 and k-1 = 0.6 ± 7.7 s-1 for E236A monomer. For mant-ADP binding: k+1 = 14.6 ± 0.5 μM-1s-1 and k-1 = 95 ± 12 s-1 for wild-type monomer, and k+1 = 8.0 ± 0.2 μM-1s-1 and k-1 = 61 ± 6 s-1 for E236A monomer. E236A showed a reduced ATP off-rate (k-1) while maintaining an ADP off-rate comparable to wild-type.
-
Figure 2—figure supplement 2—source data 1
Excel file containing mant-ATP-binding trace data for Figure 2—figure supplement 2A.
- https://cdn.elifesciences.org/articles/106228/elife-106228-fig2-figsupp2-data1-v1.xlsx
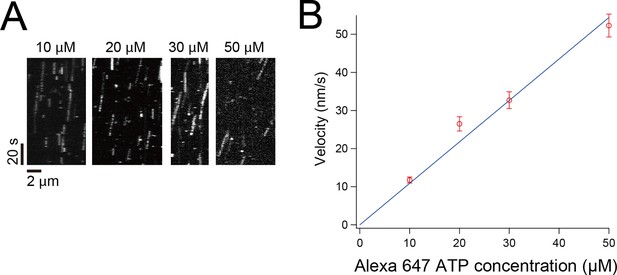
Processive motility of wild-type kinesin dimer driven by Alexa 647 ATP.
(A) Kymographs showing the motilities of the ATTO 488-labeled K490 dimeric kinesin in the presence of varying amounts of Alexa 647-conjugated ATP, recorded at 5 fps. (B) Mean velocities of the processive movement were plotted against Alexa 647-ATP concentrations. The solid line represents a fit with a linear line under the fixed zero intercept; the slope was 1.09 ± 0.05 nm/s/µM. The velocities were about 10-fold smaller than those observed in the presence of unmodified ATP (495 nm/s at 50 µM ATP; Isojima et al., 2016), suggesting that the Alexa-conjugated ATP binds to the head with lower affinity than unmodified ATP.
-
Figure 2—figure supplement 3—source data 1
Excel file containing single-molecule fluorescent motility data for Figure 2—figure supplement 3B.
- https://cdn.elifesciences.org/articles/106228/elife-106228-fig2-figsupp3-data1-v1.xls
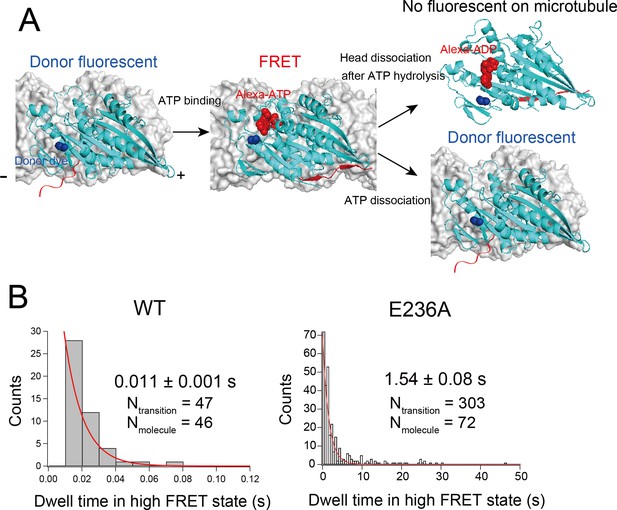
Dwell time of Alexa-ATP-bound state for wild-type and E236A monomers determined using single-molecule fluorescence resonance energy transfer (smFRET).
(A) Schematic showing the single-molecule FRET observation of ATP binding to the monomer head, observed using total-internal reflection fluorescent microscopy. The Cys55 (blue sphere) on Cys-light K349 monomeric kinesin, which is located close to the nucleotide pocket, was labeled with a donor fluorescent dye, and observed in the presence of the acceptor-labeled ATP (Alexa-ATP; red). The FRET efficiency increases up to ~90% when Alexa-ATP binds to the donor-labeled head on the microtubule. The donor and acceptor fluorescent both disappear when the head hydrolyses ATP and detaches from the microtubule. Conversely, the donor fluorescent recovers when the bound Alexa-ATP dissociates from the head reversibly. (B) Single-molecule FRET between ATTO488-labeled wild-type or Cy3-labeled E236A monomeric kinesin and Alexa 647 ATP was observed. Typical time traces are shown in Figure 2D, E. Histograms of the dwell time of the high FRET state were fit with a single exponential (red lines). The numbers represent the average dwell times (± SEM) determined from the fit. The high FRET state of the wild-type monomer includes head dissociation after ATP hydrolysis and reversible ATP dissociation. The inverse of the mean dwell time for wild-type and E236A monomers are 91.5 ± 4.7 and 0.65 ± 0.04 s–1, respectively (Figure 2F). The inverse of the mean dwell time for head dissociation and ATP dissociation for the wild-type were 89.7 ± 6.7 s–1 (N = 26) and 93.5 ± 9.4 s–1 (N = 20), respectively; they are nearly indistinguishable, in part because of limited temporal resolution (10 ms).
-
Figure 2—figure supplement 4—source data 1
Excel file containing single-molecule FRET histogram data for Figure 2—figure supplement 4B, Figure 4—figure supplement 3, and Figure 6—figure supplement 5B.
- https://cdn.elifesciences.org/articles/106228/elife-106228-fig2-figsupp4-data1-v1.xlsx
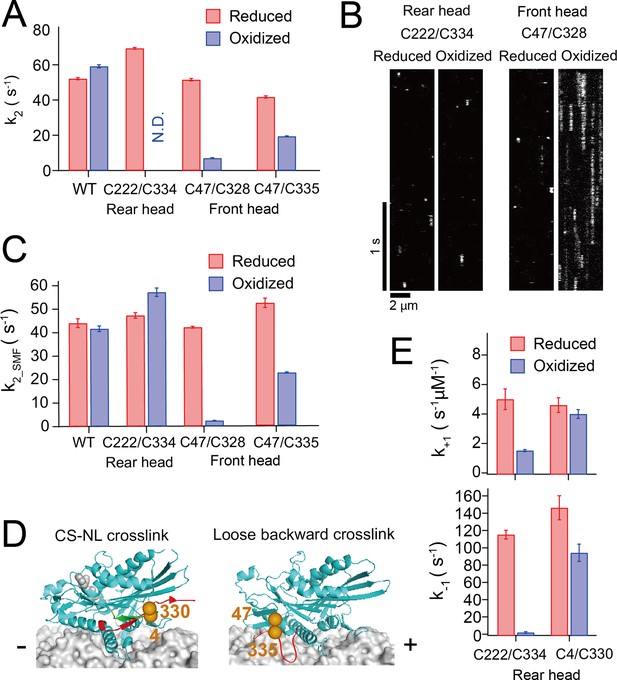
Microtubule-detachment kinetics of disulfide-crosslinked monomers.
(A) ATP-induced dissociation rate from microtubule k2 measured by turbidity change after the rapid mixing of kinesin-microtubule complex with 1 mM ATP. The turbidity time traces are shown in Figure 3—figure supplement 1. (B) Kymographs showing the binding and dissociation of GFP-fused kinesin under reduced and oxidized conditions on the microtubule. C47/C328 showed an extended dwell on the microtubule after crosslinking. (C) ATP-induced dissociation rate from the microtubule measured using single-molecule observations of GFP-fused kinesin. k2_SMF was determined as an inverse of the mean dwell time of the fluorescent kinesin on the microtubule in the presence of 1 mM ATP. The dwell time histograms are shown in Figure 3—figure supplement 2B. (D) Positions of Cys4 and Cys330 residues for disulfide-crosslinking of a monomer between the N-terminal cover strand (CS; green) and the neck linker (NL; red) (left). Positions of Cys47 and Cys335 residues for disulfide-crosslinking of a monomer to constrain the neck linker in the backward-pointing configuration allowing partial docking of the neck linker (right; Figure 3—figure supplement 3). The ATP-induced dissociation rates from the microtubule of C47/C335 monomer before and after crosslinking, measured by turbidity change (k2) and single-molecule fluorescence (k2_SMF), are shown in the figure (A, C). Histograms are displayed in Figure 3—figure supplement 4E. (E) Mant-ATP-binding rate k+1 and dissociation rate k−1 of C4/C330 monomer before and after crosslinking (data for C222/C334 are included from Figure 2B for comparison). The kobs plots are shown in Figure 3—figure supplement 4B.
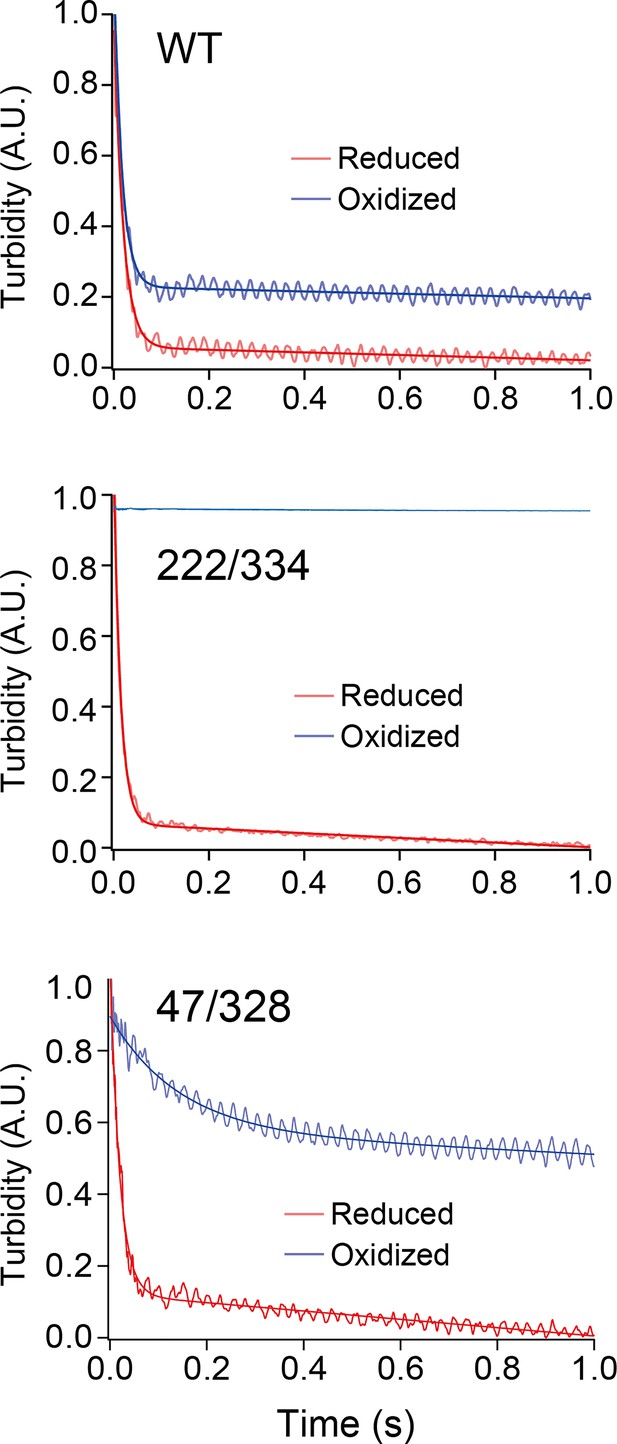
ATP-induced dissociation kinetics of crosslinked kinesin from microtubule measured using light scattering.
Turbidity (absorption at 340 nm) was monitored after rapidly mixing the kinesin–microtubule complex with 1 mM ATP and 150 mM KCl. The solid lines represent a fit to a burst equation (Equation 2 in Materials and methods). To prevent the motors from rebinding to the microtubule, we increased the KCl concentration of the kinesin–microtubule solution to 150 mM immediately before loading into the syringe. The C222/C334 did not show any initial rapid decrease in turbidity, presumably because the crosslinked C222/C334 has low microtubule affinity and would immediately dissociate from the microtubule after increasing the KCl concentration.
-
Figure 3—figure supplement 1—source data 1
Excel file containing light scattering trace data for Figure 3—figure supplement 1 and Figure 3—figure supplement 4C.
- https://cdn.elifesciences.org/articles/106228/elife-106228-fig3-figsupp1-data1-v1.xlsx
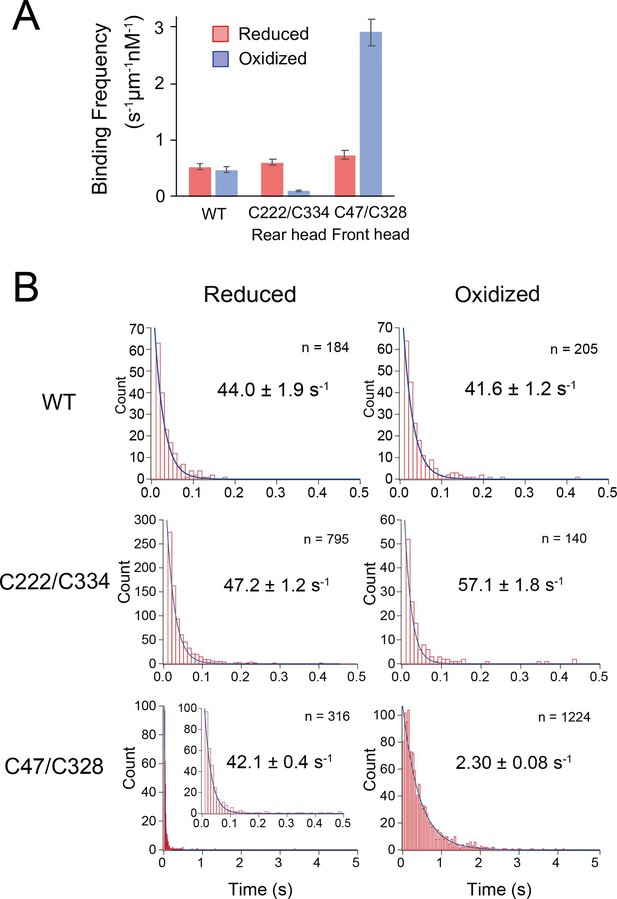
ATP-dependent microtubule-binding and -dissociation of crosslinked kinesins observed by single-molecule fluorescence microscopy.
(A) Frequency of binding of GFP-labeled kinesin to microtubule. The binding/unbinding of GFP-fused kinesin to/from microtubule was observed in the presence of 1 mM ATP and 100 mM KCl using total-internal reflection fluorescent microscopy (refer to Figure 3B for kymographs). Number of fluorescent spots were divided by time period (s), microtubule length (µm), and kinesin concentration (nM). The frequency decreased sixfold for C222/C328 and increased fourfold for C47/C328, demonstrating that forward and backward constraints alter the microtubule affinity in different ways. (B) Histograms of the dwell time of GFP-labeled kinesin on microtubule. Solid lines represent a fit to an exponential decay curve. The numbers represent the average dwell times (± SEM) determined from the fit.
-
Figure 3—figure supplement 2—source data 1
Excel file containing microtubule-binding frequency data for Figure 3—figure supplement 2A.
- https://cdn.elifesciences.org/articles/106228/elife-106228-fig3-figsupp2-data1-v1.xlsx
-
Figure 3—figure supplement 2—source data 2
Excel file containing microtubule-binding dwell time data for Figure 3—figure supplement 2B and Figure 3—figure supplement 4E.
- https://cdn.elifesciences.org/articles/106228/elife-106228-fig3-figsupp2-data2-v1.xlsx

Locations of the C47/C328 and C47/C335 residues when the substituted head is in the front or rear of dimeric kinesin.
The crosslinking between C47 (blue sphere) and C328 (red sphere) prevents the I325 residue (green sphere) from reaching the hydrophobic pocket on the head, as depicted in the front head. On the other hand, the crosslinking of C47 and C335 (red sphere) creates a flexible loop, which allows the initial segment of the neck linker, which includes the I325 residue, to dock onto the head as shown in the rear head.

Kinetics measurements of ATP binding and microtubule dissociation of C4/C330 and C47/C335 crosslinked monomeric kinesins.
Positions of cysteine residues for disulfide-crosslinking are shown in Figure 3D. The crosslinking efficiencies estimated by SDS–PAGE under non-reducing conditions were 92 and 85% for 4/330 and 47/335, respectively. Steady-state microtubule-activated ATPase rates (A), pre-steady-state mant-ATP-binding kinetics (B), pre-steady-state microtubule dissociation kinetics (C), kymographs of single-molecule fluorescence microscopy of GFP-fused monomers with 1 mM ATP and 100 mM KCl (D), and histograms of dwell time on microtubule (E), before and after oxidative crosslinking. The mean values and fit parameters are summarized in Table 1.
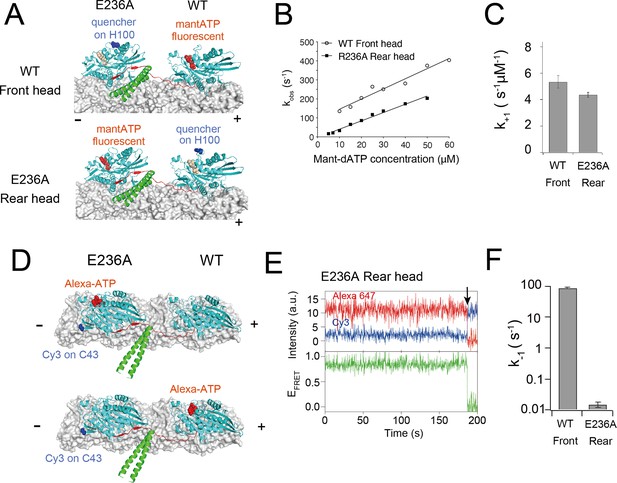
ATP-binding/dissociation kinetics of the E236A–WT heterodimer.
(A) Diagram showing the positions of H100 residue (highlighted in blue) labeled with Alexa 488 dye. This label quenches the mant-ATP signal of either the rear W236A head (upper; used to measure ATP binding to the front head) or the front wild-type head (lower; used to measure ATP binding to the rear head). (B) kobs plots for mant-ATP binding to the front wild-type and rear E236A heads of the E236A–WT heterodimer (typical fluorescent transients are shown in Figure 4—figure supplement 2). Solid lines indicate a linear fit. The fit parameters are k+1 = 5.3 ± 0.5 μM–1 s–1 and k−1 = 92 ± 16 s–1 for WT front head and k+1 = 4.4 ± 0.2 μM–1 s–1 and k−1 = −3.3 ± 5.1 s–1 for E236A rear head. (C) ATP-binding rate (k+1) for front and rear heads of the E236A–WT heterodimer, as determined by fitting in panel (B). (D) Diagram showing the position of Cys43 residue of E236A chain for labeling with donor (Cy3; blue) fluorophore, and the Alexa 647 ATP (red) bound to the rear E236A head (upper) or the front wild-type head (lower) of the E236A–WT heterodimer. High FRET efficiency is expected when the Alexa-ATP binds specifically to the rear E236A head. (E) A representative trace of fluorescence intensities of donor (Cy3; blue) on the E236A rear head and acceptor (Alexa 647; red) fluorophores, with calculated FRET efficiency (green), for the E236A–WT heterodimer at 200 nM Alexa-ATP recorded at 5 fps. The black arrow indicates the dissociation of Alexa-ATP, accompanied by the recovery of donor fluorescent. ATP remained bound to the rear head significantly longer compared to the E236A monomer (Figure 2E). (F) ATP dissociation rate (k−1) for front and rear heads of the E236A–WT heterodimer. Note that these rates were measured using different methods (stopped flow for the WT font head (B) and single-molecule fluorescence resonance energy transfer (smFRET) for the E236A rear head (E)) and thus cannot be directly compared.
-
Figure 4—source data 1
Excel file containing kobs plot data of mant-ATP binding for Figure 4B and Figure 2—figure supplement 2B.
- https://cdn.elifesciences.org/articles/106228/elife-106228-fig4-data1-v1.xlsx
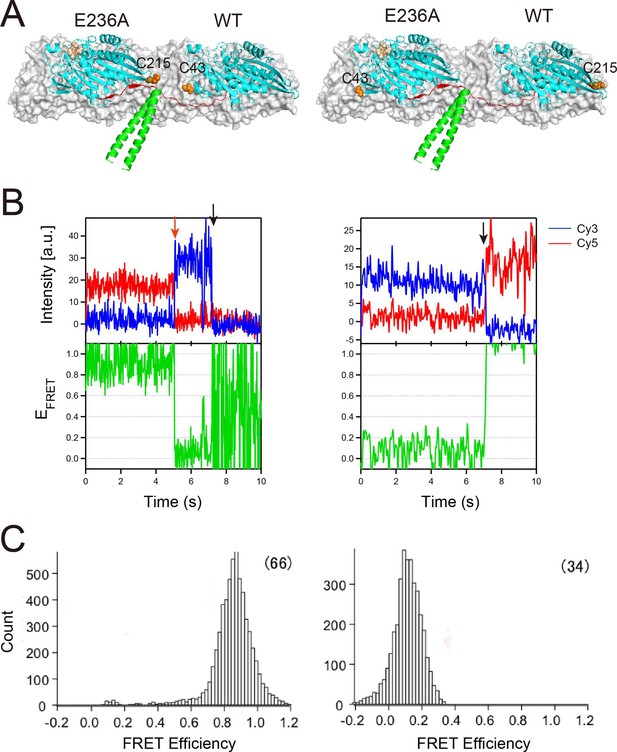
Single-molecule FRET between two heads of E236A–WT heterodimer.
(A) Schematic diagrams showing the positions of introduced cysteines (orange spheres) for labeling with donor/acceptor fluorophores; left; 215Cys on E236A-chain and 43Cys on WT-chain, right; 43Cys on E236A-chain and 215Cys on WT-chain. When the E236A head and WT head occupy the trailing and leading positions, respectively, the distances between these two cysteine residues are ~3 and ~13 nm. (B) Typical traces of fluorescence intensities of donor (Cy3, blue) and acceptor (Cy5, red) fluorophores, as well as the calculated FRET efficiency (EFRET), for 215-43 labeled E236A–WT heterodimers, in the presence of 200 nM ATP at 50 fps. The red arrow indicates the photobleaching of the acceptor dye, while the black arrows indicate the photobleaching of the donor dye. We were unable to detect a transient FRET efficiency change associated with head detachment (toward ~30% FRET, or the one-head-bound state) at this temporal resolution, which requires measurements with a higher temporal resolution (Figure 4). (C) Histograms of FRET efficiencies (for each frame of images) of donor and acceptor labeled E236A–WT heterodimer, observed in the presence of 200 nM ATP. The number of molecules analyzed is shown in parentheses.
-
Figure 4—figure supplement 1—source data 1
Excel file containing fluorescent intensity time traces of donor and acceptor for Figure 4—figure supplement 1B.
- https://cdn.elifesciences.org/articles/106228/elife-106228-fig4-figsupp1-data1-v1.xlsx
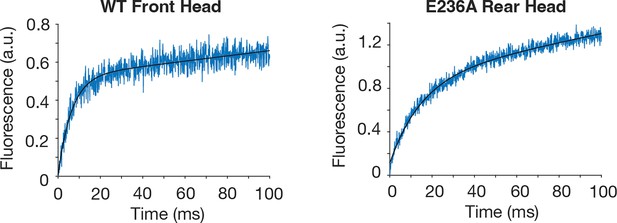
ATP-binding kinetics for front and rear heads of E236A–WT heterodimer.
Examples of fluorescence signal time trace for WT front head and E236A rear heads of E236A–WT heterodimer after rapid mixing with 15 µM mant-dATP. The traces were fitted to a burst equation. The kobs plots are shown in Figure 4B.
-
Figure 4—figure supplement 2—source data 1
Excel file containing mant-ATP-binding trace data for Figure 4—figure supplement 2.
- https://cdn.elifesciences.org/articles/106228/elife-106228-fig4-figsupp2-data1-v1.xlsx
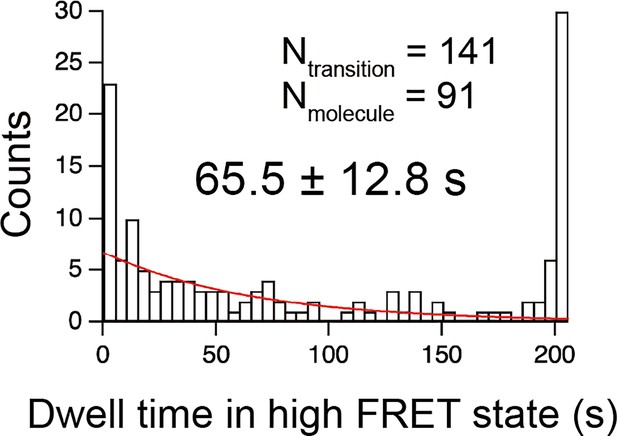
Single-molecule FRET observation between donor dye on the E236A head of E236A–WT heterodimer and acceptor-labeled ATP.
Histogram of the dwell time of the high FRET state is shown. Typical time trace of the FRET efficiency is shown in Figure 4E. The solid lines show fit with an exponential decay (red lines). The number represents the average dwell times (± SEM) determined from the fit. The inverse of the mean dwell time k−1 is 0.015 ± 0.003 s–1 (Figure 4F).
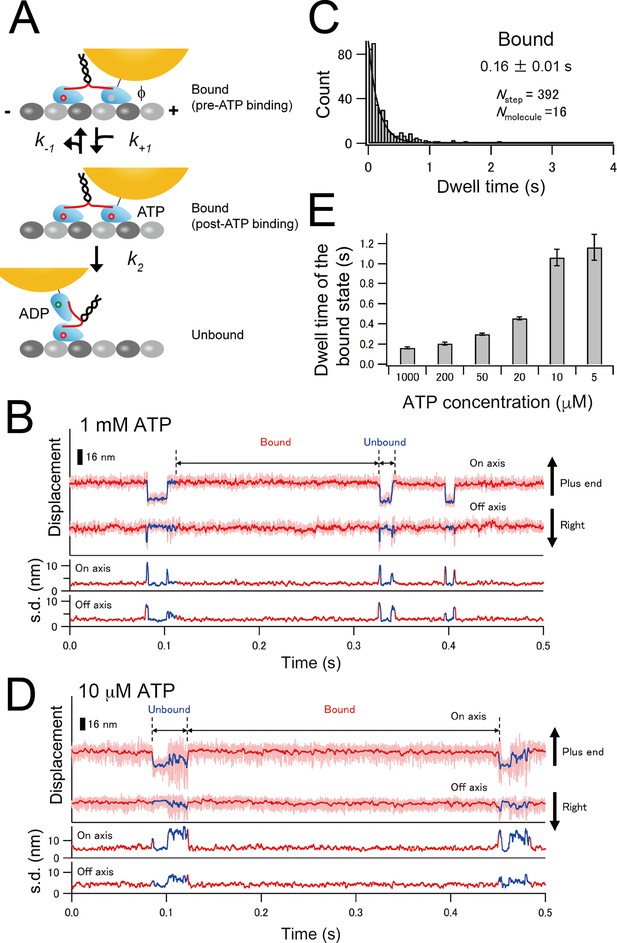
Microtubule-detachment kinetics of the front WT head of E236A–WT heterodimer.
(A) A diagram illustrating the kinetic transitions for the detachment of the gold-labeled WT head of the E236A–WT heterodimer. Upon binding to the microtubule, the front head releases ADP, becoming a nucleotide-free state (indicated as ϕ; pre-ATP binding). The detachment kinetics from this state can be described using the same scheme as the monomer (Figure 1B). (B) Typical trace for the centroid positions of the gold probe attached to the WT head of the E236A–WT heterodimer in the presence of 1 mM ATP (light red lines), toward the microtubule long axis (on axis) and perpendicular to the microtubule axis (off axis). Red and blue lines depict the median-filtered trace (with a window size of 51 frames) for the bound and unbound states, respectively. Lower panels display the standard deviation (s.d.) of on- and off-axis positions for each time frame t (calculated as [t – 20, t + 20]). (C) Histogram of the dwell time in the bound state. The solid line shows the fit with an exponential function. The number represents the average dwell time (± SEM) determined from the fit. (D) Typical trace for the centroid positions of the gold probe attached to the WT head of E236A–WT heterodimer in the presence of 10 µM ATP. (E) Mean dwell times in the bound state under various ATP concentrations as determined from the fit of the histograms of the dwell times (Figure 5—figure supplement 3).
-
Figure 5—source data 1
Excel file containing 2D time traces of centroid position of gold probe for Figures 5B, D, 6C, Figure 5—figure supplement 1, and Figure 6—figure supplement 4A.
- https://cdn.elifesciences.org/articles/106228/elife-106228-fig5-data1-v1.xlsx
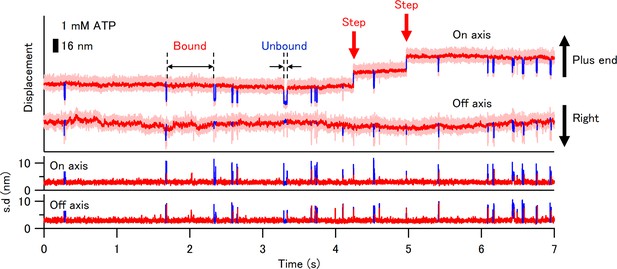
Long-term trace of the gold-labeled E236A–WT heterodimer exhibiting very slow stepping motion.
A typical trace of the gold-labeled WT head of the E236A–WT heterodimer in the presence of 1 mM ATP recorded at 20,000 fps for 7 s. The detached WT head mostly rebound to the same tubulin-binding site without exhibiting a step. However, the head occasionally bound to the 16 nm forward binding site (indicated by red arrows; forward-step), which happened about once every 10 s (similar to the ATP hydrolysis rate of the E236A head). Upon unbinding, the detached WT head displaced toward the plus-end of the microtubule before stepping forward, which suggests that the WT head was in the trailing position before detachment. On the other hand, in the case of the rebinding, the detached head displaces toward the minus end of the microtubule, suggesting that the WT head was in the leading position before it detaches (refer to Figure 5A). If the trailing head detaches and rebinds to the same binding site, the displacement upon unbinding would be toward the plus-end (as seen in Figure 5a in Isojima et al., 2016).
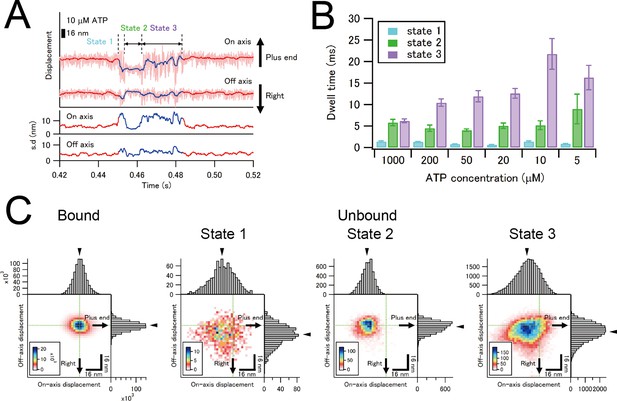
The unbound state of the gold-labeled WT head of the E236A–WT heterodimer.
(A) A close-up view of the unbound state in the typical trace of gold-labeled WT head observed in the presence of 10 µM ATP (Figure 5D). This state is interrupted by a state of reduced fluctuation, which was not observed in the ‘trailing’ head of the wild-type dimer as reported in Isojima et al., 2016. We refer to the initial high standard deviation (s.d.), second low s.d., and the last high s.d. states as states 1, 2, and 3. (B) The duration of each state in the unbound state. The dwell times for states 1 and 2 remain independent of ATP concentration (1.1 and 5.6 ms on average, respectively), whereas the dwell time for state 3 weakly depends on ATP concentration and is longer than those of states 1 and 2. (C) The ensemble-averaged 2D histograms of the bound and unbound states of the gold-labeled WT head. Green lines for unbound states display the average on- and off-axis positions during the preceding bound state (representing the mean positions for the bound state). Arrowheads indicate the average position for the projected 1D distributions. The fluctuation in the position of unbound state 2 is suppressed, much like the bound state, suggesting that the motility of the detached head is temporarily restrained. In contrast, the unbound state of G7 and G12 mutants with flexible insertions did not display the initial confined state (Figure 6C), suggesting that the confinement does not result from direct interaction between heads or between the head and the microtubule, but presumably rather from confinement on the neck linker of the unbound head. We propose that the detached front head initially exists in the ADP-bound/closed state (Sindelar et al., 2002; Figure 7), and its neck-linker docks onto the head after microtubule detachment (transitioning from states 1 to 2). In this state, both neck linkers are docked onto the head, and as a result, the mobility of the unbound head is limited due to the restricted degree of freedom in the neck linkers. Finally, the neck linker of the unbound head undocks, transitioning into the highly mobile state 3 (ADP-bound/semi-open state), which allows the unbound head to bind to the tubulin-binding site and release ADP.
-
Figure 5—figure supplement 2—source data 1
Excel file containing dwell time histogram data of unbound state for Figure 5—figure supplement 2B.
- https://cdn.elifesciences.org/articles/106228/elife-106228-fig5-figsupp2-data1-v1.xlsx
-
Figure 5—figure supplement 2—source data 2
Excel file containing 2D histogram data of unbound state for Figure 5—figure supplement 2C.
- https://cdn.elifesciences.org/articles/106228/elife-106228-fig5-figsupp2-data2-v1.xlsx
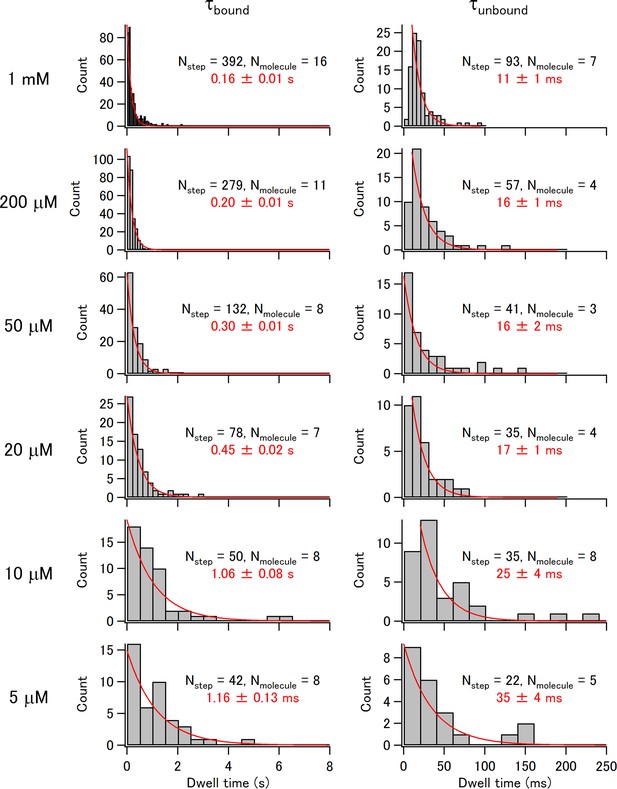
Distributions of the dwell time in the bound and unbound states of the leading WT head of E236A–WT heterodimer.
Histograms of the dwell time in the bound (τ bound) and unbound (τ unbound) states of the gold-labeled WT head of the E236A–WT heterodimer at various ATP concentrations. Red lines indicate the fit with exponential decay. The average dwell times (± SEM), as determined from the fits, are shown in red numbers and those of the bound state are summarized in Figure 5E.
-
Figure 5—figure supplement 3—source data 1
Excel file containing dwell time histogram data of E236A–WT heterodimer for Figure 5—figure supplement 3 and Figure 6—figure supplement 2, Figure 6—figure supplement 3 and Figure 6—figure supplement 4C.
- https://cdn.elifesciences.org/articles/106228/elife-106228-fig5-figsupp3-data1-v1.xlsx
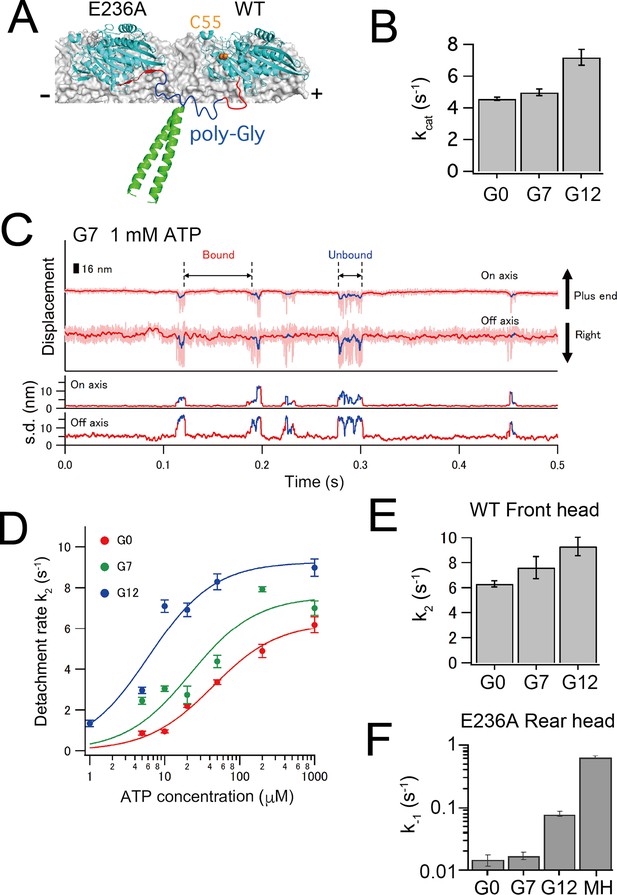
Effect of the tension applied to the neck linker on the gating examined using the neck-linker extended E236A–WT heterodimer.
(A) Diagram showing the position of the poly-Gly residues (blue) inserted between the neck linker (red) and the neck coiled-coil (green). The Cys55 residue for gold labeling is represented by an orange sphere. (B) kcat for the microtubule-activated ATPase of E236A–WT heterodimers without (termed as G0) and with neck-linker extensions (G7 and G12) (Figure 6—figure supplement 1). (C) Typical trace for the centroid positions of the gold probe attached to the WT head of the E236A–WT heterodimer with a 7 poly-Gly insertion in the presence of 1 mM ATP. (D) The inverse of the mean dwell time in the bound state was plotted as a function of ATP concentration, as determined from the fit of the histograms of the dwell times (Figure 6—figure supplements 2 and 3). Error bars represent SEM. The solid lines show the fit with the Michaelis–Menten equation. The fit parameters are kcat (or k2) = 6.3 ± 0.2 s–1 and Km (ATP) = 43 ± 6 µM for G0, kcat = 7.6 ± 0.9 s–1 and Km (ATP) = 22 ± 10 µM for G7, and kcat = 9.3 ± 0.7 s–1 and Km (ATP) = 6.1 ± 2.1 µM for G12. The Km (ATP) decreases as the insertion length increases. (E) The kcat value, which represents the ATP-induced detachment rate of the front head k2, obtained from the fit shown in panel D. The error bars represent SEM. (F) The ATP dissociation rates k−1 for the E236A rear head of the wild-type (G0), G7, and G12 E236A–WT heterodimer were determined using single-molecule fluorescence resonance energy transfer (smFRET). The k−1 was calculated as the inverse of the mean dwell time for the high FRET state (see the typical trace and dwell time histograms for the G7 and G12 heterodimers in Figure 6—figure supplement 5). For comparison, data for the E236A monomer head (referred to as MH) is included from Figure 2F.
-
Figure 6—source data 1
Excel file containing microtubule-detachment rate data for Figure 6D.
- https://cdn.elifesciences.org/articles/106228/elife-106228-fig6-data1-v1.xlsx

Microtubule-activated ATPase rates of the E236A–WT heterodimer with and without neck-linker extension.
ATPase rates measured for E236A–WT heterodimer without neck-linker extension (termed G0), and with neck-linker extension of 7 (G7) or 12 poly-Gly insertion (G12) were plotted against microtubule (MT) concentrations. The solid lines represent the fit with the Michaelis–Menten equation. The fit parameters are kcat = 4.6 ± 0.1 ATP/s per head and Km (MT) = 0.54 ± 0.04 µM for G0, kcat = 5.0 ± 0.2 ATP/s per head and Km (MT) = 0.18 ± 0.03 µM for G7, and kcat = 7.2 ± 0.5 ATP/s per head and Km (MT) = 0.14 ± 0.03 µM for G12.
-
Figure 6—figure supplement 1—source data 1
Excel file containing ATPase measurement data for Figure 6—figure supplement 1.
- https://cdn.elifesciences.org/articles/106228/elife-106228-fig6-figsupp1-data1-v1.xlsx
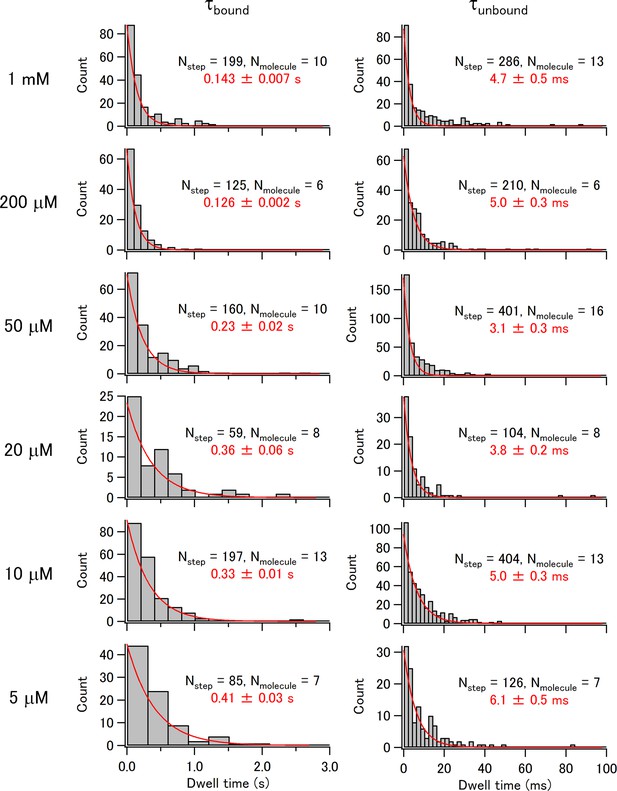
Distributions of the dwell time in the bound and unbound states of the leading WT head of E236A–WT heterodimer with 7 poly-Gly insertion (G7).
Histograms of the dwell time in the bound (τ bound) and unbound (τ unbound) states of the gold-labeled WT head of the E236A–WT heterodimer at various ATP concentrations. Red lines indicate the fit with exponential decay. The average dwell times (± SEM), as determined from the fits, are shown in red numbers. The inverse of the mean dwell time in the bound state k2 is summarized in Figure 6D.
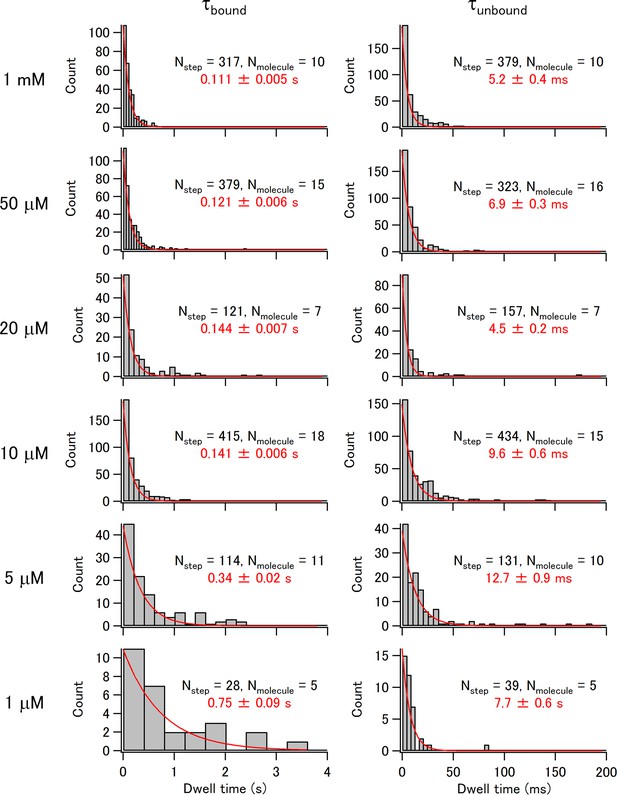
Distributions of the dwell time in the bound and unbound states of the leading WT head of E236A–WT heterodimer with 12 poly-Gly insertion (G12).
Histograms of the dwell time in the bound (τ bound) and unbound (τ unbound) states of the gold-labeled WT head of the E236A–WT heterodimer at various ATP concentrations. Red lines indicate the fit with exponential decay. The average dwell times (± SEM), as determined from the fits, are shown in red numbers. The inverse of the mean dwell time in the bound state k2 is summarized in Figure 6D.
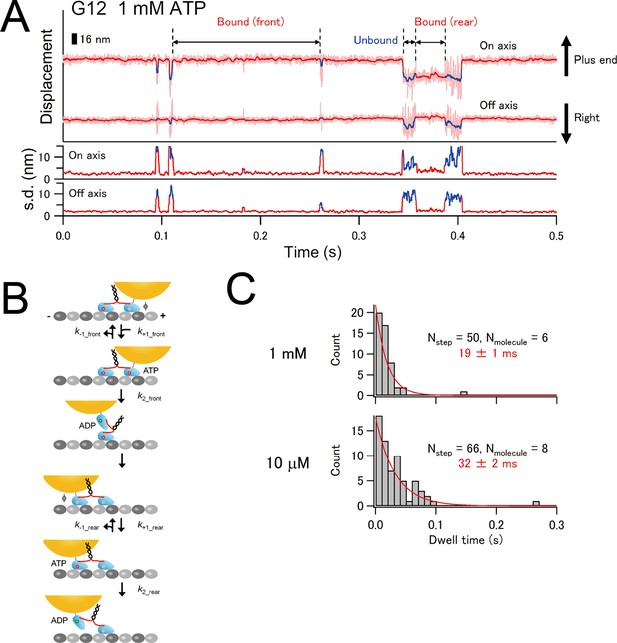
Binding to the rear-tubulin-binding site observed for the G12 E236–WT heterodimer.
(A) Typical trace for the centroid positions of the gold probe attached to the WT head of the E236A–WT heterodimer with a 12 poly-Gly insertion in the presence of 1 mM ATP. The WT head of the G12 heterodimer occasionally takes a backward step (i.e., binding to the rear-binding site 16 nm toward the minus-end of the microtubule; indicated as ‘bound (rear)’). The frequency of this backward step was 14% (N = 50 among 317 unbinding events) under the 1 mM ATP condition. (B) The schematic showing the observed backward step for the G12 heterodimer; the gold-labeled leading WT head detaches and binds to the rear-tubulin binding site. The dwell time of the bound state in the rear-binding site includes the ATP-binding rate k+1_rear and the ATP-promoted detachment rate k2_rear. (C) Histograms of the dwell time of the bound state in the rear-binding site in the presence of 1 mM and 10 µM ATP. The dwell time under the 10 µM ATP condition was greater than that under the 1 mM ATP condition, suggesting that the bound state involves ATP-binding and -hydrolysis of the gold-labeled head.
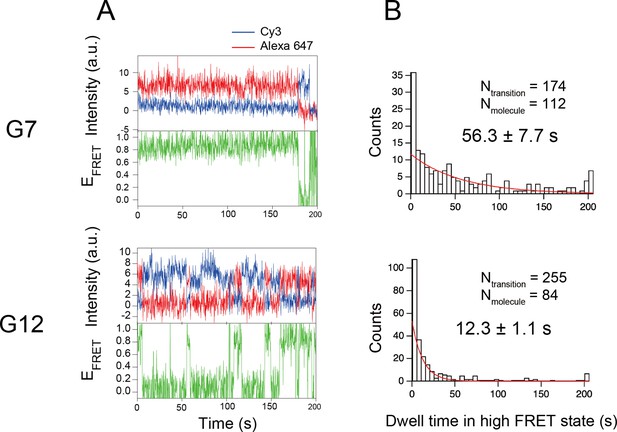
Single-molecule FRET between donor-labeled E236A head of E236A–WT heterodimer with extended neck linker and acceptor-conjugated ATP.
(A) Typical traces of fluorescence intensities of donor (Cy3, blue) and acceptor (Alexa 647, red) fluorophores, calculated FRET efficiency (EFRET), for E236A–WT heterodimer with Cy3 dye on the E236A head in the presence of 200 nM Alexa 647-conjugated ATP recorded at 5 fps. G7 and G12 represents E236A–WT heterodimer with 7 or 12 poly-Gly insertion between the neck linker and the neck coiled-coil. (B) Histogram of the dwell time of high FRET state. The solid lines show fit with exponential decay and the mean dwell time (± SEM) is shown within the histogram. The inverse of the mean dwell time for G7 and G12 E236A–WT heterodimers are 0.018 ± 0.002 and 0.081 ± 0.006 s–1, respectively (Figure 5D).
-
Figure 6—figure supplement 5—source data 1
Excel file containing fluorescent intensity time traces of donor and acceptor for Figure 6—figure supplement 5A.
- https://cdn.elifesciences.org/articles/106228/elife-106228-fig6-figsupp5-data1-v1.xlsx
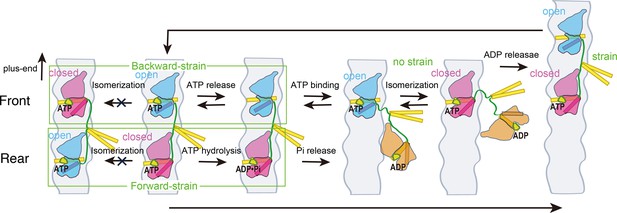
Model to explain how the tension exerted by the stretched neck linker in dimeric kinesin coordinates the microtubule detachment of the two heads.
This model is based on the proposal that the transition between ATP-bound open and closed conformations of the head is regulated by the neck-linker strain (Figure 7—figure supplement 2). The open and closed conformational states of the head are indicated in blue and red, respectively, with the α6 helix, which connects to the neck linker, highlighted as a rod. The microtubule-detached ADP-bound state is shown in orange. The neck linker is depicted in green, while the α4 helix, which directly interacts with the microtubule, and the neck coiled-coil are shown in yellow. In the two-head-bound state, the front head remains in the open state because the backward strain prevents it from transitioning to the closed state. In this state, ATP can weakly bind to the nucleotide pocket but often dissociates. Conversely, the rear head is stabilized in the closed state because the transition to the open state is suppressed by the forward strain. ATP is tightly bound to the closed nucleotide pocket, causing the rear head to hydrolyze ATP and detach from the microtubule before the front head does. In the one-head-bound state, the microtubule-bound head can transition between the open and closed states. However, once the tethered head binds preferentially to the forward tubulin-binding site, the strain built up between the two microtubule-bound heads stabilizes the rear head in the closed conformation and the front head in the open conformation.
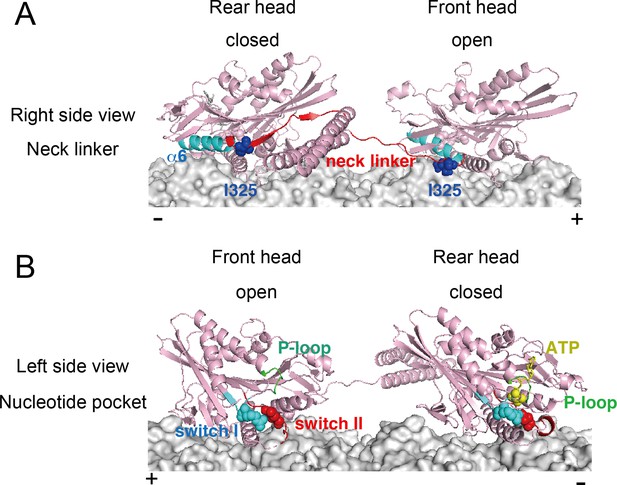
Structural difference between the open and closed conformational states of the kinesin head.
The structural model of dimeric kinesin is based on PDB# 4HNA (rear head in closed conformation; Gigant et al., 2013) and 4LNU (front head in open conformation; Cao et al., 2014). (A) The neck linker and α6 helix, which connects the neck linker, are shown in red and cyan, respectively. The I325 residue is depicted in blue spheres. In the open state, I325 residue is prohibited from binding to the complementary hydrophobic pocket due to the backward strain applied to the neck linker. In contrast, in the closed rear head, the hydrophobic interaction is stabilized by the forward strain. (B) ATP, P-loop, switch I, and II loops in the nucleotide pocket region are shown in yellow, green, cyan, and red, respectively. R203 residue in switch I and E236 residue in switch II, both essential for the hydrolysis reaction (Parke et al., 2010), and γPi of ATP are shown in space-filling. In the open head, switch I and II loops are distanced from the P-loop, whereas in the closed state, these loops approach the γPi of ATP and become capable of catalyzing the ATP hydrolysis reaction.
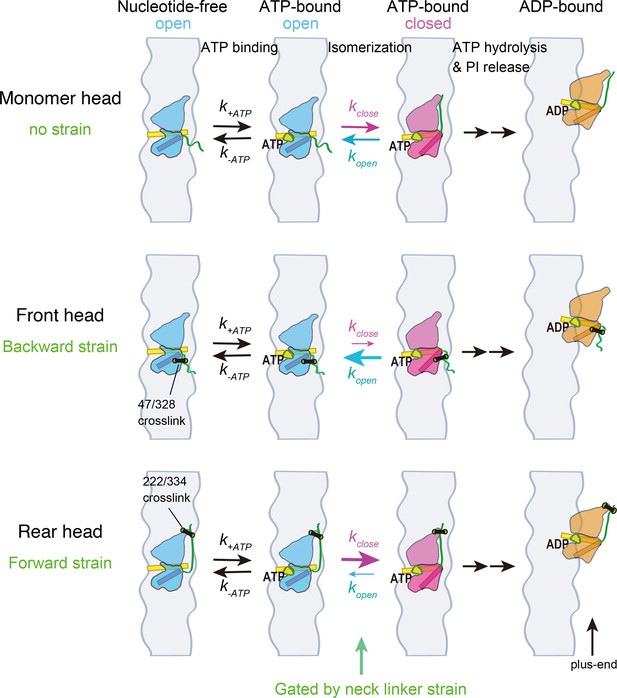
Schematic model showing that the open-closed conformational transition (isomerization) is gated by the neck-linker strain.
The color coding is the same as in Figure 7. Monomer head without the neck-linker strain can transit between two distinct ATP-bound configurations (Figure 2C; kclose ~ kopen): the neck-linker undocked, nucleotide-pocket opened (open; bule) state, and the neck-linker docked, nucleotide-pocket closed (closed; red) state. Backward strain applied to the front head (or C47/C328 crosslink) shifts the equilibrium toward the open state (kclose < kopen), suppressing ATP hydrolysis and promoting reversible ATP dissociation. On the other hand, forward strain applied to the rear head (or C222/C334 crosslink) shifts the equilibrium toward the closed state (kclose > kopen), increasing ATP affinity and facilitating ATP hydrolysis. We speculate that the rear head initially adopts an open conformation after ATP binding and the ATP-binding kinetics to the open head (k+ATP and k-ATP) is the same as the monomer without neck-linker constraint. Assuming the transition rate to the closed state significantly exceeds the ATP dissociation rate in the rear head (k−ATP < kclose), the apparent ATP release rates (k−1) observed for the E236A mutants (Figures 2D and 5B) are dependent on the rate constants for the transition from closed to open state (kopen). As a result, k−1 of E236A mutants become lower as the forward strain increases (which decreases the kopen) (Figure 5D).
Tables
Kinetic rate constants for disulfide-crosslinking monomers.
Steady-state ATP-turnover rate (kcat and Km(MT)), pre-steady-state mant-ATP binding (k+1 and k−1), and microtubule dissociation rates (k2 and k2_SMF, measured by light scattering and single-molecule fluorescence) measured under reduced and oxidized conditions.
WT | C222/C334 | C47/C328 | ||||
---|---|---|---|---|---|---|
Reduced | Oxidized | Reduced | Oxidized | Reduced | Oxidized | |
kcat (s−1) | 57.1 ± 4.4 | 58.5 ± 3.4 | 27.0 ± 2.3 | 2.2 ± 0.5 | 32.2 ± 1.3 | 1.5 ± 0.2 |
Km(MT) (µM) | 0.31 ± 0.08 | 0.36 ± 0.08 | 0.23 ± 0.06 | 0.200 ± 0.007 | 0.28 ± 0.06 | 0.13 ± 0.04 |
k+1 (s−1 µM−1) | 5.5 ± 0.1 | 6.0 ± 0.4 | 5.1 ± 0.7 | 1.62 ± 0.07 | 4.0 ± 0.2 | 2.0 ± 0.1 |
k−1 (s−1) | 136 ± 5 | 125 ± 12 | 117 ± 23 | 3.7 ± 1.9 | 96 ± 6 | 42 ± 4 |
k2 (s−1) | 52.1 ± 0.6 | 59.1 ± 0.9 | 69.3 ± 0.5 | N.D. | 51.5 ± 0.7 | 7.0 ± 0.3 |
k2_SMF (s−1) | 44.0 ± 1.9 | 41.6 ± 1.2 | 47.2 ± 1.2 | 57.1 ± 1.8 | 42.1 ± 0.4 | 2.30 ± 0.08 |
C4/C330 | C47/C335 | |||||
Reduced | Oxidized | Reduced | Oxidized | |||
kcat (s−1) | 33.5 ± 2.1 | 32.6 ± 1.2 | 43.1 ± 3.0 | 11.9 ± 0.6 | ||
Km(MT) (µM) | 0.42 ± 0.08 | 8.36 ± 0.98 | 0.33 ± 0.05 | 0.10 ± 0.01 | ||
k+1 (s−1 µM−1) | 4.7 ± 0.5 | 4.1 ± 0.3 | 9.1 ± 0.3 | 4.5 ± 0.1 | ||
k−1 (s−1) | 148 ± 18 | 96 ± 9 | 85 ± 10 | 41 ± 5 | ||
k2 (s−1) | 68.6 ± 0.5 | 80.8 ± 0.8 | 41.6 ± 0.6 | 19.4 ± 0.2 | ||
k2_SMF (s−1) | 59.5 ± 2.3 | 58.4 ± 2.6 | 52.1 ± 2.0 | 22.5 ± 0.3 |