Spatial tuning and brain state account for dorsal hippocampal CA1 activity in a non-spatial learning task
Figures
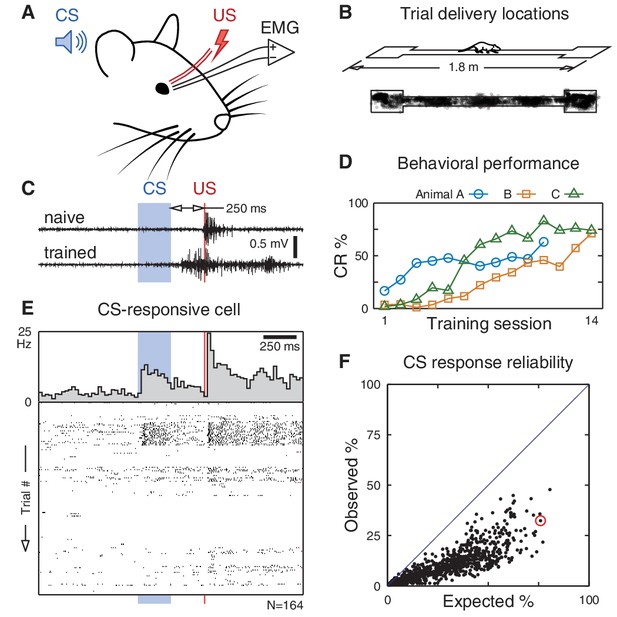
CA1 pyramidal responses during trace eyeblink conditioning in a freely moving rat.
(A) An eyeblink trial is a sequence of a tone (CS), a stimulus-free period (trace interval), and a blink-inducing electrical pulse (US). Blinks are measured on an eyelid electromyogram (EMG). (B) Trials are delivered randomly throughout the environment as the rat traverses a linear track for water reward. (C) Eyelid EMG from early and late in learning. A conditioned response (CR) is defined as an increase in EMG power anticipating the US onset. (D) Learning is apparent as an increase in CR frequency over the course of training. (E) Example pyramidal cell that significantly increased its firing rate following the CS onset. If spike counts on each trial were Poisson distributed according to this cell’s average response (top), we would expect to observe this CS-evoked increase in 76% of trials. Instead, its spike rasters (bottom) show that the response is much less reliable and occurs in only 32% of trials. (F) Observed vs. expected CS response reliability. All 1264 recorded pyramidal cells (back dots) responded in less than half of trials. The example from (E) is circled in red.
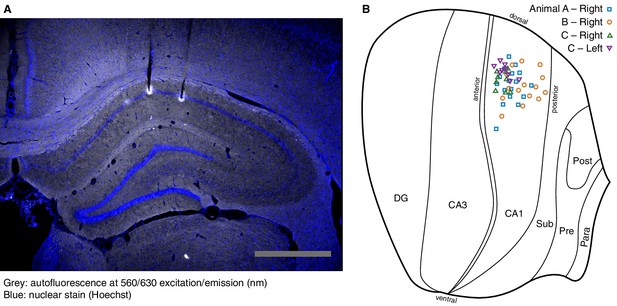
Location of recording sites.
(A) Example coronal section used to reconstruct recording sites. The electrolytic lesions used to mark the final tetrode locations can be detected as autofluorescence. This is superimposed with a nuclear stain to identify the CA1 cell layer. Scale bar: 1 mm. (B) Recording sites were registered onto a brain atlas (Paxinos and Watson, 2007), then projected onto a flatmap of the unfolded hippocampal formation (Petrovich et al., 2001). This shows only those sites from which we recorded at least one pyramidal cell.
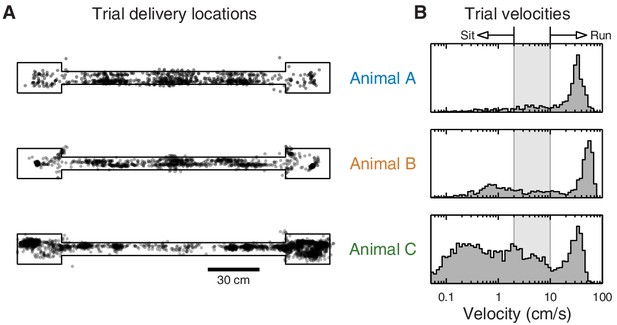
Distribution of animal location and velocity during eyeblink trials.
(A) Animal locations where eyeblink trials were delivered. All animals received eyeblink conditioning trials at random locations on the track. Animal C also received trials at random time intervals, producing a denser sampling of the endboxes. (B) Histogram of animal velocity when eyeblink trials were delivered. The indicated thresholds were used to classify individual trials as 'sit' vs. 'run' in subsequent analyses.
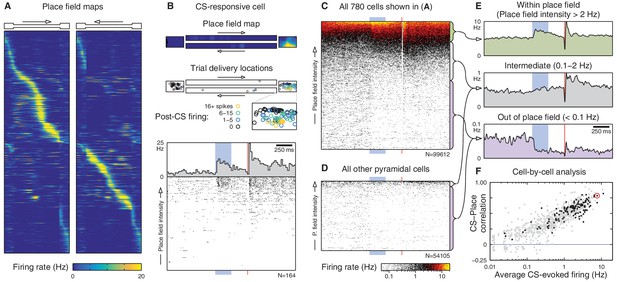
Spatial tuning modulates CA1 responses to eyeblink stimuli.
(A) Spatial firing rate maps (place fields) for the 780 cells active on the linear track. Each row is a single cell; left and right panels show different directions of traversal. These maps are computed excluding eyeblink trials. (B) Place fields predict CS responses for the example cell from Figure 1E. Top: Place field map. Boxes are the track endboxes, and the track itself is duplicated to show different directions of traversal. Middle: Animal location during eyeblink trials, color-coded by CS-evoked firing. Bottom: Spike rasters ordered by place field intensity at trial delivery locations. (C) Spike rasters for all cells in (A), ordered by place field intensity. For visualization, many rasters are compressed onto a single row, and overlapping spikes are given warmer colors. Electrical artifacts prevent spike detection during the 10 ms US delivery window. (D) Spike rasters for the remaining 484 cells not active on the linear track. (E) Average firing rate over all cells and trials, grouped by place field intensity. (F) Cell-by-cell analysis of the correlation (Spearman’s ρ) between CS-evoked firing rate and place field intensity. Most cells have a large positive correlation, especially those that appeared significantly CS-responsive (black dots). The only cells without a positive correlation are those that fired very few spikes. The cell from (B) is circled in red.
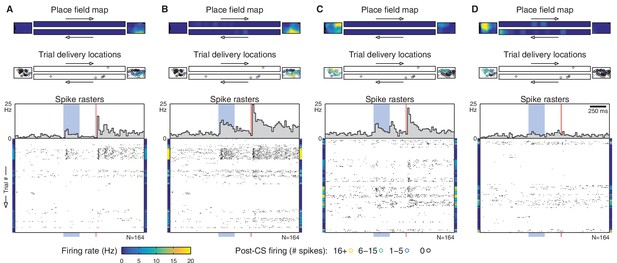
Additional simultaneously-recorded cells.
The example cell from Figures 1E and 2B is shown in panel (B). The other panels of this figure show simultaneously recorded cells from different tetrodes. As in Figure 2B, each panel contains a place field map, trial delivery locations (color-coded by CS-evoked firing), and spike rasters for a single cell. Rasters are shown in chronological order (as in Figure 1E), so the same row in each panel corresponds to the same trial. Note that different sets of cells fire as the animal moves around, and cells with disjoint place fields fire on different subsets of trials. The colored bars flanking each raster show the cell’s place field intensity for each trial.
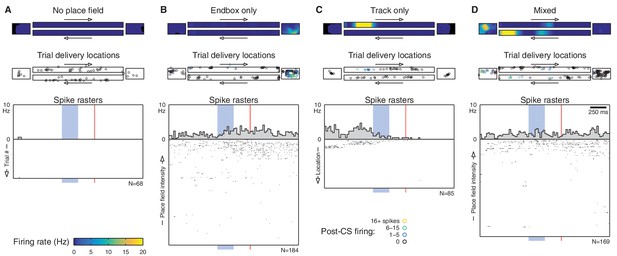
Additional single-cell examples.
This figure shows additional single-cell examples, presented in the same fashion as Figure 2B. (A) 484 cells (38%) did not have a place field in the environment and fired very few spikes during eyeblink trials. (B) 301 cells (24%) had a place field in the endbox only. However, this count is very sensitive to the threshold on the place field’s peak firing rate. For example, increasing this rate threshold from 2 Hz to 5 Hz would reclassify 160 of these 'endbox only' cells as 'no place field'. A small fraction of 'endbox only' cells (14 cells total) had place fields in both endboxes. (C) 325 cells (26%) had a place field on the track proper. Most of these (188 cells) had a single uni-directional place field. Note that non-uniform sampling of the track can cause place field traversals to appear as a CS-evoked change in firing. In this example, the animal received eight eyeblink trials as it was exiting this cell’s place field, but only one trial as it was entering. As a result, we observe a significant decrease in firing following the CS onset (p=0.006, Wilcoxon signed-rank test). (D) 154 cells (12%) had place fields on the track and in the endbox(es). Many of these were due to a single place field that extended onto both areas, but some (like this example) had multiple distinct place fields.
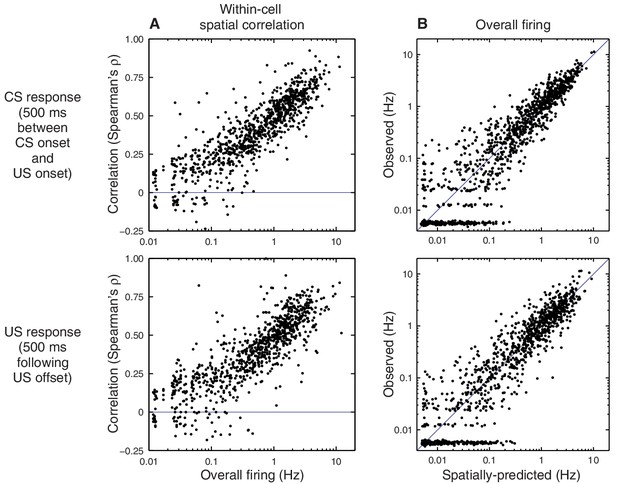
Spatial tuning predicts CS- and US-evoked responses on a single-cell basis.
This figure shows additional cell-by-cell analyses. Each dot represents a single recorded cell. The top row analyzes the CS response (firing in the 500 ms window between the CS onset and the US onset), and the bottom row analyzes the US response (firing in the 500 ms following the US offset). (A) Within-cell correlation (Spearman’s ρ), across trials, between spatial tuning and stimulus-evoked firing. A correlation of 1 indicates that a cell exhibits a strictly monotonic relationship between place field intensity and observed firing. Note that all cells exhibit a positive correlation except those that fired very few spikes overall; such cells have many trials with zero observed spikes, and these ties reduce the measured correlation. The top plot is a duplicate of Figure 2F. (B) Predicted vs. observed stimulus-evoked firing rate for each cell (averaged over trials). Note that all cells lie close to the diagonal, and there are no outliers to suggest a subpopulation of cells that deviates from the spatially-predicted rates. Values less than 0.01 are not shown to scale.
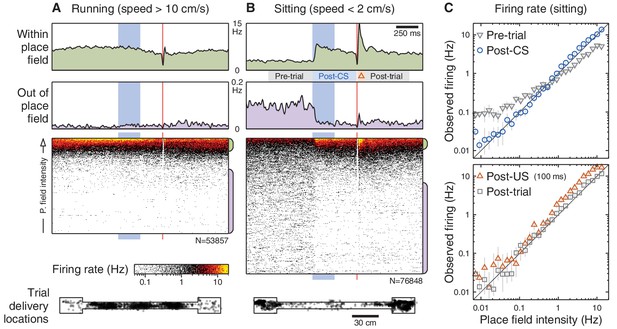
Brain state modulates CA1 responses to eyeblink stimuli.
(A) Responses on trials delivered while the animal was running (40% of all trials). Top: Average firing rate among trials presented within (green) and out of (purple) the place field (same thresholds as Figure 2E). Middle: Spike rasters ordered by place field intensity. Bottom: Distribution of animal locations for this set of trials. (B) Responses on trials delivered while the animal was sitting (46% of all trials). (C) Top: Firing rates, on sitting trials, immediately before (grey) and after (blue) the CS onset. Note that the post-CS firing matches the place field intensity more closely than the pre-CS firing does. Each marker shows the mean and bootstrapped 95% confidence interval for a small range of intensities; values less than 0.01 Hz are not shown to scale. Bottom: Same analysis showing the predicted vs. observed firing rates immediately after the US (red) and shortly after that (grey). The time windows for analysis are indicated in panel (B).
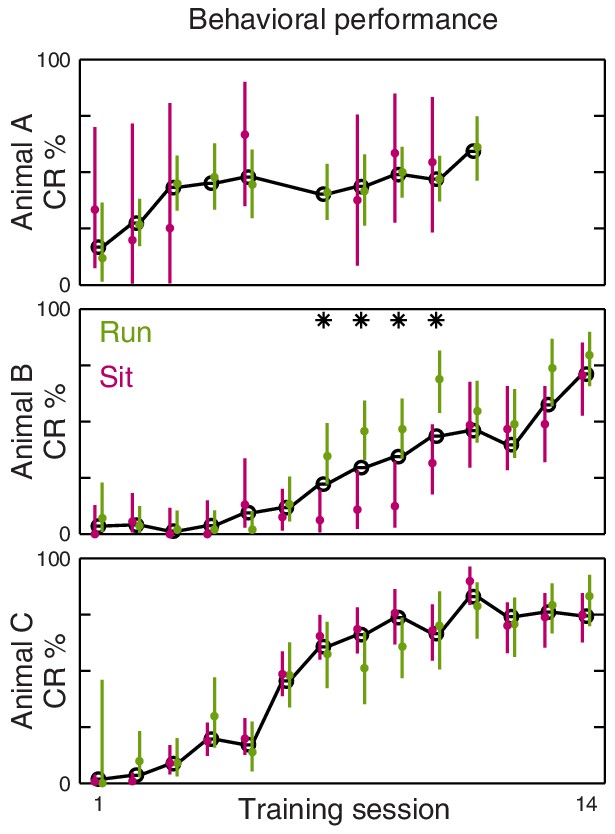
Eyeblink performance is not impaired in running trials.
Plots show behavioral performance over the course of training for the three animals. The mean CR rate over all trials is shown in black. Mean with 95% Bernoulli confidence intervals for running/sitting trials are shown in green/magenta, respectively. Asterisks indicate sessions where either confidence interval does not include the overall mean.
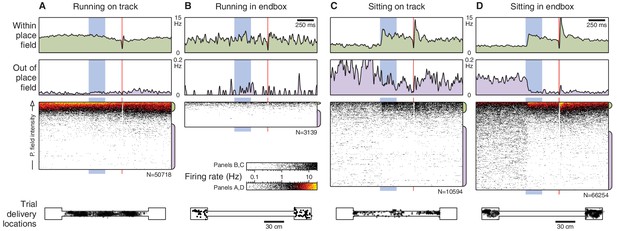
State-dependent responses, separated by location.
In Figure 3, most running trials occur on the track, whereas the sitting trials occur mostly in the endboxes. To check whether it is behavior (run vs. sit) or location (track vs. endbox) that is the relevant feature, we repeated this analysis for each of the four conditions. Panels (B) and (C) contain fewer trials than (A) or (D), so their spike rasters are shown at a different scale.
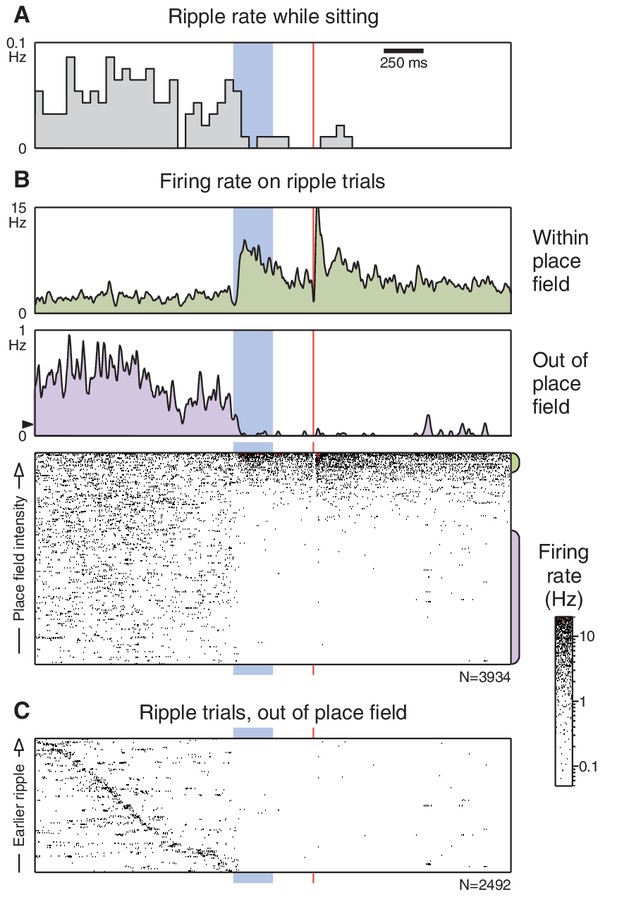
Ripples cease following the CS onset.
(A) Ripple occurrence rate during trials presented while the animal was sitting (ripples did not occur when the animal was running). (B) Unit responses on trials where a ripple was detected prior to the CS onset. Layout is similar to Figure 3B, but note the difference in scale for the 'out of place field' peri-event firing rate. The black triangle marks the average pre-trial firing rate from the corresponding panel in Figure 3B. (C) 'Out of place field' rasters sorted by the time that the ripple occurs. Some trials had more than one ripple; for these the longest ripple was used.
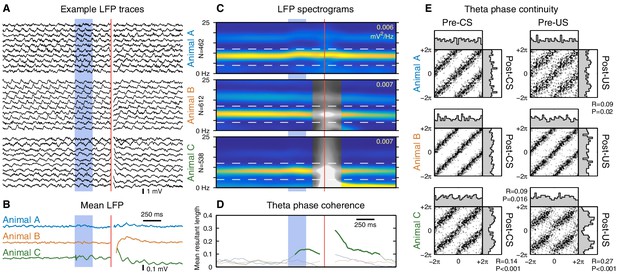
The CS does not reset ongoing hippocampal theta oscillations.
This figure shows CS- and US-evoked changes to ongoing theta oscillations in the hippocampal local field potential (LFP) while running. (A) Randomly selected example LFP traces from each animal. Note that theta oscillations are prominent and consistent across trials. (B) Averaged hippocampal LFP. Theta-band oscillations are visible in animal C, but note the 10-fold reduction in scale compared to the raw LFPs. (C) LFP spectrograms. Note the strong theta power that persists throughout the trial. Horizontal dashed lines indicate the 4–12 Hz theta band, and the peak spectral power density is indicated in the top right of each spectrogram. Animals B and C exhibited high-amplitude transients following the US, and time windows that overlap this transient are shown in greyscale. (D) Mean resultant length, a measure of theta phase coherence across trials. Values that correspond to a significantly non-uniform phase distribution (p<0.01, Rayleigh test) are shown in bold. Animal C exhibits a sustained phase alignment that persists for several theta cycles, but the other two animals show no significant phase alignment at all. (E) Phase transition curves. Each scatterplot compares the theta phase before and after the stimulus (see Materials and methods and Figure 5—figure supplement 3); each dot is a single trial and two cycles are shown for clarity. A diagonal line (e.g. animal B) indicates that the theta rhythm is unaffected by the eyeblink stimuli, whereas a horizontal line would indicate theta reset to a fixed phase. Each scatterplot is accompanied by histograms of the phase distributions. The mean resultant length (R) and Rayleigh test P-value are reported for cases where P<0.05. Note that even in cases with a significant stimulus-evoked alignment of theta phase, the actual perturbation of ongoing theta oscillations is very subtle.
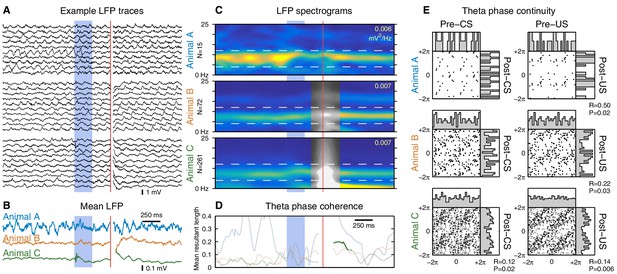
Stimulus-evoked changes in hippocampal theta oscillations while sitting.
Same analysis as Figure 5, but for trials delivered during periods of relatively high theta power while sitting. Overall, the results are similar to, but noisier than, the running case. Inclusion criteria are: (1) the animal was sitting, (2) the ratio of pre-trial theta power (4–12 Hz) to delta power (1–4 Hz) was > 4, and (3) the pre-trial theta power was greater than the 5th percentile of theta power observed during running. (A) Despite the stringent inclusion criteria, these randomly-selected examples reveal a more diverse set of LFP activity than observed during running. (B) Mean LFPs. Animal A received very few trials that met the inclusion criteria, producing a very noisy mean LFP. (C) LFP spectrograms are shown using the same color scale as in Figure 5C. Note the reduced theta power compared to the running case. (D) No animals exhibited a significant alignment of theta phase to the CS, but this analysis is potentially confounded by smaller sample sizes. (E) Phase transition curves show much greater dispersion compared to the running case, which may reflect: (1) reduced accuracy of phase extraction due to reduced theta power, (2) unintentional inclusion of non-theta trials, or (3) increased variability in theta frequency.
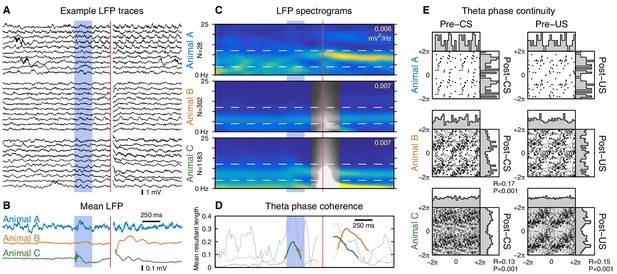
Stimulus-evoked initiation of hippocampal theta oscillations.
Same analysis as Figure 5, but for trials delivered during periods of non-theta (i.e. the remaining sitting trials not shown in Figure 5—figure supplement 1). (A) These randomly-selected LFP traces show several clear examples of theta initiation (a change from non-theta to theta), but also demonstrate that it does not always occur. (B) Mean LFPs. Note that the mean CS response for animal C is dominated by a transient rather than the persistent theta oscillations seen in Figure 5B. (C) LFP spectrograms show large changes in the spectral power following the onset of the eyeblink stimuli. (D) Animal C again shows significant alignment of theta phase following the CS, but the timing and duration of this alignment suggests that it is due to the broadband transient rather than a genuine alignment of the theta rhythm. (E) The theta phase continuity plots are very noisy because the pre-CS theta phase is not well-defined and the presence of post-CS theta oscillations is highly variable.
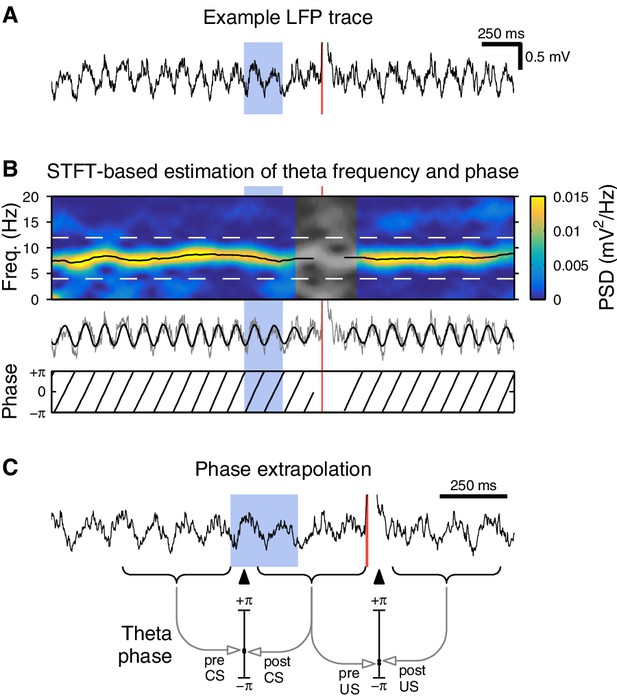
Methods for theta phase analysis.
(A) Example hippocampal local field potential (LFP) from a single eyeblink trial. A high-amplitude transient can be seen immediately following the US delivery. (B) Instantaneous theta frequency and phase were estimated using a short-time Fourier transform (STFT). Top: At each time point, we determine the theta frequency by finding the frequency with the highest spectral power (black line). The theta amplitude and phase are then extracted from the STFT at that frequency. Middle: Raw trace from (A) with extracted theta overlaid in black. Bottom: Corresponding theta phase. This is used for the theta coherence analysis in Figure 5D. (C) Phase extrapolation for the phase continuity analysis in Figure 5E. We use non-overlapping STFT windows (indicated by curly braces below the LFP trace) to ensure that the pre- and post-stimulus phase are estimated independently of each other. To assist in comparison, we use the corresponding frequency estimates to extrapolate both phase estimates to the same point in time (indicated by black triangles). This procedure is repeated for the US analysis.
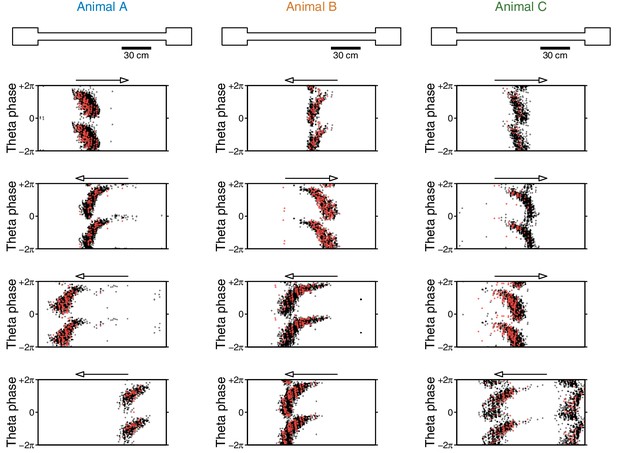
Phase precession is unaffected by the eyeblink stimuli.
Phase precession plots for 12 example cells (four per animal). Each dot shows the animal’s location (x-axis; arrow indicates direction of traversal) and theta phase (y-axis; two cycles shown) for a single spike. As the animal runs through the cell’s place field, the cell fires at an earlier phase of theta; this phenomenon is known as phase precession. Black dots are spikes that are not associated with eyeblink trials, and red crosses are spikes fired within 500 ms of the CS or US. Note that the CS- and US-evoked spikes fall within the distribution of non-eyeblink spikes. These example cells were chosen because they had many eyeblink trials in their place field(s), providing favorable conditions for observing any deviations from their phase precession curves. Theta phase is extracted from the same tetrode that the single unit was recorded on.
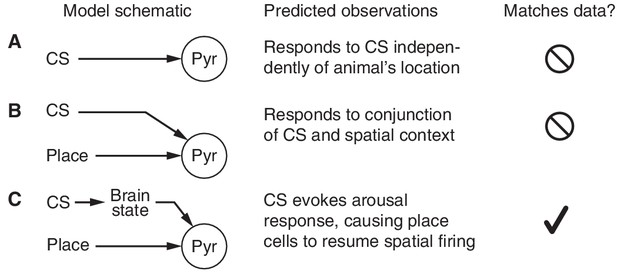
Models of CS-responsiveness.
(A) CS cells respond to the CS independently of place. None of the recorded cells consistently responded in space-invariant manner. (B) CS-place cells encode a context-specific representation of the non-spatial stimulus. The lack of CS responses when the animal is running is not consistent with this model. (C) Place cells encode purely spatial information, but arousal-mediated activation of place cells can produce changes in firing following a non-spatial stimulus.