Dynamic control of Hsf1 during heat shock by a chaperone switch and phosphorylation
Figures
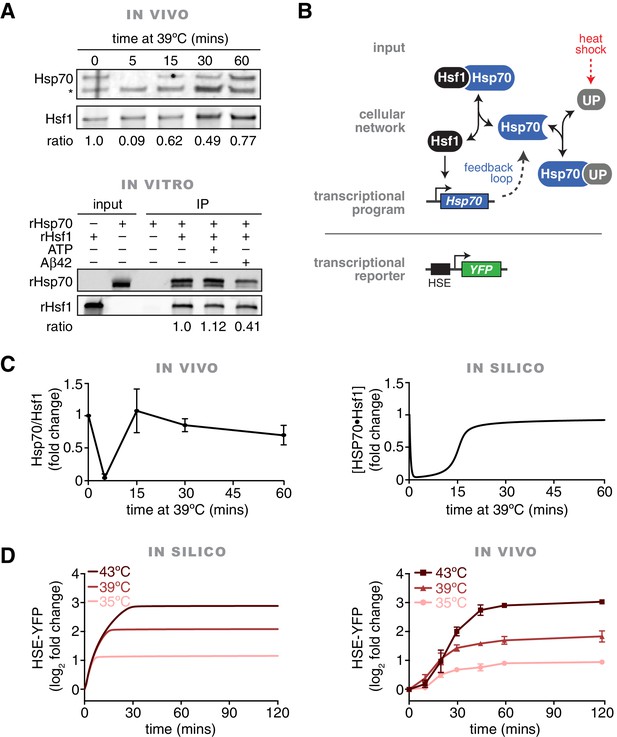
In vivo, in vitro and in silico evidence for an Hsp70•Hsf1 dissociation switch as the core mechanism regulating the heat shock response.
(A) IP/Western blot showing Hsp70 transiently dissociating from Hsf1 during heat shock (upper panel). Western blots were probed with antisera recognizing Ssa1/2 (top) and an anti-FLAG antibody to recognize Hsf1 (bottom). IP of recombinant proteins were performed with rHsf1-3xFLAG as bait and analyzed by Western blot (lower panel). Blots were probed with an anti-HIS antibody to recognize recombinant Ssa2 (rSsa2, top) and with an anti-FLAG antibody to recognize recombinant Hsf1 (rHsf1, bottom). The IPs were also performed in the presence of 1 mM ATP or five-fold molar excess of Aβ42 peptide. The numbers below the blots indicate the normalized ratio of Ssa2/Hsf1. (B) Cartoon schematic of the mathematical model of Hsf1 regulation illustrating the network connections and the feedback loop. UP is an abbreviation for 'unfolded proteins'. See Figure 1—figure supplement 2 and Materials and methods for details, equations and parameters. (C) Quantification of the top three peptides derived from Hsp70 proteins Ssa1 or Ssa2 (Ssa1/2 are grouped due to 98% identity) relative to the top three peptides from Hsf1 as determined by IP/MS (left panel). The IP experiments were performed in triplicate at the indicated time points following a shift to 39°C. See Figure 1—figure supplement 1. The values are the average of the three replicates and error bars depict the standard deviation. Source data are included as Figure 1—source data 1. Simulation of the levels of the Hsf1•Hsp70 complex over time following a shift from 25°C to 39°C (right panel). (D) Simulation of the levels of the HSE-YFP reporter over time following upshift from 25°C to the indicated temperatures (left panel). Flow cytometry measurements of cells expressing the HSE-YFP reporter following upshift from 25°C to the indicated temperatures (right panel). See Materials and methods for assay and analysis details.
-
Figure 1—source data 1
Table of peptide counts from proteins identified in Hsf1-3xFLAG-V5 IP/MS experiments.
- https://doi.org/10.7554/eLife.18638.004
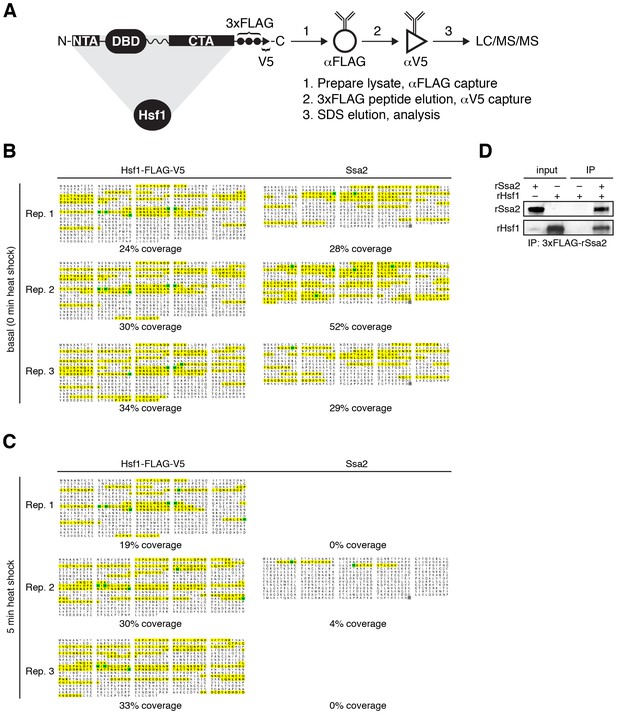
Ssa2 co-precipitates with Hsf1 in basal conditions but is greatly reduced immediately following heat shock.
(A) Schematic of the Hsf1-3xFLAG-V5 construct and the two-step serial affinity purification strategy. (B) Sequence coverage of Hsf1 and Ssa2 as determined by mass spectrometry in basal conditions at 25°C. Three IP replicates were performed in each condition. The sequences of identified peptides are highlighted in yellow. (C) Sequence coverage of Hsf1 and Ssa2 as determined by mass spectrometry following five minutes at 39°C. Three IP replicates were performed in each condition. The sequences of identified peptides are highlighted in yellow. (D) IP of recombinant proteins were performed with FLAG-rSsa2 as bait and analyzed by Western blot. Blots were probed with an anti-FLAG antibody to recognize recombinant Ssa2 (rSsa2, top) and with an anti-HIS antibody to recognize recombinant Hsf1 (rHsf1, bottom).
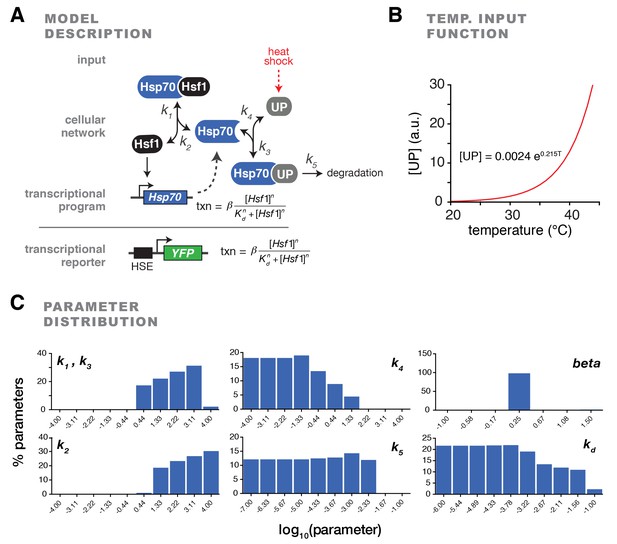
Description and parameterization of mathematical model of the heat shock response.
(A) Model schematic depicting the kinetic parameters and transcriptional Hill function. (B) The function used to relate the concentration of unfolded proteins (UP) to temperature to stimulate the heat shock response. (C) The model was simulated with a wide range of values for each kinetic parameter. ‘Successful’ parameter sets fulfilled specific criteria observed in the experimental data (see Materials and methods). The percentages of successful parameter sets that include the indicated values of each parameter are plotted.
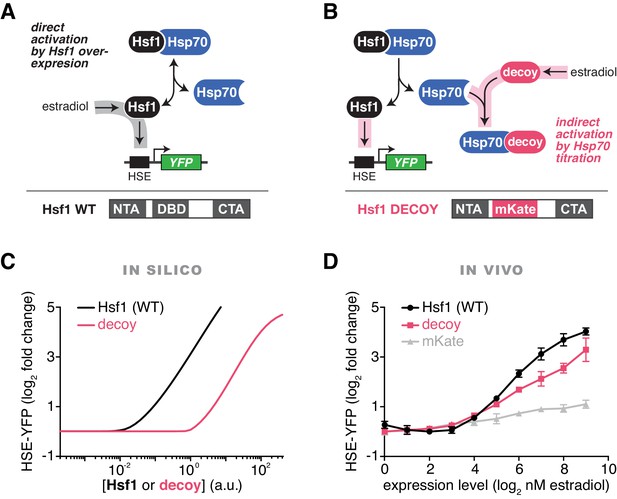
Prediction and validation of synthetic perturbations to the Hsf1-Hsp70 feedback loop.
(A) Cartoon schematic of activation by overexpressing full length Hsf1. Hsf1 can be expressed at many different levels by titrating the concentration of estradiol in the media (See Figure 2—figure supplement 1 and Materials and methods). The Hsf1 domain architecture is displayed below. The DNA binding domain (DBD) is between N- and C-terminal activation domains (NTA and CTA). (B) Cartoon schematic of activation via overexpression of the Hsf1 decoy. The decoy domain architecture is displayed below. (C) Simulation of the HSE-YFP reporter as a function of the expression level of full length Hsf1 or the decoy. (D) Experimental measurement of the HSE-YFP reporter by flow cytometry in cells expressing full length Hsf1, the decoy or mKate alone across a dose response of estradiol. Cells were monitored following growth in the presence of the indicated concentrations of estradiol for 18 hr. Data points are the average of median YFP values for three biological replicates, and error bars are the standard deviation. See Materials and methods for assay and analysis details.
-
Figure 2—source data 1
Table of peptide counts from proteins identified in decoy IP/MS experiments with decoy-3xFLAG-V5 and Hsf1-3xFLAG-V5 as bait.
- https://doi.org/10.7554/eLife.18638.008
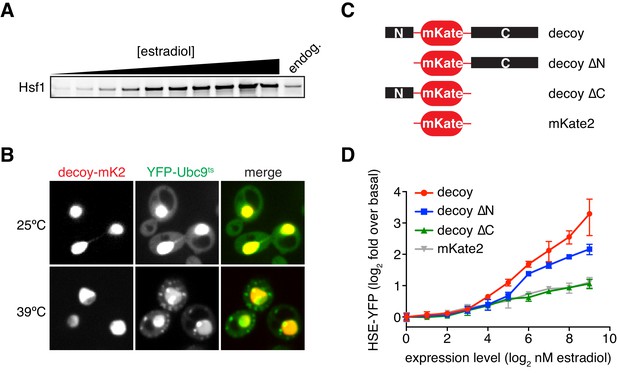
Overexpression of a decoy of Hsf1 activates endogenous Hsf1.
(A) Anti-FLAG western blot of cells expressing Hsf1-FLAG-V5 under the control of an estradiol-inducible promoter. Cells were incubated for four hours across a two-fold dilution series of estradiol (from 512 nM down to 1 nM) and the Hsf1 expression level was compared to expression from the endogenous promoter. (B) Cells expressing YFP-Ubc9ts were induced to express the mKate2-labeled decoy for four hours with 512 nM estradiol and were either left at 25°C or shifted to 39°C for 15 min and imaged by spinning disc confocal microscopy. YFP-Ubc9ts forms aggregates during heat shock, while the decoy remains apparently soluble. (C) Schematic of decoy constructs for domain analysis. (D) Measurement of the HSE-YFP reporter as a function of estradiol for the constructs depicted in (C).
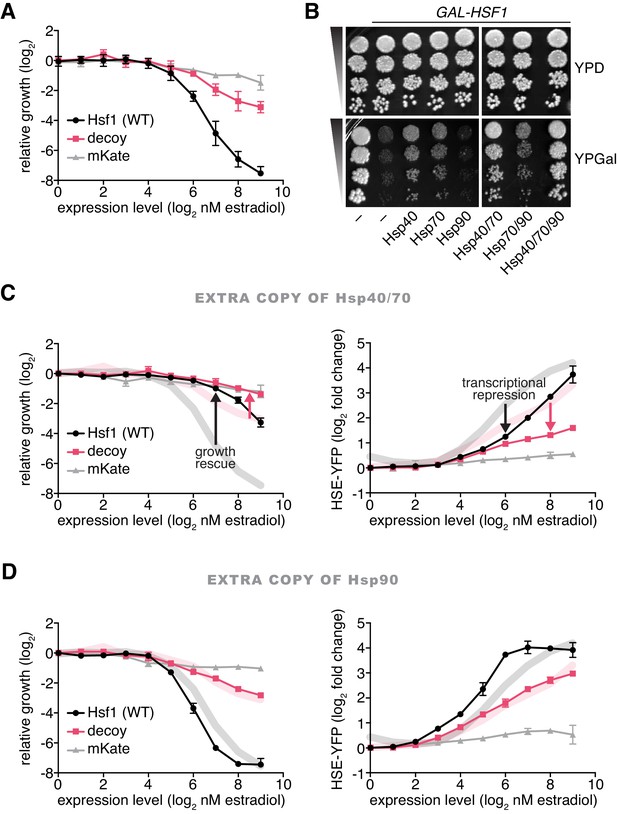
Hsp70 and Hsp40 suppress Hsf1 overexpression.
(A) Cells expressing full length Hsf1, the decoy or mKate alone were assayed for growth by flow cytometry following 18 hr of incubation with the indicated doses of estradiol. Data points are the average of normalized cell count values for three biological replicates, and error bars are the standard deviation. See Materials and methods for assay and analysis details. (B) Dilution series spot assays in the absence and presence of galactose to monitor growth of cells expressing full length Hsf1 from the GAL1 promoter. Ydj1 (Hsp40), Ssa2 (Hsp70), Hsc82 (Hsp90) and combinations thereof were expressed from strong Hsf1-independent promoters and assayed for their ability to rescue the growth defect caused by Hsf1 overexpression. (C) Cells expressing an extra copy of Hsp70 and Hsp40 were assayed for growth (left panel) and for induction of the HSE-YFP reporter (right panel) as a function of the expression level of full length Hsf1, the decoy or mKate by flow cytometry following 18 hr of incubation with the indicated doses of estradiol. The thick lines in the background are the reference curves for cells lacking extra chaperone expression (taken from Figures 3A and 2D). (D) Cells expressing an extra copy of Hsp90 were assayed for growth (left panel) and for induction of the HSE-YFP reporter (right panel). Reference curves are depicted as above.
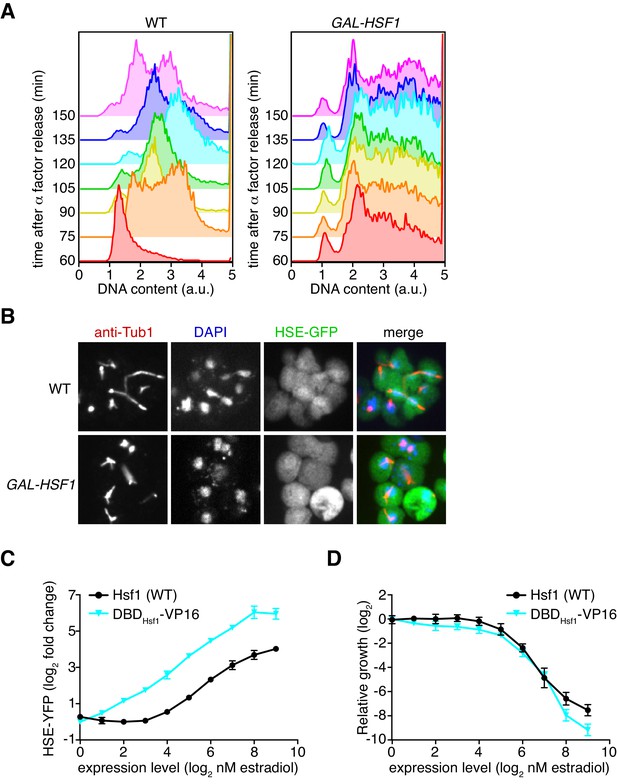
Hsf1 overexpression does not lead to a specific cell cycle arrest and cannot be explained by induction of a gratuitous transcriptional program.
(A) Flow cytometry analysis of DNA content of wild type cells and cells overexpressing Hsf1 from a galactose-inducible promoter following release from arrest with alpha factor for the indicated times. Wild type cells arrest in G1 and progress normally through the cell cycle. GAL-HSF1 cells fail to synchronize an show a broad distribution of DNA contents, indicating that no specific cell cycle stage is enriched. (B) Anti-tubulin immuno-fluorescence images of cycling wild type cells and cells GAL-HSF1 cells that had been grown in the presence of galactose for 12 hr. Wild type cells show G1, S and G2 phases, while GAL-HSF1 cells show non-standard tubulin staining. (C) Cells expressing wild type Hsf1 or a chimera of Hsf1’s DNA binding domain and the VP16 activation domain (DBDHsf1-VP16) were assayed for HSE-YFP induction by flow cytometry following 18 hr of incubation with the indicated doses of estradiol. Wild type Hsf1 data are from Figure 3D. (D) Relative growth of cells expressing wild type Hsf1 or DBDHsf1-VP16 across an estradiol dose response.
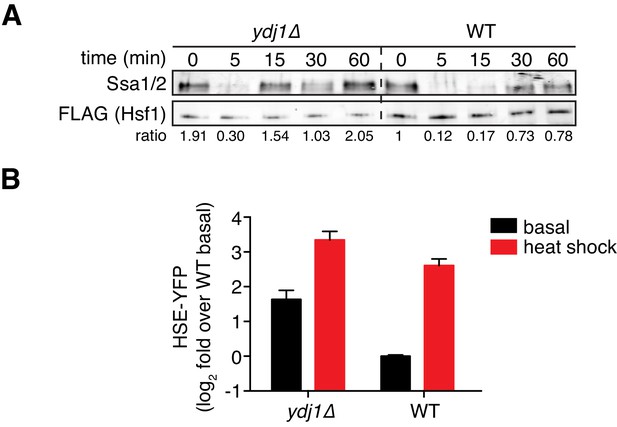
The Hsp40 Ydj1 is not required for the interaction between Hsf1 and Hsp70.
(A) IP/Western blot showing that Hsp70 binds to Hsf1 under basal conditions and transiently dissociates from Hsf1 during heat shock in both wild type and ydj1∆ cells. Western blots were probed with antisera recognizing Ssa1/2 (top) and an anti-FLAG antibody to recognize Hsf1 (bottom). The numbers below the blots indicate the ratio of Ssa1/2:Hsf1 normalized to the ratio in wild type cells under basal conditions. The relative level of Hsp70 binding to Hsf1 and the dissociation dynamics during heat shock are altered in the ydj1∆ cells, but this is difficult to interpret due to the increased basal Hsf1 activity and reduced fold change in activity during heat shock in these cells (see below). (B) Wild type and ydj1∆ cells expressing the HSE-YFP reporter were assayed for Hsf1 transcriptional activity in control and heat shock conditions by flow cytometry. Bars are the average of median YFP values for three biological replicates, and error bars are the standard deviation. Compared to wild type cells, ydj1∆ cells show increased basal Hsf1 activity and reduced fold change in activity during heat shock.
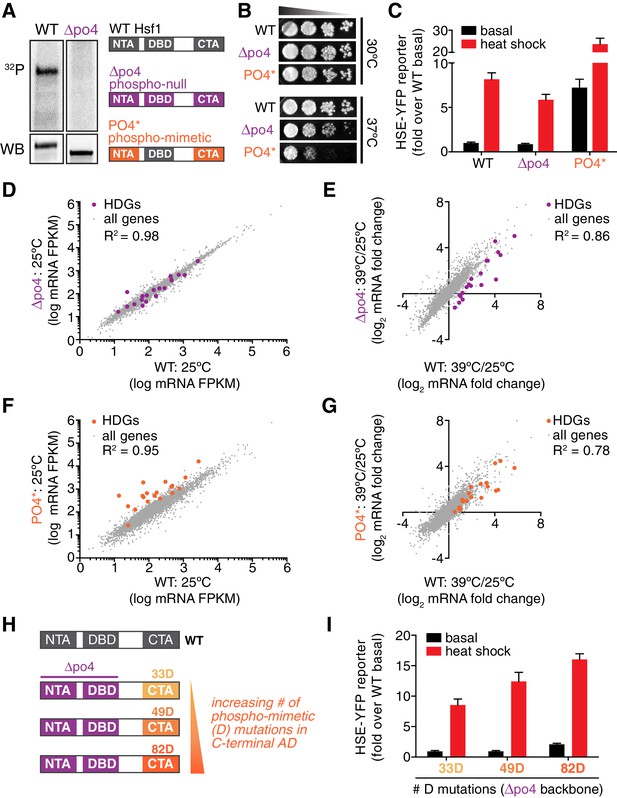
Phosphorylation is dispensable for Hsf1 function but tunes the gain of its transcriptional activity.
(A) 32P incorporated into wild type Hsf1-3xFLAG-V5 and Hsf1∆po4-3xFLAG-V5 during 30 min of heat shock (upper panel). Hsf1-3xFLAG-V5 and Hsf1∆po4-3xFLAG-V5 were affinity purified by anti-FLAG IP, resolved by SDS-PAGE and phosphor-imaged. Western blot of total lysate from wild type Hsf1-FLAG-V5 and Hsf1∆po4-FLAG-V5 cells was probed with an anti-FLAG antibody; Hsf1∆po4-FLAG migrates faster than wild type Hsf1-FLAG (lower panel). Schematics of the domain architecture and color code for wild type Hsf1, Hsf1∆po4 and Hsf1PO4*. (B) Wild type, Hsf1∆po4 and Hsf1PO4* cells were monitored for growth by dilution series spot assays. Cells were incubated at the indicated temperatures for two days. (C) Wild type, Hsf1∆po4 and Hsf1PO4* cells expressing the HSE-YFP reporter were assayed for Hsf1 transcriptional activity in control and heat shock conditions by flow cytometry. Bars are the average of median YFP values for three biological replicates, and error bars are the standard deviation. See Materials and methods for assay and analysis details. (D) Genome-wide mRNA levels were quantified in basal conditions in wild type and Hsf1∆po4 cells by RNA-seq. Within each sample, relative expression levels for each mRNA (gray dots) are plotted as fragments per kilobase per million mapped reads (FPKM). Hsf1-dependent genes (HDGs) are highlighted in purple. Source data are included as Figure 4—source data 2. (E) Fold changes of each mRNA in heat shock conditions compared to basal conditions were calculated for wild type and Hsf1∆po4 cells and plotted against each other. Hsf1-dependent genes (HDGs) are highlighted in purple. Source data are included as Figure 4—source data 2. (F) Genome-wide mRNA levels were quantified in basal conditions in wild type and Hsf1PO4* cells by RNA-seq (gray dots). Hsf1-dependent genes (HDGs) are highlighted in orange. Source data are included as Figure 4—source data 2. (G) Fold changes of each mRNA in heat shock conditions compared to basal conditions were calculated for wild type and Hsf1PO4* cells and plotted against each other. Hsf1-dependent genes (HDGs) are highlighted in orange. Source data are included as Figure 4—source data 2. (H) Schematic of mutants with different numbers of aspartate (D) residues. 33, 49 or 82 D residues were introduced in the CTA in the ∆po4 background. (I) Mutants depicted in (H) expressing the HSE-YFP reporter were assayed for Hsf1 transcriptional activity in control and heat shock conditions by flow cytometry as above.
-
Figure 4—source data 1
Table of Hsf1 phosphorylation sites identified in Hsf1-3xFLAG-V5 IP/MS IP/MS experiments in various conditions.
- https://doi.org/10.7554/eLife.18638.014
-
Figure 4—source data 2
Table of genome wide transcript levels as measured by RNA-seq under basal (30°C) and heat shock conditions (30 min at 39°C) in wild type, Hsf1∆po4 and Hsf1PO4* cells.
Values are FPKM.
- https://doi.org/10.7554/eLife.18638.015
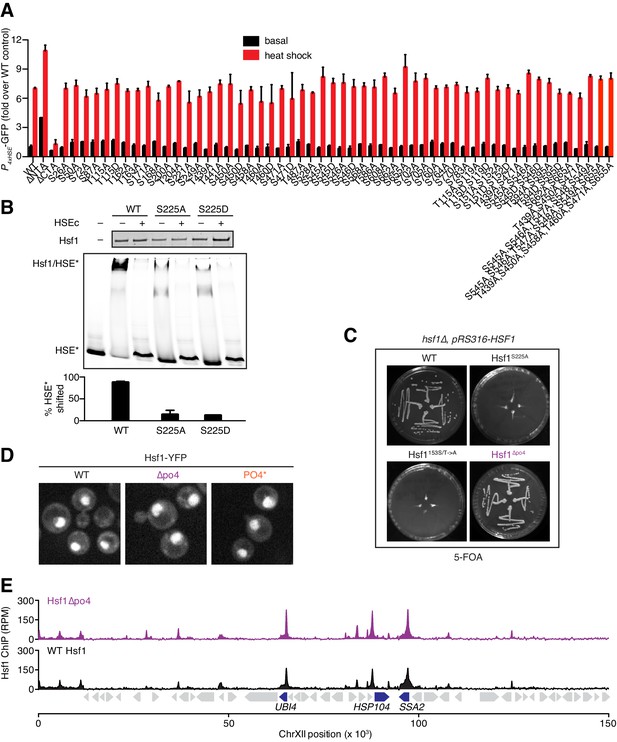
Mutational analysis of Hsf1 phosphorylation sites reveals a single essential serine and allows for generation of a phospho-mimetic.
(A) Hsf1 mutants lacking or mimicking single or clustered phosphorylation sites were assayed for activity in basal and heat shock conditions by measuring the HSE-YFP reporter by flow cytometry. The average of the median of the YFP distribution of three replicates of each mutant is plotted and the error bars represent the standard deviation. The ∆NTA and ∆CTA mutants are known to be hyperactive and impaired, respectively (Sorger, 1990), and serve as positive controls for altered activity. (B) Electrophoretic mobility shift assay showing that recombinant full-length wild type Hsf1 efficiently binds to and shifts HSE-containing DNA. The shift can be reverted with competition with excess unlabeled HSE. However, mutations S225A, which removes the hydroxyl group, and S225D, which partially mimics a phosphate group, reduce DNA binding and thus diminish the shift. The same amount of total Hsf1 was loaded in each lane, and the percent of labeled HSE shifted was quantified. (C) S225 is the only essential serine in Hsf1. Mutation of S225 to alanine renders cells inviable (top right plate). Restoration of S225 as the only serine in a mutant with all the other 152 S/T residues mutated to alanine rescues growth. hsf1∆ cells bearing a URA3-marked copy of wild type HSF1 on a plasmid (pRS316-HSF1) and transformed with the indicated Hsf1 mutant were streaked on 5-FOA plates and incubated at 30°C for two days. (D) Fluorescent microscopy images of wild type Hsf1, Hsf1∆po4 and Hsf1PO4* under basal conditions tagged at their C-termini with YFP showing that all localize to the nucleus. (E) ChIP-seq data (reads per million mapped reads, RPM) for Hsf1 and hsf1∆PO4 under basal conditions plotted along the first 150 kb of chromosome XII.
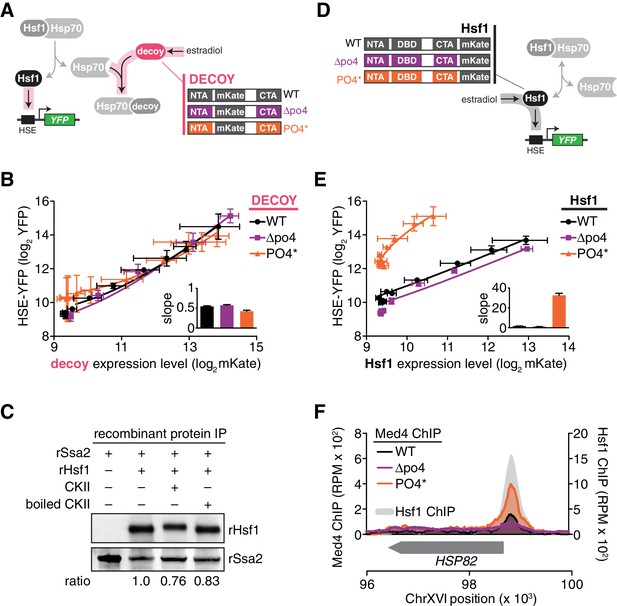
Hsp70 binding and phosphorylation are uncoupled Hsf1 regulatory mechanisms.
(A) Schematic cartoon of decoy constructs based on wild type Hsf1 (WT, black), Hsf1∆po4 (∆po4, purple) and Hsf1PO4* (PO4*, orange). The various decoys will activate endogenous Hsf1 in proportion to their affinity for Hsp70. (B) Measurement of the HSE-YFP reporter by flow cytometry in cells expressing decoy constructs derived from wild type Hsf1, Hsf1∆po4 or Hsf1PO4* as a function of the expression level of each decoy (mKate fluorescence). Data points are the average of median YFP and mKate values for three biological replicates, and error bars are the standard deviation. See Materials and methods for assay and analysis details. The slope of the input-output curves are plotted (inset). (C) IPs of recombinant proteins were performed with 3xFLAG-rSsa2 as bait and analyzed by Western blot. rHsf1 was pre-incubated with ATP alone or in the presence of ATP and either active casein kinase II (CKII) or boiled CKII. Blots were probed with an anti-FLAG antibody to recognize recombinant rSsa2 (top) and with an anti-HIS antibody to recognize recombinant rHsf1 (bottom). The numbers below the blots indicate the normalized ratio of Hsf1/Ssa2. (D) Schematic cartoon of full-length overexpression constructs for wild type Hsf1 (WT, black), Hsf1∆po4 (∆po4, purple) and Hsf1PO4* (PO4*, orange), each with mKate2 fused to its C-terminus. The full-length constructs will activate the HSE-YFP reporter in proportion to their transcriptional activity. (E) Measurement of the HSE-YFP reporter by flow cytometry in cells expressing full length constructs of wild type Hsf1, Hsf1∆po4 or Hsf1PO4* tagged at their C-termini with mKate2 as a function of expression level as in B. (F) ChIP-seq for Med4-3xFLAG-V5, a component of the Mediator complex, in basal conditions in wild type Hsf1, Hsf1∆po4, and Hsf1PO4* cells at the HSP82 locus. Wild type Hsf1-3xFLAG-V5 ChIP-seq was also performed in basal conditions (gray filled curve). See Figure 5—figure supplement 1 for more loci.
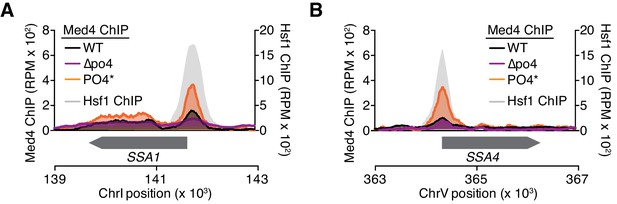
Hsf1PO4* recruits Mediator more efficiently than wild type Hsf1 or Hsf1∆po4.
(A) DNA binding profiles were determined by ChIP-seq for Med4-FLAG-V5, a component of the Mediator complex, in basal conditions in wild type, Hsf1∆po4, and Hsf1PO4* cells. Wild type Hsf1-FLAG-V5 ChIP-seq was also performed in basal conditions. Med4 enrichment in Hsf1, Hsf1∆po4, and Hsf1PO4* cells was plotted as reads per million mapped reads (RPM) at the SSA1 locus (left y-axis). Hsf1 enrichment was plotted as RPM (right y-axis). The lower track shows the position of the SSA1 open reading frame. (B) As in (A), but at the SSA4 locus.
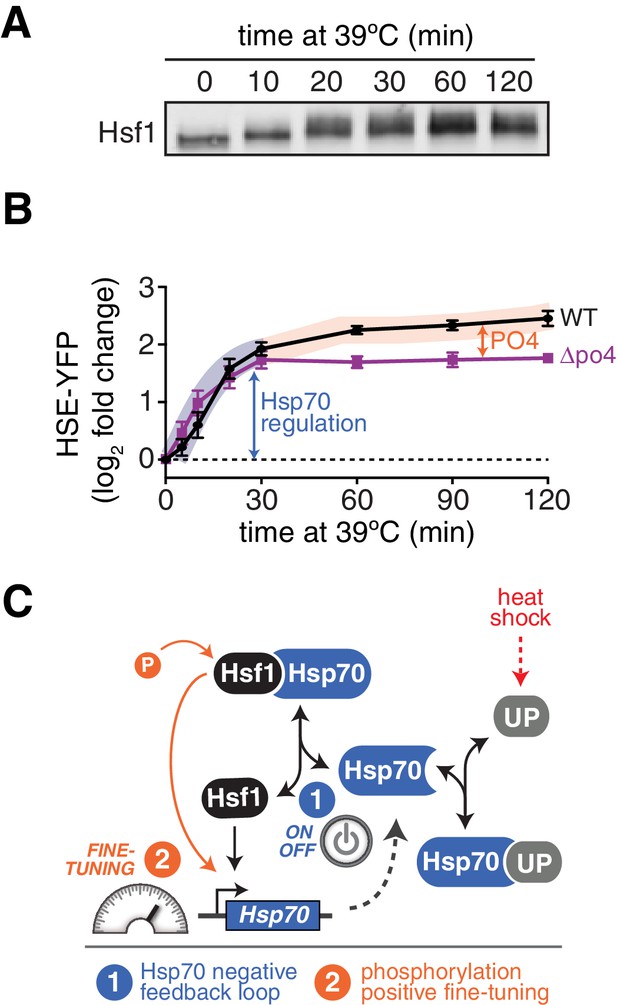
Hsf1 phosphorylation accounts for sustained Hsf1 transcriptional activity during heat shock.
(A) Western blot of Hsf1 phosphorylation, indicated by its mobility shift, over time following temperature upshift. (B) The HSE-YFP reporter was measured by flow cytometry in wild type Hsf1 and Hsf1∆po4 cells over time following upshift to 39°C. Data points are the average of median YFP values for three biological replicates, and error bars are the standard deviation. See Materials and methods for assay and analysis details. The sustained activation attributable to phosphorylation is depicted as the orange segment of the wild type curve. (C) Cartoon schematic of the integrated phosphorylation/chaperone titration model of Hsf1 regulation. Phosphorylation (PO4) increases the transcriptional activity of Hsf1 in a manner uncoupled from the Hsp70 feedback loop.
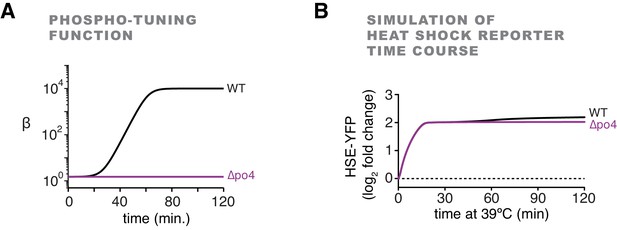
Inclusion of the role of phosphorylation in the mathematical model of Hsf1 regulation.
(A) Phosphorylation gain (β) is incorporated dynamically according to the kinetics determined experimentally. (B) Simulation of the HSE-YFP reporter over a heat shock time course in wild type Hsf1 and Hsf1∆po4 cells using a model that includes both the Hsp70 negative feedback loop and uncoupled activation gain control by phosphorylation (see Materials and methods for details.)
Tables
Model parameter values.
Parameter | Value | Description |
---|---|---|
k1, k3 | 166.8 min−1 a.u.−1 | Client•Hsp70 on-rate constant |
k2 | 2.783 min−1 | Hsp70•HSF1 off-rate constant |
k4 | 0.0464 min−1 | Hsp70•UP off-rate constant |
k5 | 4.64e-7 min−1 | Degradation rate of UP by Hsp70•UP |
β | 1.778 min−1 | Transcriptional activation rate |
Kd | 0.0022 a.u. | Dissociation constant of Hsf1-DNA interaction |
kdil (fixed) | 0 min−1 | Dilution rate of YFP |
n (fixed) | 3 | Hill coefficient |
Model initial conditions.
Species | Initial value (a.u.) | Description |
---|---|---|
[HSP]o | 1 | Free Hps70 |
[Hsf1]o | 0 | Free Hsf1 |
[HSP•Hsf1]o | 1/500 | HSP70•Hsf1 complex |
[HSP•UP]o | 0 | Hsp70•UP complex |
[YFP]o | 3 | Initial YFP concentration |
[UP]o (@ 39°C) | 10.51 | UP concentration at 39°C |
Additional files
-
Supplemental file 1
Yeast strains used in this study.
- https://doi.org/10.7554/eLife.18638.023
-
Supplemental file 2
Plasmids used in this study.
- https://doi.org/10.7554/eLife.18638.024
-
Source code 1
This is a .zip file containing 7 .m files to run the heat shock simulations in MATLAB.
- https://doi.org/10.7554/eLife.18638.025