Large, long range tensile forces drive convergence during Xenopus blastopore closure and body axis elongation
Figures
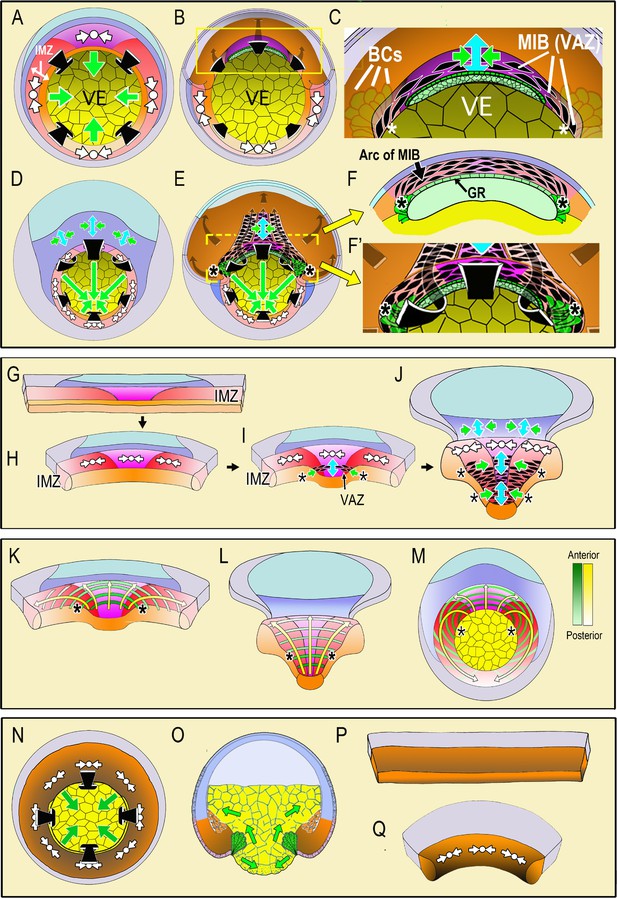
Diagrams illustrate the spatial and temporal aspects of the expression of Convergent Thickening (CT) and Convergent Extension (CE) in embryos and explants.
In the top panel, a vegetal view at the onset of gastrulation (A; Video 1, 285 min) shows expression of CT (white symbols, implying circumferential convergence and thickening perpendicular to the surface of the embryo) in the Involuting Marginal Zone (IMZ), and the effect of its convergence in producing involution (black arrows) and blastopore closure (green arrows, (A). A cutaway of the dorsal sector of a midgastrula, showing post-involution IMZ tissues (B). An enlargement (C) show the onset of CE (green arrows = convergence, blue arrow = the resulting extension). CE is produced by expression of mediolataeral intercalation behavior (MIB) which is expressed initially in the shape of an arc of elongated, intercalating cells (the Vegetal Alignment Zone, VAZ) attached at both ends (asterisks) to the vegetal endoderm (VE) in the region of the bottle cell (BCs). A vegetal view of the late gastrula (D; Video 1, 525 min) shows continued expression of CT in the IMZ. A cutaway of the same stage (E) shows the progressive, posterior expansion of the array of MIB-expressing cells, which advances with the closing blastopore as more cells involute and are added to the array. MIB arcing across the inside of the blastopore (flanked by asterisks) drives CE that acts with CT outside to close the blastopore. Enlargement (F) showing a cross-section at the level of the yellow bar in (E) showing continued expression of MIB anteriorly, which converges and extends the post-involution notochordal and somitic mesoderm along the length of the axis, which lies between the posterior neural plate and the gastrocoel roof (GR) in embryos. In the middle panel, diagrams of explants of the circumblastoporal region show expression of CT movements at the early gastrula (G-H; Video 2, 12:26:04 to 13:46:56), the onset of CE (and MIB) to form the VAZ at the midgastrula stage (I), and the posterior progression of CE/MIB, as cells expressing CT transition into expressing MIB and CE (J; Video 2, after 13:46:56). In explants, MIB/CE pulls the unanchored lateral margin of the somitic mesoderm medially while extending and narrowing the somitic and notochordal mesoderm (I–J). CT feeds cells into the A-P progressive expression of MIB (I–J). Note that MIB occurs in the deep layers of the IMZ, underneath the superficial epithelium, so is not visible in movies of explants shown here, although the resulting CE movements are visible. The bottom panel shows the progressive transition of cells expressing CT to expressing CE and MIB from anterior to posterior (yellow arrows) and the progressive pattern of anterior-to-posterior hoop stress (green hoops) in explants at the midgastrula stage (K), the late gastrula (L) and the presumptive pattern mapped on the midgastrula embryo (M). The last panel shows expression of CT in ventralized embryos, which lack presumptive somitic, notochordal and neural tissue, and thus lacking CE/MIB, and express only CT, which closes the blastopore symmetrically (N-vegetal view; O-sectional view). Explants from such embryos show only CT (P-Q, Video 3). Presumptive tissues are indicated (orange- head, heart, lateroventral mesoderm; magenta- notochord; red- somitic mesoderm; dark blue-posterior neural, hindbrain, spinal cord; light blue-forebrain; gray-epidermis; yellow- vegetal endoderm). Shading from dark to light, where used, indicates progressively more anterior to posterior position, respectively.
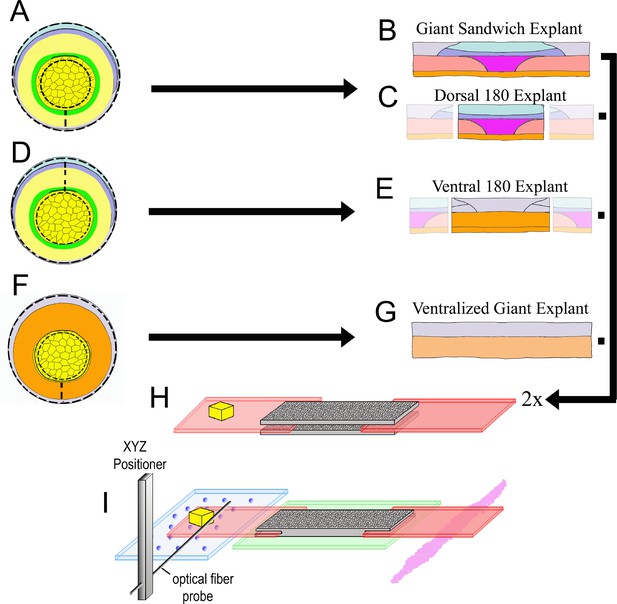
Explant construction.
Giant sandwich explants are made by cutting early gastrula stage embryos mid-ventrally, then vegetally just below the lower edge of the IMZ, such that the bottle cells are included, then animally roughly 30° above the equator (dashed lines, A; see also Figure 2—figure supplement 2). Two such explants are then recombined, inner face to inner face, to make a Giant Sandwich explant (B, H). Giant explants contain presumptive notochordal mesoderm (magenta), somitic mesoderm (red), posterior neural tissue (hindbrain-spinal cord), as well as presumptive brain (light blue), epidermis (grey), and migratory leading edge mesoderm (orange). Dorsal 180° explants are made the same way as standard giant explants, with the right and left quarters cut off (C). Ventral 180° sandwich explants are constructed similarly, except the IMZ is cut dorsally rather than ventrally (dashed lines, (D). Ventralized giant explants are made from UV irradiated embryos, and thus they form no or very limited dorsal tissues (F, G). For mechanical measurements with the tractor-pull apparatus, the two halves of the sandwich are apposed with their inner, deep surfaces next to one another, with fibronectin coated plastic strips, one bearing a raised cleat, inserted at each end (H). The explant is allowed to heal and attach to the strips, and then positioned above a cover slip window in a culture chamber (I). The stationary ‘anchor’ strip is attached to the window with silicone high vacuum grease (magenta), and the explant is placed over an agarose pad (green). The moveable ‘sled’ strip rests on glass beads resting on a cover slip filler layer (blue). An XYZ positioner is used to move a calibrated optical fiber probe, mounted on an aluminum bar, near the cleat, and the imaging chamber, which rests on a motorized stage, is then moved such that the cleat is as close to the probe tip as possible without deflecting the probe (See Video 4).
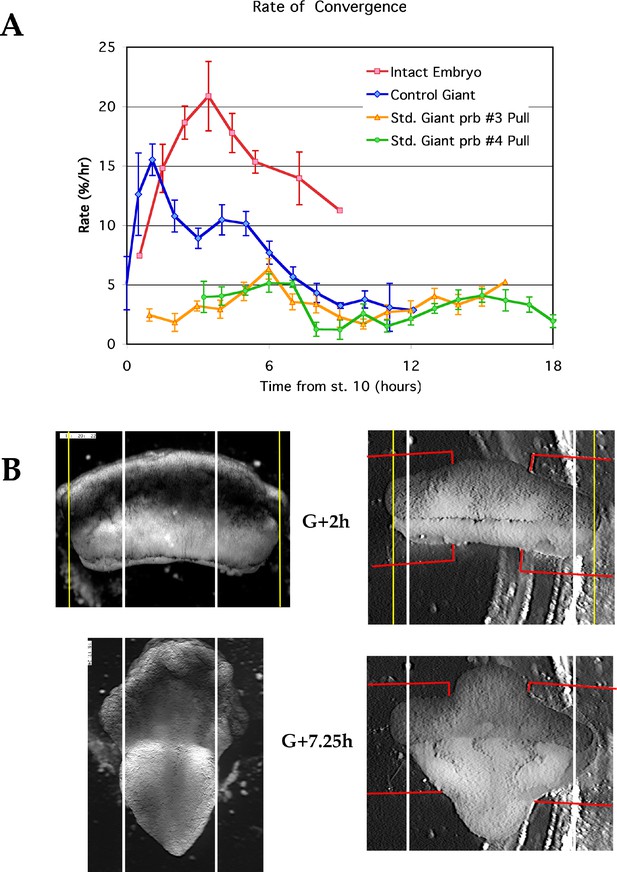
Comparison of convergence along the limit of involution (LI).
(A) The rates of convergence along the circumference or mediolateral extent of the LI in whole embryos, unencumbered giants and giants in tractor pulls under probe #3 or 4. (B) The extent of convergence at the limit of involution by G+7.5hr of giant sandwich explants is retarded in the tractor pull (right), compared to an unencumbered giant (left). Red lines indicate the position of the strips.
-
Figure 2—figure supplement 1—source data 1
Source data for convergence rates.
- https://doi.org/10.7554/eLife.26944.009
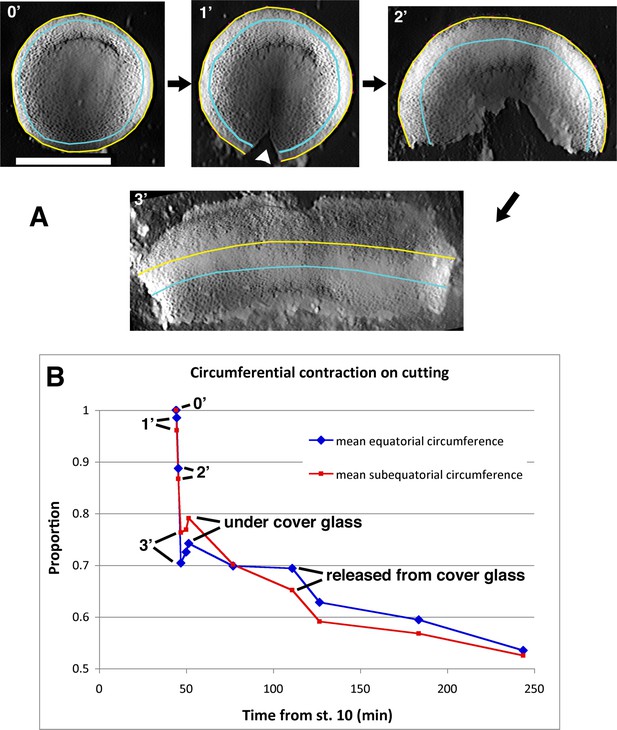
Convergence during explant construction.
Embryos rapidly contract along their circumferential axis upon being cut. An example is shown (A), tracing the length of the equatorial (yellow) and sub-equatorial (cyan) circumference of the embryo, corresponding roughly to the limit of involution and the middle of the marginal zone. Stills are shown immediately before (0’) and one minute after the embryo is initially cut (1’, arrow head), after the removal of further vegetal endoderm at two minutes (2’) and after flattening (3’), in preparation to construct a giant sandwich. Scale bar = 1 mm. Comparing the circumferential lengths to that immediately prior to cutting (B; ‘Proportion’=proportion of initial length) shows a rapid contraction along the mediolateral (former circumferential) axis. The explant in A was combined with another such explant to make a giant sandwich and gently pressed together under a cover glass to heal.
-
Figure 2—figure supplement 2—source data 1
Source data for Convergence during explant construction.
- https://doi.org/10.7554/eLife.26944.011
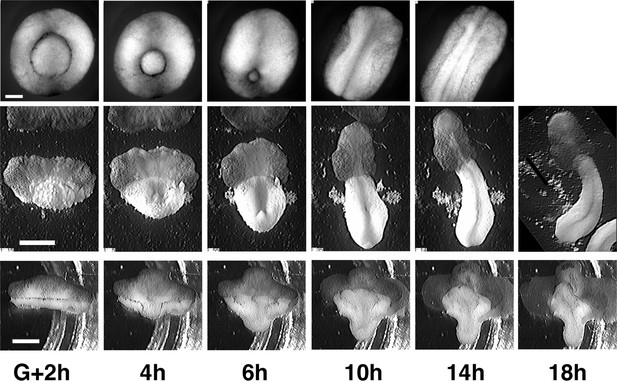
Comparison of morphogenesis in embryos, giants and tractor pulls.
Intact embryos (top) are compared to unencumbered giants (middle) and standard giants in tractor pulls (bottom). Time of controls are as indicated at bottom. Scale for embryos = 250 μm, for giants and tractor pulls = 1 mm.
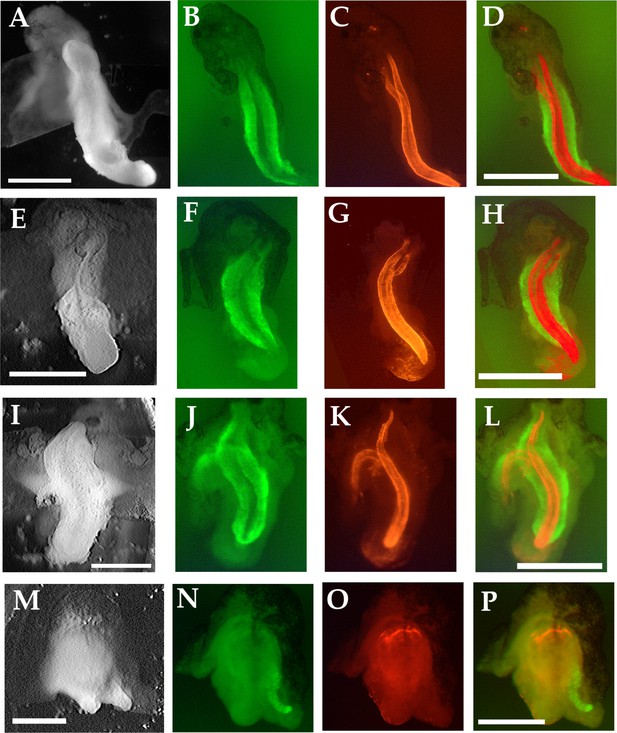
Notochordal and somitic tissue in tractor pull explants.
Sandwich explants used in tractor pulls were fixed after the force measurement assay was finished (stage 25–30) (A, E, I, M) and stained for somitic tissue with the 12/101 antibody (B, F, J, N) and for notochordal tissue with the Tor70 antibody (C, G, K, O). The two staining patterns are also shown juxtaposed D, (H, L, P). In a standard giant explant (A–D), a dorsal 180° explant (E–H) and a standard giant explant that had additional tension imposed on it (0.6μN, at G+5hr) (I–L), both somitic and notochordal staining are evident. In a ventral 180° explant, (M–P) a small amount of somitic tissue at the lateral edge of the explant is observed, but no notochordal tissue. Tor70 signal in O is typical of that seen in the remnant of the blastocoel cavity of ventralized embryos with no axial tissues, and does not correspond to notochordal tissue. Scale bars = 1 mm.
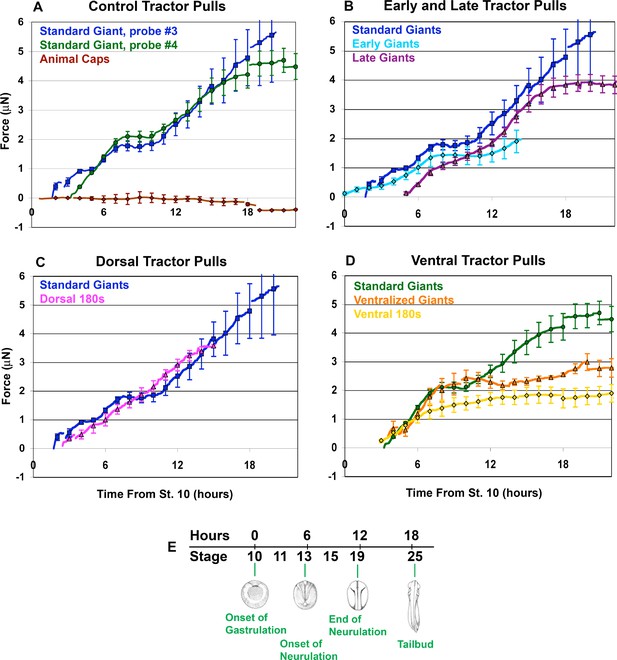
Force vs. time traces for tractor pulls.
Mean force production over time is indicated (A-D; solid lines). Time is measured from the onset of gastrulation at stage 10, and the correspondence with developmental stage is shown (E). Hourly means are shown as symbols, with standard errors of the hourly means indicated. The onset of individual traces represents the time at which the sled was initially pulled against the probe, with the exception of the Animal Cap explants. All pulls were against probe #3, except ‘Standard Giants, probe #4’ (green, A and D) and the ‘Ventralized Giants’ and ‘Ventral 180 s’ (orange and yellow respectively, (D). The force trace for Standard Giant explants vs. probe #3 (blue) is included for all graphs except where only probe #4 was used (D). Gaps in force traces represent points at which different numbers of explants are included in the mean force trace. Controls (A) included Standard Giant sandwich explants (vs. probe #3, dark blue, n = 2 up to 2 hr, n = 4 through 15 hr; vs. probe #4, green, n = 6 through 12 hr, n = 5 through 18 hr), Animal Cap sandwich explants (purple, n = 4 from 5 to 17 hr, 2 to 3 otherwise). Early and Late tractor pulls (B) include Early Giant explants (from stage 10; light blue, n = 3) and Late Giant explants (from stage 12.5; purple, n = 5). Dorsal tractor pulls (C) include Dorsal 180° explants (pink, n = 3). Ventral tractor pulls (D) include UV ventralized explants (orange, n = 3 at 4 hr, 4 from 5 to 7 hr, 5 from 8 to 9 hr and 4 from 10 to 20 hr), ventral 180° explants (yellow, n = 3 at 3 hr, 4 from 4 to 20 hr) and are compared to Standard Giant explants vs. probe #4.
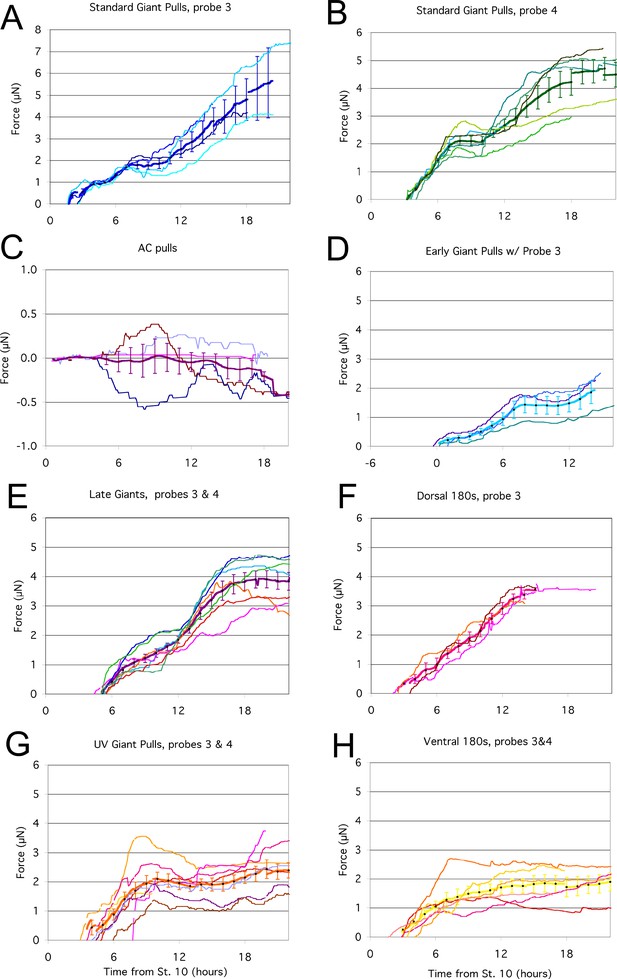
Plots of all the individual force traces.
For each tractor pull condition, the force traces of each pull are plotted (thin lines), as well as the mean of the pulls (thick line), with error bars representing standard error. The accuracy of the plots along the time axis is approximately ±30 min. For Late Giants, UV Giant Pulls and Ventral 180° pulls, plots using both probes #3 and #4 are shown, as no significant difference was seen between the two probes for these conditions.
-
Figure 3—figure supplement 1—source data 1
Source data for all individual force traces; data for each panel (A–H) broken down by sheets within the Excel file.
- https://doi.org/10.7554/eLife.26944.017
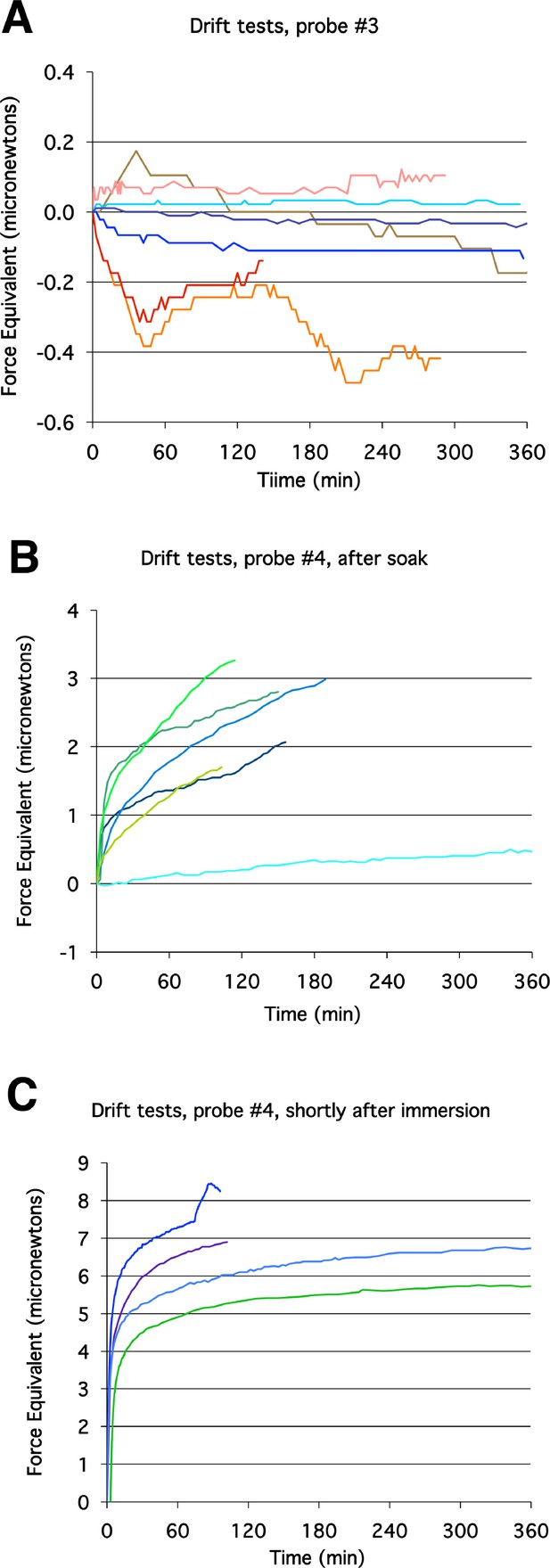
Probe drift tests.
Probe drift over time was assayed with the probe immersed in culture media or water. Probe movement was translated into force equivalents, to determine how much it would influence tractor pull and stress-relaxation test measurements. Representative plots with probe #3 (A) showed substantially less tendency to drift than with probe #4 (B, C). Drift was greatest immediately after immersion (C).
-
Figure 3—figure supplement 2—source data 1
Source data for probe drift tests; data for each panel (A–C) broken down by sheets within the Excel file.
- https://doi.org/10.7554/eLife.26944.019
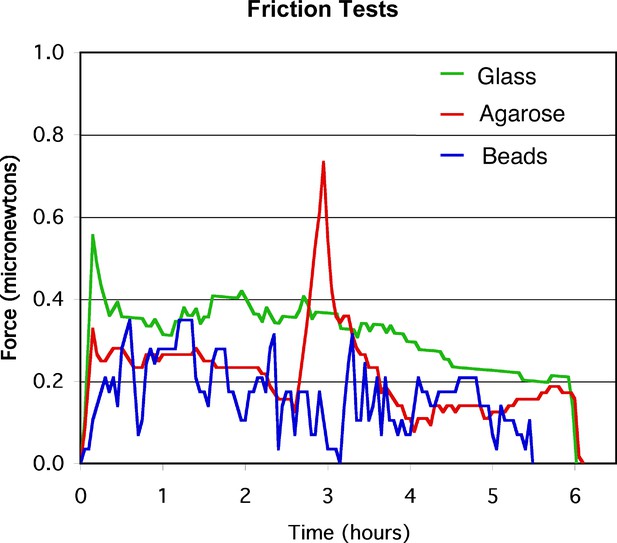
Sled friction tests.
Representative force plots for sled friction on three different substrates: cover glass in a 0.1% solution of BSA, a 1% agarose pad, or 100 μm glass beads.
-
Figure 3—figure supplement 3—source data 1
Source data for sled friction tests.
- https://doi.org/10.7554/eLife.26944.021
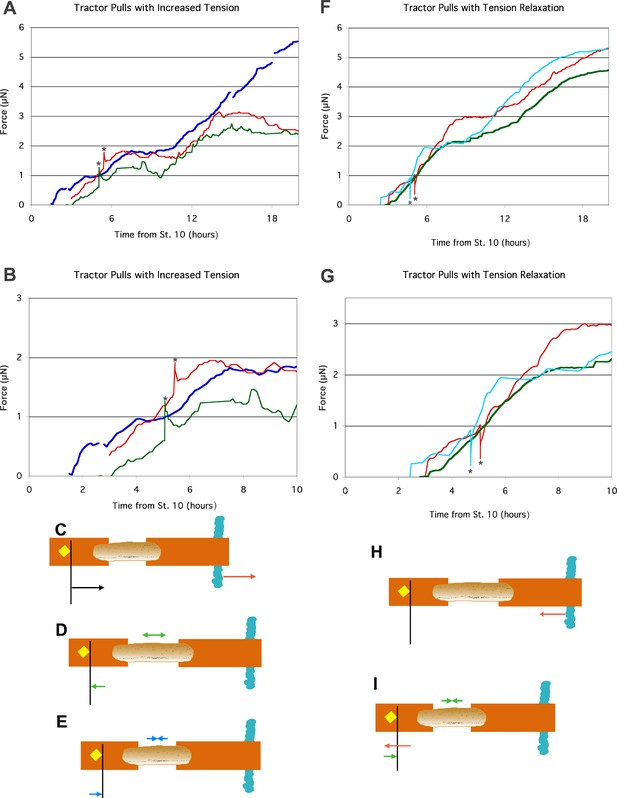
Response of explants in tractor pull after tension increase or relaxation.
The anchor strip was moved 50 μm away from the probe to increase tension on the explant by 0.5 to 0.6 μN (A, B). Two examples (green and red in A, B) are compared to mean control values (blue in A, (B). The anchor strip was moved 100 μm toward the probe to relax tension on the explant by 0.7 μN (F,G). In all cases, the point of maximum deflection is indicated by *. Two examples (blue and red in F, G) are compared to mean control values (green in F, (G). Close-ups of the time period around the tension adjustment are shown in B, G. Tension increase via anchor movement (red arrow; C), resulted in an immediate increase in the deflection of the probe (black arrow; C); as the explant underwent stress-relaxation (double headed green arrow; D), tension was reduced (green arrow; D). Eventually, continued convergence (double headed blue arrow; E) added additional tension to the system (blue arrow; E). Tension reduction via anchor movement (red arrow; H), resulted in an immediate decrease in the deflection of the probe (red arrow; I); rapid elastic recoil (<5 s) of the explant as stored elastic energy was released resulted in convergence of the explant (double headed blue arrow; I), and increased deflection of the probe (blue arrow, I). Further morphogenic convergence of the explant occurred subsequently (as in E).
-
Figure 3—figure supplement 4—source data 1
Source data for Increased tension or Relaxation during tractor pulls, panels A,B and F,G on different sheets within Excel file.
- https://doi.org/10.7554/eLife.26944.023
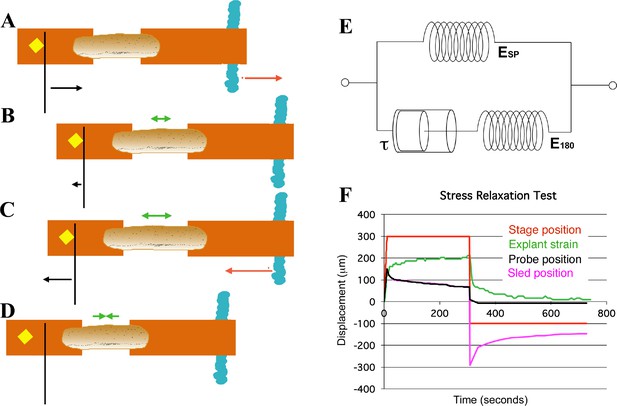
Schema of movements and measures involved in stress-relaxation test.
Start position, with cleat adjacent to probe. Stage is moved 300 microns (A, red arrow) against probe, to impose stress, with resulting probe deflection (A, black arrow). The explant shows an instantaneous strain (B, green arrow), then exhibits viscoelastic decay, or ‘relaxation’ over time (C, green arrows), reducing the deflection of the probe (B, black arrow), until tension equals residual stiffness (in practice, E180). Finally, the stage is moved back 400 microns (C, red arrow), which de-stresses the explant and allows the probe to return to its starting position (C, black arrow). The explant shows elastic recovery (D, green arrows). (E) A model of the explant as a viscoelastic material, with springs representing instantaneous (ESP) and residual (E180) stiffness, and a dashpot representing the viscosity, with relaxation time (half-time of decay), tau (τ). In an example of a stress-relaxation test (F), the stage, to which the anchor strip (left) is attached, is moved (F, red line) to impose a stress, by pulling the cleat against the probe (as in A, (B). This imposes a strain (F, green line) on the explant, and deflects the probe (F, black line), as in (A). The explant continues to undergo strain, as in C, until it reaches its residual stiffness. The movement of the sled (F, magenta) initially parallels that of the probe, until the stage is moved away from the probe (as in C, D) at about 300 s, at which point the explant shows elastic recovery of the imposed strain (as in D), pulling the sled with it. In order to estimate Esp and τ we used non-linear regression curve fitting of the stress relaxation phase (B, C, green arrows; F, green line).
-
Figure 4—source data 1
Source data for Stress Test Example, Panel F.
- https://doi.org/10.7554/eLife.26944.025
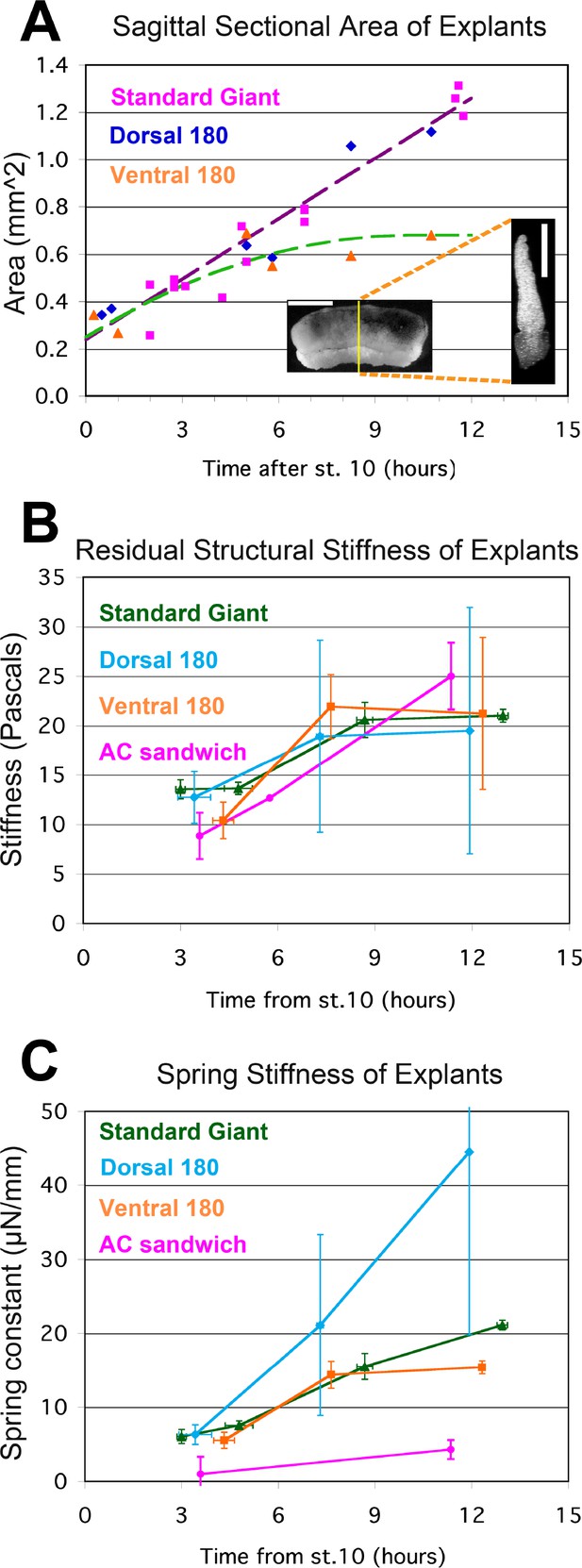
Stress-relaxation tests.
The sagittal sectional area (SSA) for different kinds of unencumbered explants was determined at points throughout gastrulation and neurulation from confocal z-series of RDA labeled explants (A, inset; scale bar = 1 mm in intact giant, 0.5 mm for sagittal cross section (at yellow line in giant)). Standard giant (magenta squares) and Dorsal 180° sandwich explants (blue diamonds) show similar progressions of SSA; a regression on both (violet dashed regression line, 0.085 mm2/hour * (hours after G0)+0.24 mm2) was used to estimate stage specific SSA for both kinds of explants in stress-relaxation tests. The increase in SSA for V180° explants tended to plateau by about 8 to 10 hr, so a first order polynomial regression (green dashed line, −0.0042 mm2/hour2 * (hours after G0)2 + 0.085 mm2/hour * (hours after G0) +0.25 mm2, or 0.68 mm2 at 10 hr or later) was use to estimate the stage specific SSA for V180° explants in stress-relaxation tests. The SSA of animal cap explant sandwiches was a consistent 0.16 mm2. Estimated SSA, measured force on the probe at 180 s, and measured proportional strain on the explant at 180 s were used to determine the stiffness (E180) at several times during gastrulation and neurulation (B). Standard giant sandwich explants (dark green line), as well as Dorsal 180° (light blue) and Ventral 180° (orange) sandwich explants and animal cap sandwich explants (Yellow) were tested. In order to compare the force-bearing capacity of different tissues, a bulk spring stiffness (Force at 180 s / Strain at 180 s) was plotted (C). Error bars = standard error of the mean, n’s = 3 to 6, except where no error bar is shown, where n = 1.
-
Figure 5—source data 1
Source data for Sagittal Sectional Area (A) and Structural Stiffness and Spring Stiffness (B, C), on separate panels within Excel file.
- https://doi.org/10.7554/eLife.26944.029
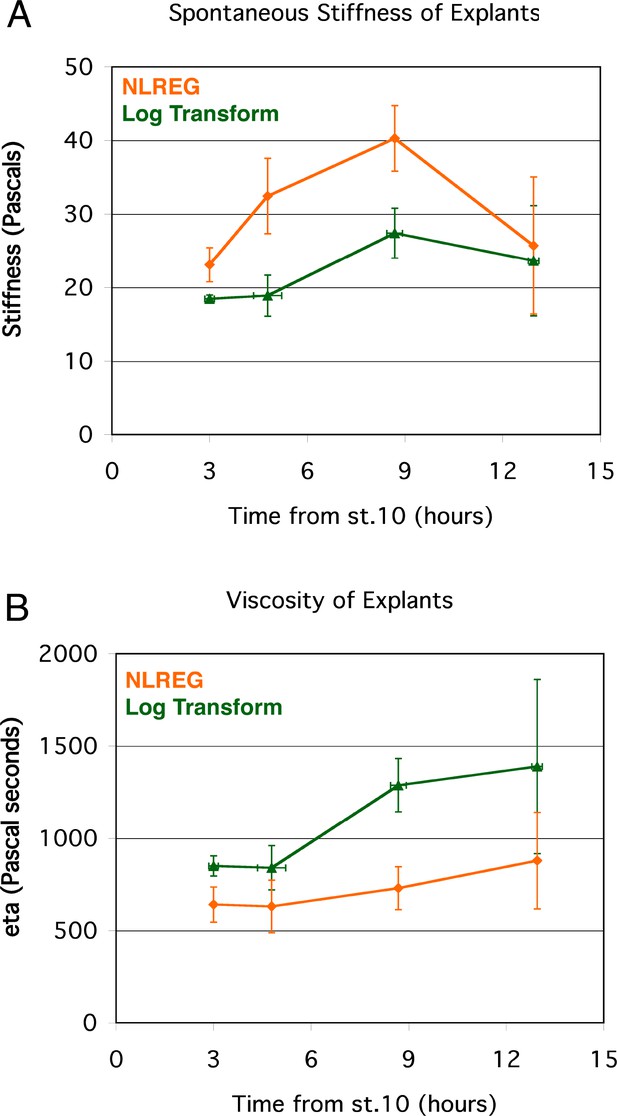
Instantaneous Structural Stiffness and Viscosity of standard giant sandwich explants.
Parameters for the spring and dashpot model (Figure 4E), calculated using two different methods (NLREG or Log Transform).
-
Figure 5—figure supplement 1—source data 1
Source data for Instantaneous Stiffness and Viscosity measures.
- https://doi.org/10.7554/eLife.26944.028
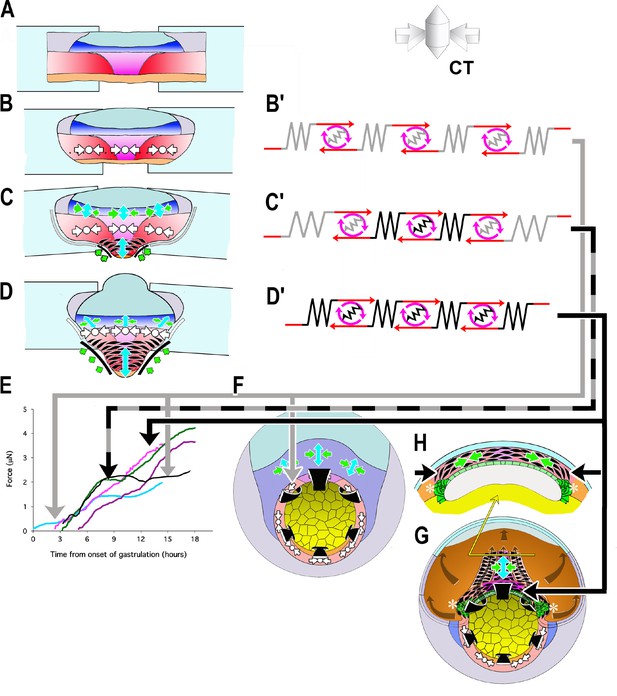
Model of how the convergence forces measured in the tractor pull are related to modules of cell behavior in explants and embryos.
Early convergence forces are generated largely by the CT machine (CT symbols, (B–D). The CT symbol implies ML tensile force, represented by the inward pointing arrows, and radial compressive force, represented by the dot and indicating force directed in and out along the radial axis of the embryo (see 3D representation of CT). As CE begins, MIB (fusiform, black cells) progressively replaces the CT machine from presumptive anterior to posterior (CE symbol: green convergence, blue extension arrows, (C, D) while CT continues in more posterior tissues (CT symbol, C, D). At or shortly after the onset of mesodermal MIB, MIB and CE begin in the posterior neural tissue (blue tissue, CE symbols, (C, D). Thus the IMZ tissues express a changing combination of CT and CE as development progresses. We represent CT and CE as modules, expressing distinctive spring constants (grey or black coils) and motor strengths (red and grey or black symbols), with the lighter spring and motor indicating CT and the darker CE (B’–D’). Force plots in (E) are means from Figure 3: blue = Early Giants; pink = Dorsal 180 s; green = Standard Giants, Probe #4; purple = Late Giants; black = ventralized giants). Initially, up to about G+2hr, the entire IMZ is comprised of CT-modules (B’), which represents the situation and generates the forces seen in the first two hours of early pulls (follow grey arrow from B’ to E, blue line). These forces likely approximate force generated in the preinvolution (uninvoluted) IMZ of the whole embryo (follow the gray arrow to F, CT symbols). As MIB begins, a CE module lies centrally, flanked by CT-modules in series (C’) with lateral edges attached to the strips (C), which represents the situation and generates the forces measured from G+2 to 10.5hr, including the period during the plateau in standard pulls (follow black/gray dashed arrows to E, green line), but with an increasing contribution from CE vs. CT modules after G+2hr. As more cells express MIB, the number pulling in parallel increases, increasing the spring constant and motor strength of the CE module. Because the VAZ forms as an arc it does not initially pull directly on the attachment strips (C; green arrows at edge) but on the intervening CT modules. The nascent CE module is initially small and weaker than the adjacent CT modules, but becomes larger over time, resulting in both increasing spring constant and motor strength. This eventually overpowers the shrinking CT modules, such that their convergence is reduced (C’, more open coils), which dissipates some of the tension generated by the CE module, and thereby contributing to the plateau. In contrast, in the embryo, the CE (MIB) module is, from the beginning, always anchored to the endoderm at both ends, with only an indirect connection to CT modules in the lateral and ventral portion of the MZ (not shown in F; see H, G, asterisks). Thus CT acts as a continuous but diminishing ring of converging tissue outside the blastopore, while CE-expressing tissue inside the blastopore, primarily in series with the relatively inert endodermal tissue, acts in parallel with this ring. The transient decline rather than plateau in the rate of force increase during late pulls (E, purple line) can be explained by a larger domain comprised of CE modules and smaller domains comprised of CT modules, compared to standard control pulls at the onset of the plateau, such that not all force generated by the CE domain was absorbed by reduced convergence in the CT domain. Once MIB progresses laterally to points of attachment with the strips (D, D’), the decline ends; this represents the situation during the second phase of force increase (follow black arrow to E, magenta line and to H, (G). At this point, all the force generated by CE and MIB in posterior tissues are transmitted to the attachment strips, while, with the progression of MIB posteriorly, force from more anterior tissues is transmitted progressively more indirectly, at an angle (green arrows; D). Dorsal pulls show no plateau, because they contain little or no tissue comprised of CT modules (D’) by the onset of the plateau (follow solid black arrow to E, pink line). Ventralized giant pulls show maximal force generation at the level of the plateau, being comprised of only CT modules (B’; follow the gray line to E, black line).
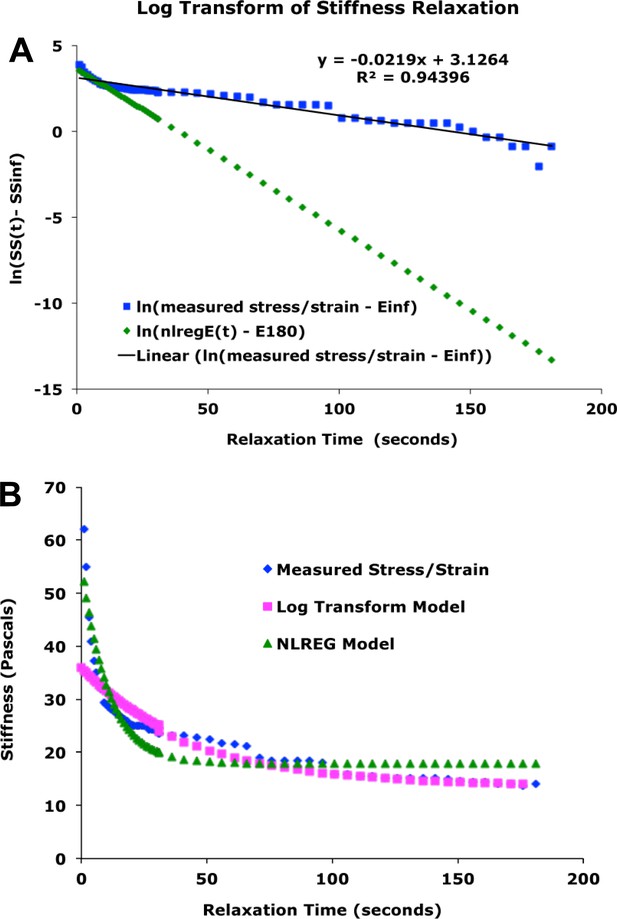
Comparison of methods for estimating parameters.
(A) Example of estimation of parameters from linear regression on log transform of time-dependent stiffness data. Blue: plot of ln(measured SS(t) – measured SS(180) * 0.97)). Black: linear regression plot on log transformed data. green: plot of ln(SSsp * e^(-t/tau) – E180), with parameters as estimated by NLREG. (B) Comparison of Model vs. Measured Stiffness for the case in A, above. Blue: measured time dependent structural stiffness (SS(t)). Magenta: plot of E(180) + SS(sp) * e(-t/tau), with the later two parameters based on linear regression on log transform, as in A, above. Green: plot with all parameters from NLREG.
-
Appendix 4—figure 1—source data 1
Source data for parameter estimation method examples.
- https://doi.org/10.7554/eLife.26944.038
Videos
Movie comparing gastrulation and BP closure in normal (left) and ventralized (right) embryos.
Timestamp shows minutes elapsed. The movie begins during cleavage stages and runs through neurulation. Gastrulation begins at control stage 10 (G0) at 285 min, stage 10.5 (G+2hr) at 405 min.
Movie showing unencumbered giant sandwich explant.
Timestamp shows hh:mm:ss. Stage 10.5 (G + 2 hr) at about 13:34:57.
Movie showing ventralized giant sandwich explant.
Timestamp shows hh:mm:ss
Movie showing standard giant in tractor pull apparatus.
Probe, sled and anchor are indicated in the first frame. Timestamp shows hh:mm:ss. Movie begins shortly after st. 10.5 (G + 2 hr)
Tables
Convergence and strain.
Negative strains indicate convergence. ‘Standard Pull’ refers to standard giant explants that have developed tension within the tractor pull apparatus.
Average rate of convergence (μm/min) (n) | Average rate of convergence (%/hr) (n) (SEM) | Strain of dorsal tissue (%/hr) (n) (SEM) | Strain of LV tissue (%/hr) (n) (SEM) | Shear w.r.t. attachment strips (%/hr) | |
---|---|---|---|---|---|
Intact embryo, LI, 2 to 7 hr | 10 (2 to 7) | 17.5 (2 to 7) (1.8) | |||
Giant explant, unencumbered, LI, 0 to 7 hr | 5 (3 to 10) | 10 (3 to 10) (1.5) | |||
Standard pull, probe 3 (2 to 7.5 hr) | 4.1 (4 to 5) (0.7) | −7.2 (6) (1.5) | 1.1 (6) (1.5) | 3.7 | |
Standard pull, probe 3 (7.5 to 10.5 hr) | 2.5 (5) (0.8) | −7.0 (6) (2.1) | 1.7 (6) (1.9) | 2.5 | |
Standard pull, probe 3 (10.5 to 15.5) | 3.7 (5) (0.7) | −5.5 (6) (1.6) | −0.5 (6) (1.3) | 3.2 | |
Standard pull, probe 4 (2.5 to 7.5 hr) | 4.5 (2 to 4) (0.7) | 3.1 | |||
Standard pull, probe 4 (7.5 to 10.5 hr) | 1.7 (4) (0.8) | 1.7 | |||
Standard pull, probe 4 (10.5 to 16.5) | 3.0 (4) (0.6) | 2.1 |
Estimates of force per cell and tensional stress within effective sagittal section area (SSA) (deep mesoderm only).
Based on a mean cell sectional area of 625 nm2.
Time from onset of gastrulation (hours) | Force (μN (n,±SEM)) | Effective SSA (mm2 (n,±SEM)) | Estimated force/cell (nN) | Force per effective SSA (Pascals) |
---|---|---|---|---|
1 | 0.25 (3, 0.08) | 0.12 (1, n/a) | 1.3 | 2.1 |
2.1 | 0.31 (3, 0.09) | 0.11 (4, 0.014) | 1.7 | 2.8 |
2.9 | 0.49 (4, 0.16) | 0.13 (4, 0.018) | 2.3 | 3.8 |
4.3 | 0.94 (4, 0.04) | 0.27 (3, 0.024) | 2.2 | 3.5 |
6.5 | 1.6 (5, 0.11) | 0.41 (3, 0.020) | 2.5 | 3.9 |
11.8 | 2.6 (6, 0.19) | 0.68 (3, 0.051) | 2.4 | 3.8 |
Additional files
-
Transparent reporting form
- https://doi.org/10.7554/eLife.26944.032
-
Appendix 4—figure 1—source data 1
Source data for parameter estimation method examples.
- https://doi.org/10.7554/eLife.26944.038