Super-resolution imaging of synaptic and Extra-synaptic AMPA receptors with different-sized fluorescent probes
Figures
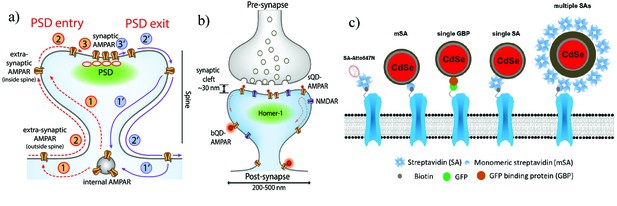
Schematic diagrams of the dynamics of AMPARs and labeling strategies for AMPARs.
(a) AMPAR entry into and exit from the PSD. From an internal pool, AMPARs either enter the spine via membrane diffusion or through direct insertion (1) and then diffuse towards the PSD (2). From here, it enters the PSD in a rate-limiting step according to the slot theory (3). Similarly, the AMPAR may exit (1’ – 3’) the PSD in a similar manner. (b) Schematic of synapse labeled with a small (sQD) or big (bQD) quantum dots. Since the synaptic cleft width is only about 30 nm, the size of the fluorescent probe may sterically hinder entry into synaptic clefts. (c) Schematics of AMPA receptors with a variety of different sized probes and with various linkers. The smallest is the organic fluorophore linked by a Streptavidin (SA); next smallest in size are the small quantum dot linked by a monomeric SA (mSA), or an anti-GFP nanobody (called GBP) attached to a GFP-AMPAR, or a (single) SA; largest are commercial quantum dot which are attached to multiple SAs.
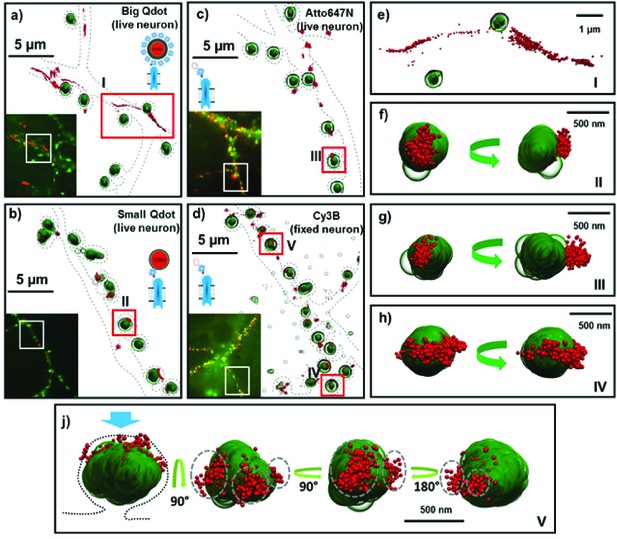
Fluorescent and super-resolution imaging of AMPARs and synapses.
Big and Small quantum dots and organic fluorophores attached to AMPAR (red) on live cultured neurons with the PSD protein, Homer1c, labeled (green). Big (or commercial) quantum dots (a, e) bound to AMPA fail to get in the synapse and are spread extra-synaptically. Small quantum dots (b, f) and with small organic fluorophores (Atto647N) (c, g), bound to AMPAR, are stuck in nanodomains within the synapse. Fixing the neurons and using a Cy3 organic dye (d) can get the number of nanodomains per synapse; mostly there is just one (h), but occasionally there are multiple nanodomains (j). The dotted lines in a-d represent the morphology of dendrites. The green arrow (f–j) indicates the direction of synaptic cleft and the dotted line represents the shape of the synapse. Each image is the rotated structure of the synapse as represented by curved arrow. The gray dotted lines in j indicate the nanodomain of the AMPA receptors. For super-resolution images, the microscope stage drift and chromatic aberrations are corrected.

TEM image for Si mask which has fiduciary markers.
Fiduciary markers are every 8 µm or 16 µm and total pattern size is 18 mm and 18 mm. Each marker size of the round column is 1 µm height and 1 µm diameter. We then made the mold using PDMS and stamped the pattern on the coverslip.
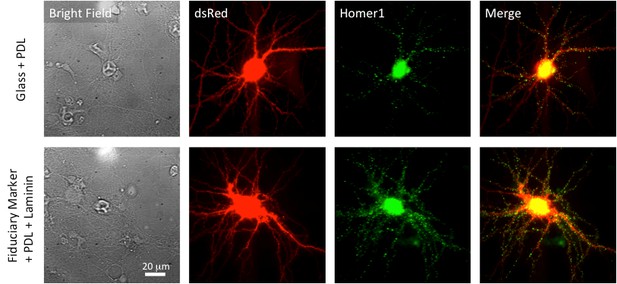
The upper panel represents cultured neurons on the poly-D-lysine (PDL) coated glass coverslip.
The first column is bright field images. Red fluorescence is free ds-Red and green is homer1-mGEOS. The last column is overlay of ds-Red and Homer1-mGEOS. The lower panel is cultured neurons on the fiduciary marked coverslip with PDL and laminin coating. Before coating PDL/Laminin, fiduciary marked coverslips were treated with oxygen plasma to make hydrophilic surface. The neuron cultured on the fiduciary marked coverslip looks very similar to the neuron that cultured on the glass coverslip in terms of their shape and number spines. All images are taken with x60 water immersion Nikon objective lens (Plan Apo VC 60x WI).
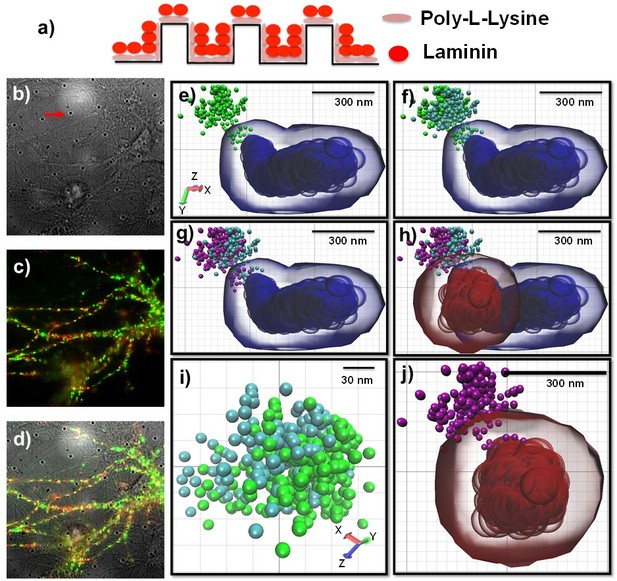
Schematics for culturing neurons on the fiduciary marked coverslips and presentative images for correcting stage drift and chromatic aberration.
(a) Fiduciary marked coverslips need to be coated with poly-(L/D)-lysine and laminin, sequentially. These coating helps neurons adhere on the surface. (b) a bright field image which shows neurons on the fiduciary marked coverslips. Periodic black dots are fiduciary markers as indicated by a red arrow. (c) fluorescence images for neurons. There is no auto-fluorescence at the illumination of visible wavelength. (d) overlay image of (c) and (d). (e) a representative reconstructed image for a synapse (homer1, blue) and the trace of a receptor (AMPA receptor, green) without correcting stage drift and chromatic aberration. (f) corrected chromatic aberration. Using the mapping function to correct chromatic aberration, the red color channel transposed to the green channel. Cyan color balls represented the trace of AMPA receptors after correcting chromatic aberration. (g) Purple balls represented the trace of a receptor after correcting stage drift. This trace (purple balls) has minimal error caused by stage drift and chromatic aberration. (h) correcting stage drift for the image of a synapse. Blue color shows before correction and red color shows after correcting stage drift. (i) zoom-in image showing difference between before and after correcting chromatic aberration.
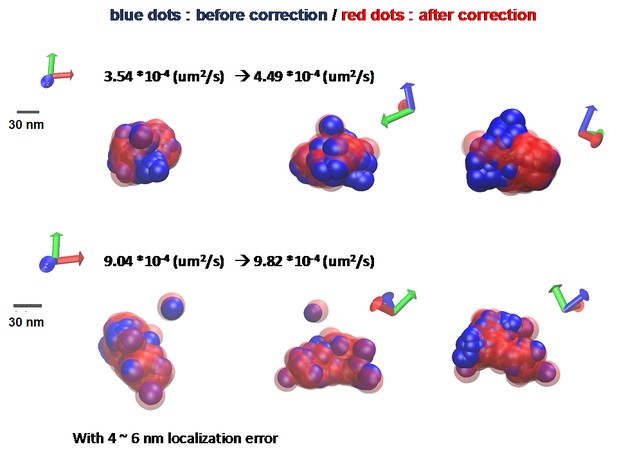
The difference of diffusion coefficient with and without correcting the stage drift.
Even though the tracking AMPA receptors takes for 50 s, the stage drift affects measuring diffusion coefficient. At maximum, there is more than 10% difference between before and after correcting the stage drift. With astigmatism for three dimensional imaging, the localization precision is about 4 nm for x and 6 nm for y.
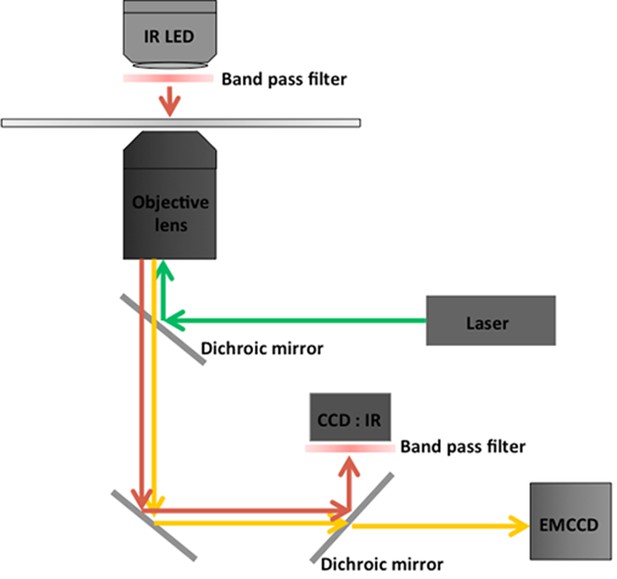
Commercially available microscopes have a perfect focus system (PFS), which normally uses IR LED light, in order to reduce the drift along z-axis.
In the case of Nikon (Ti-eclipse), the wavelength of the LED is 870 nm with 800 nm short pass dichroic mirror. We then selected 760 nm to avoid interference to the PFS. The band-width of the 760 nm LED (ELJ-750–629, Roithner-LaserTechnik GmbH) is from 725 nm to 775 nm and we added the band pass filter (769/41, OD6 blocking) at LED as well as at the IR camera in order to reduce the interference between the LED for the PFS and the IR LED for fiduciary markers.
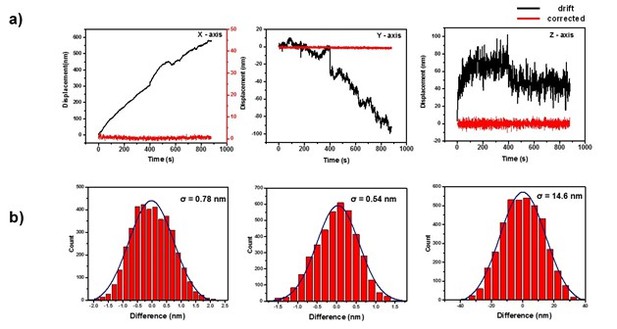
Traces of the stage drift and the precision of the correction.
(a) All three axis show significant stage drift over time. Black lines represent the stage drift and the stage drift was corrected by using fiduciary markers. Red lines represent the corrected traces of the stage. (b) The precisions are less than 1 nm for x and y axis and 14.6 nm for z-axis.
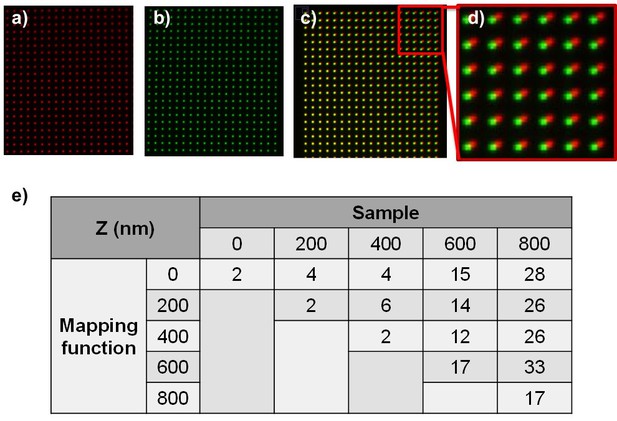
Correcting chromatic aberration using nanohole pattern.
We made 100 nm size hole every 1.5 µm on the silver coated glass coverslip. When illuminating white light through the nanohole pattern, each color image can be taken using a band pass filter.
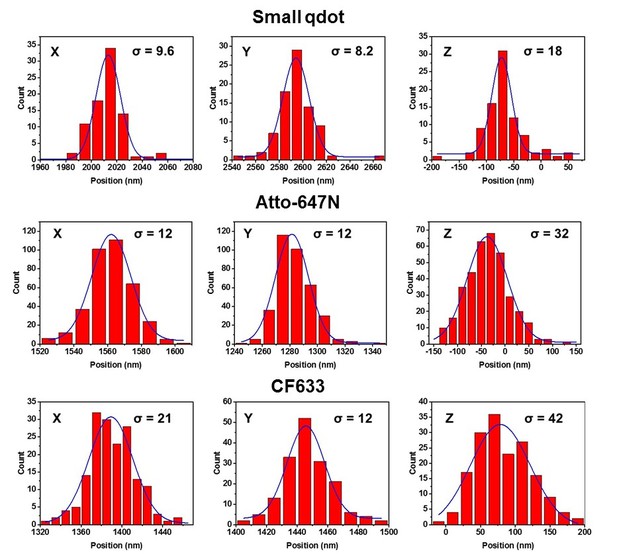
The precision of each fluorophores.
The histograms were obtained from the localization particles over 200 frames with 50 ms exposure time. All these measurements were done with astigmatism for 3D using a cylindrical lens.
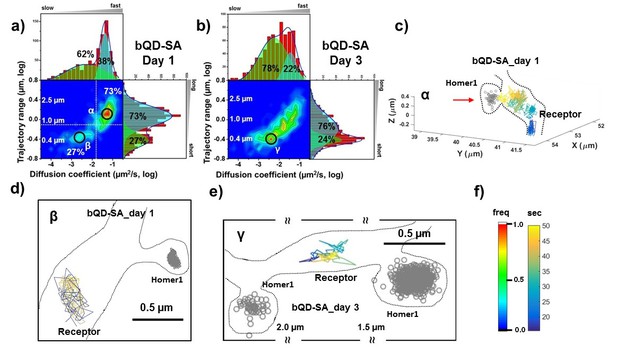
AMPARs are not synaptic as measured by bQDs.
Two-dimensional plot of the diffusion coefficient and the trajectory range of AMPA receptors using big qdots (a) measured day 1 (n=483 traces, 8 cells, 4 independent dissociation, 1 dissociation per week) and (b) day 3 (n=715 traces, 4 cells, 4 independent dissociation, 1 dissociation per week) after transfection. White dot lines are for 10−1 μm2/s (0.79 μm) and 10-1.75 μm2/s (0.018 μm2/s)) for the trajectory range and the diffusion coefficient, respectively. Right upper side represents the population of fast diffusive receptors with longer trajectory and the left lower side represents the population of slow diffusive receptors with shorter trajectory. For all histograms, the distribution could be deconvoluted by Gaussian functions. Cyan colored Gaussian functions represent the fast diffusive receptors in diffusion coefficient and longer diffusive population in trajectory range. Green shaded Gaussian functions indicate slower and shorter diffusive receptors. On Day 1, the fast and long-distance peak (α) corresponds to ~73% of all molecules with ~10-1.25 µm2/s (α) and ~1.3 µm, while the slow and short diffusion population (β) corresponds to ~27% of all molecules with ~10-2.5 µm2/s and ~0.4 µm. On day 1, the α peak is not in the synapse but diffusive relatively freely along the synapse as represented in (c). Dotted lines in (c) represent the morphology of dendrite and the red arrow in c) indicates the synaptic cleft. The remaining 27% on day 1 in Figure 3a has a short trajectory and >10 x slower diffusion than the α peak. The majority of the slow diffusive receptors on both Day one and Day three are located away from synapse as in (d) and (e). At day three after transfection, the diffusion coefficient and trajectory range measured by using big qdots are significantly changed, probably due to crosslinking. (f) shows the color coding for population of heat maps and for time traces for a single receptor.
-
Figure 3—source data 1
Diffusion coefficient and trajectory range measured by SA-bQD at day 1.
- https://doi.org/10.7554/eLife.27744.015
-
Figure 3—source data 2
Diffusion coefficient and trajectory range measured by SA-bQD at day 3.
- https://doi.org/10.7554/eLife.27744.016
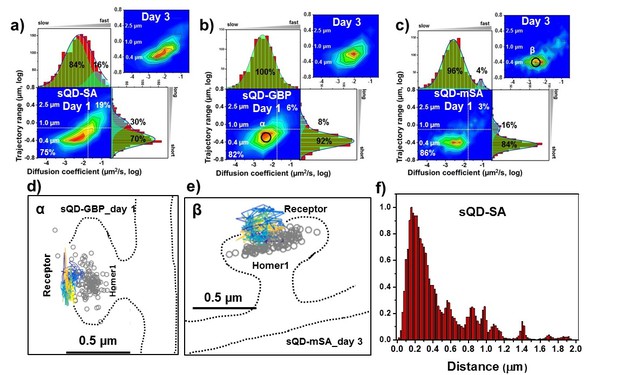
AMPARs are synaptic as measured by sQDs.
Two-dimensional plot of the diffusion coefficient and the trajectory range of AMPA receptors using small qdots with various tags, (a) SA (n=1453 (11 cells, 6 independent dissociation, one dissociation per week) and 1523 (13 cells, 6 independent dissociation, 1 dissociation per week) traces for day 1 and 3, respectively), (b) GBP (attached to a GFP-AMPAR) (n=524 (5 cells, 4 independent dissociation, 1 dissociation per week) and 537 (7 cells, 4 independent dissociation, 1 dissociation per week) traces for day 1 and 3, respectively), and (c) mSA (n=750 (8 cells, 4 independent dissociation, 1 dissociation per week) and 701 (11 cells, 6 independent dissociation, 1 dissociation per week) traces for day 1 and 3, respectively), measured day 1 and day 3 after transfection. White dot lines are for 10−1 μm2/s (0.79 μm) and 10-1.75 μm2/s (0.018 μm2/s)) for the trajectory range and the diffusion coefficient, respectively. Upper right side represents the population of fast diffusive receptors with longer trajectory and lower left size represents the population of slow diffusive receptors with shorter trajectory. Fitting the graphs via two Gaussian functions, the cyan represent the fast diffusive receptors (~10-1.25 µm2/s) in diffusion coefficient and longer diffusive population (1 µm) in trajectory range; green indicates slower (~10-2.5 µm2/s) and shorter diffusive receptors (~400 nm). For all three tags, 75–86% are slowly diffusive and have a short trajectory. Insets in a), (b), and (c) are two-dimensional plot for diffusion coefficient and trajectory range, measured day three after transfection. All insets are similar to the result, measured day 1. Slow diffusive receptors are mainly localized in synapses as in (d) (peak α in b) and (e) (peak β in c). Color-coding for heat maps and for time traces for a receptor is the same as Figure 3f. (f) histogram for the distance between receptors and the center of Homer1c. The histogram shows that 75% of receptors are localized within 0.5 µm of Homer1c (91% within 1.0 µm).
-
Figure 4—source data 1
Diffusion coefficient and trajectory range measured by mSA-sQD at day 1.
- https://doi.org/10.7554/eLife.27744.023
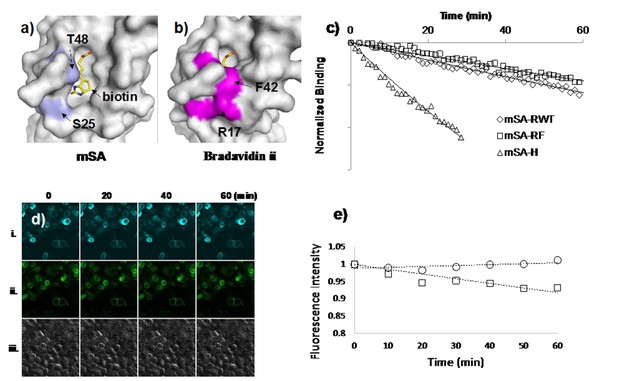
Characterization of monomeric streptavidin and conjugation with small quantum dots.
(a) The biotin binding pocket of mSA is exposed to the solvent, which results in rapid dissociation of bound biotin. In contrast, (b) the binding pocket of bradavidin ii is surrounded by R17 and F42, which surround the biotin and limit access of water molecules. Double mutations, S25R and T48F, in mSA may similarly shield bound biotin from solvent molecules and slow the rate of dissociation. (c) The dissociation rate, koff, of mSA variants was measured by fluorescence polarization spectroscopy using biotin-fluorescein as the ligand. Fluorescence polarization from the ligand is reduced upon dissociation from mSA. Normalized binding is computed by subtracting the polarization of free dye, , and dividing by the polarization difference between bound and unbound states, . Fitting normalized polarization to an exponentially decaying curve results in the off rate, koff. (d) To test for cell labeling of mSA, mSA-RF-GFP was used to label biotinylated AP-CFP that transfected on HEK293. Images of i/ii/iii show CFP, GFP, and bright field images. The fluorescence intensity of GFP and CFP does not show much difference. (d) and (e) Test specificity of mSA-RF-eGFP to AP-CFP on HEK293. After the cells were fixed, mSA-RF-EGFP was added for 30 min to label biotinylated proteins. Unbound mSA-RF—EGFP was washed away and the labeled cells were imaged for (i) CFP or (ii) GFP fluorescence every 10 min for 1 hr. (iii) DIC, differential interference contrast. The total fluorescence intensity of each image was computed at different time points by importing RGB jpg images into MatLab and by summing over green and blue channels (CFP) or over green channel only (GFP).
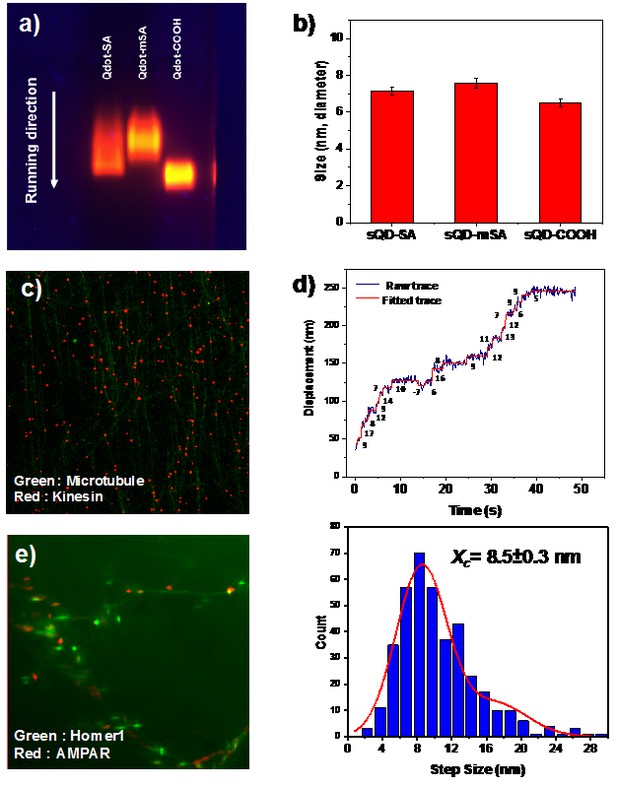
(a) Agarose gel image for SA-, mSA-, and carboxylated- small qdots and (b) histogram for their size.
(c) Motor proteins (kinesin) labeled by mSA-small qdot (red dots) are on microtubules (green, HiLyte 488). (d) Kinesin walking trace and their step size which shows 8.5 nm. (e) mSA-small qdots label AMPA receptors on neurons (green fluorescence is Homer1). mSA-small qdots are specifically bound to AMPA receptors (red).
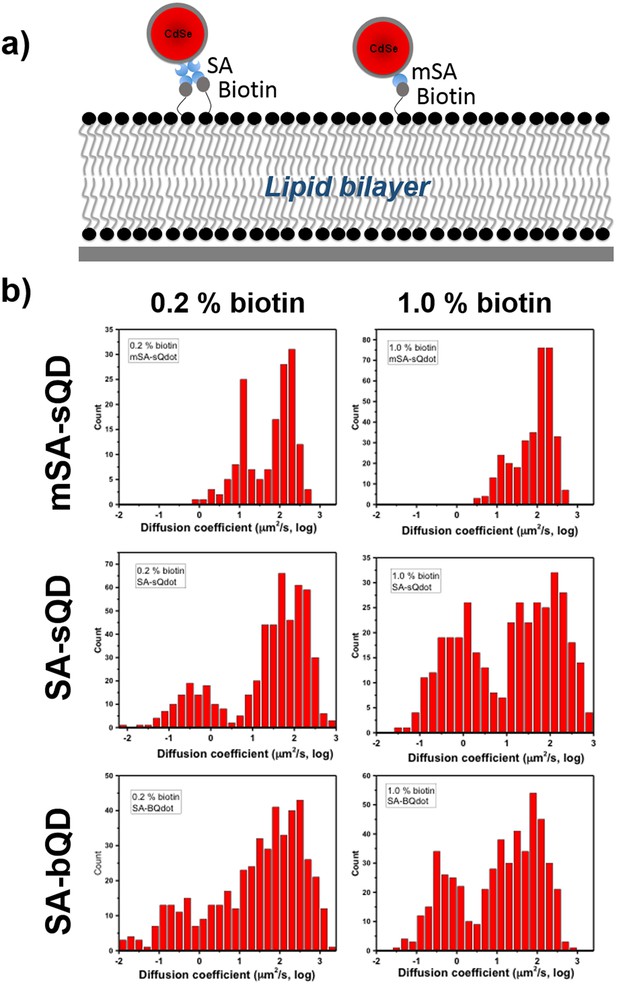
Measuring the diffusion coefficient of labeled qdots on the lipid bilayer in order to examine the possibility of cross-linking.
(a) represents the schematics of cross-linking of streptavidin(SA) conjugated qdots. SA conjugated qdots have more chance to cross-link multiple target molecules on a single qdots because a SA has four binding sites, but a monomeric SA has only one binding site. Thus, monomeric streptavidin intrinsically excludes binding multiple molecules on a single qdot. (b) histograms of diffusion coefficients measured at different conditions which are at 0.2% and 1.0% biotin concentration of lipid bilayer using three different qdots, such as SA-Bqdot(commercial qdots), SA-small qdots, and monomeric SA-small qdots.
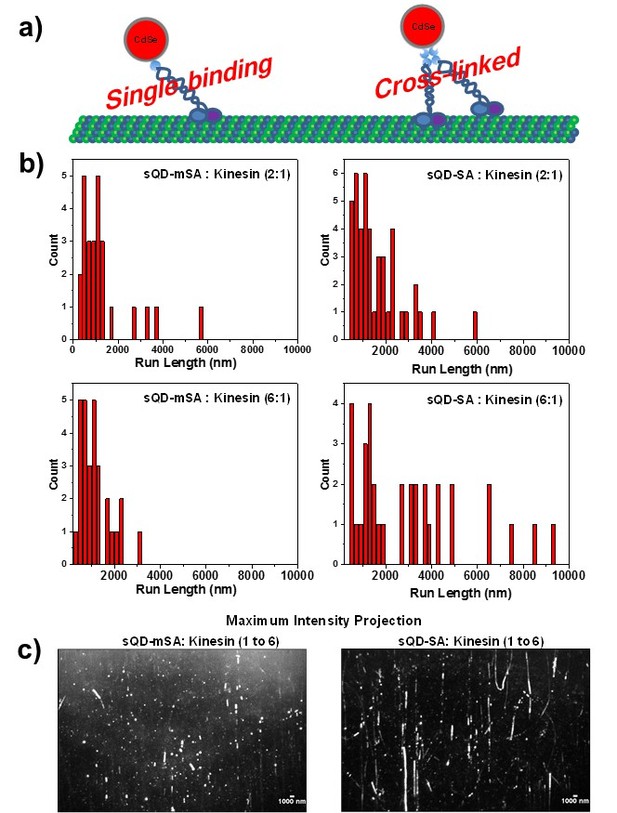
Measurement the run-length of kinesin using mSA-small qdot and SA-small qdot.
(a) schematics to show the possibility of crosslinking multiple kinesin on qdots. Since a mSA is monovalent, there is no possibility to bind multiple kinesin. By contrary, a streptavidin has four binding sites for biotin, so that, even though each small qdot has only one streptavidin, SA-small qdots can bind more than one kinesin. (b) the measurement of the run length of kinesin at two different concentration ratio between qdots and kinesin. (c) kymograph for kinesin walking traces with 1:6 (small qdot: kinesin) ratio.
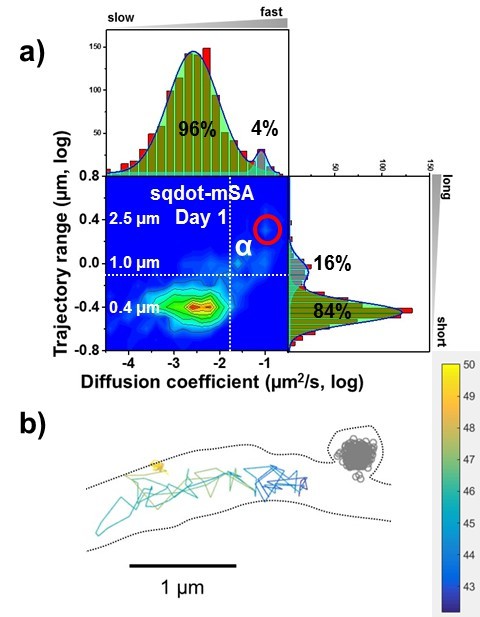
Free diffusive AMPA receptors labeled with mSA-sQD.
In the 2D plot (a), higher diffusion coefficient and longer trajectory range (right upper side in the 2D plot) means AMPA receptors freely diffuse on the dendrite as in (b).
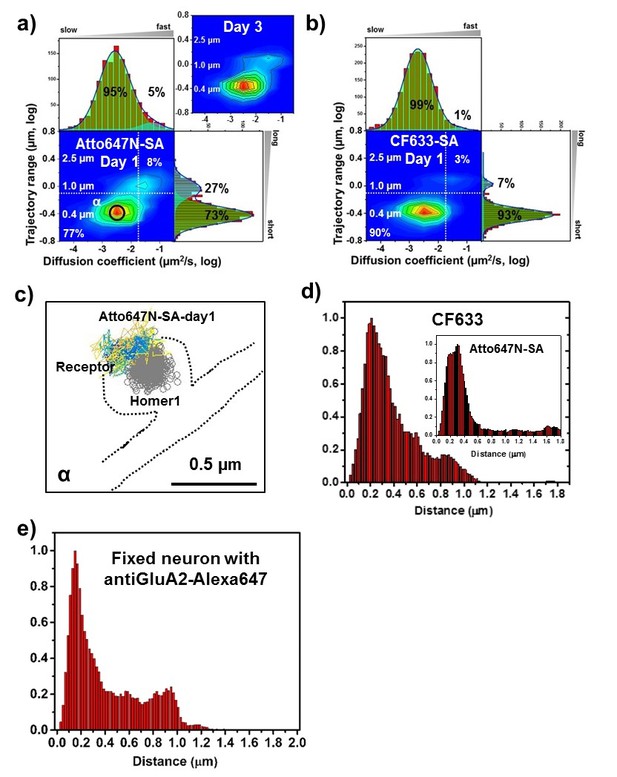
AMPARs are synaptic as measured by organic fluorophores.
Two-dimensional plot of the diffusion coefficient and the trajectory range of AMPA receptors using 1 nM organic dyes for labeling, (a) Atto647N measured at day one as well as day 3 (n=1152 (9 cells, four independent dissociation, one dissociation per week) and 3063 (7 cells, three independent dissociation, one dissociation per week) traces for day 1 and 3, respectively) and (b) CF633 measured at day 1 (n=1599 traces, 11 cells, four independent dissociation, one dissociation per week). White dot lines are for 10−1 μm2/s (0.79 μm) and 10-1.75 μm2/s (0.018 μm2/s)) for the trajectory range and the diffusion coefficient, respectively. Upper right side represents the population of fast diffusive receptors with longer trajectory and lower left size represents the population of slow diffusive receptors with shorter trajectory. Cyan colored Gaussian functions represent the fast diffusive receptors (~10-1.25 µm2/s) in diffusion coefficient and longer diffusive population (1 µm) in trajectory range. Green shaded Gaussian functions indicate slower (~10-2.5 µm2/s) and shorter diffusive receptors (~400 nm). For both organic dyes, more than 90% have a small diffusion constant and have a 73–93% have a short trajectory (based on the diffusion coefficient graph). (c) We find that they are moving slowly next to the Homer1c. (d) histogram for the distance between receptors and the center of Homer1c on a live neuron labeled with AMPAR-SA-CF633 or Atto647N inset. The histograms shows that 81% and 78% of receptors, respectively, are localized within 0.5 µm. The color-coding for heat maps and for time traces for a receptor is the same as Figure 3f. (e) Histogram of distance between native AMPAR (labeled with antibody-Alexa647 against GluA2) and Homer1c-mGeos, and fixed. Here 71% of the receptors are within 0.5 µm of the Homer1c (96% within 1.0 µm), very similar to (d).
-
Figure 5—source data 1
Diffusion coefficient and trajectory range measured by SA-CF633 at day 1.
- https://doi.org/10.7554/eLife.27744.029
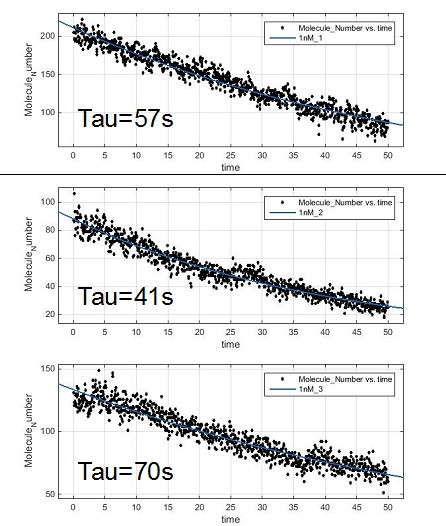
Measurement of the photobleaching time of SA-atto647N.
At 1 nM of SA-atto647N, the average photobleaching time is about 61 s. Thus, we were able to measure the diffusion coefficient of AMPA receptors using SA-atto647N.
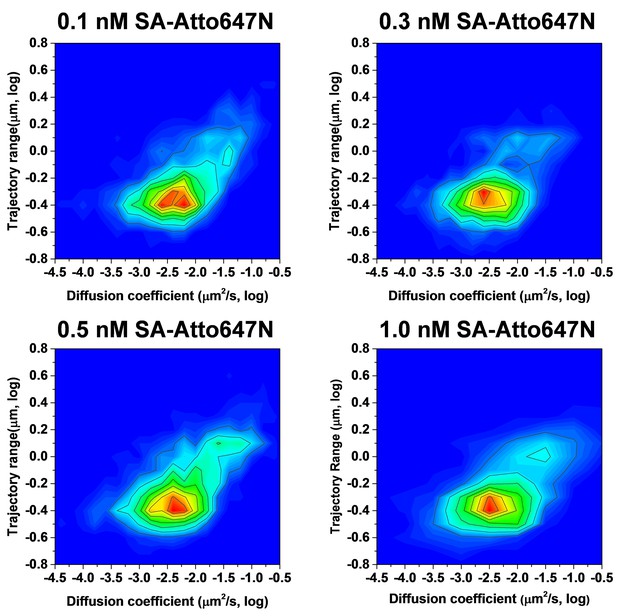
Two dimensional heat maps for the diffusion coefficient and trajectory range measured at various concentrations (0.1, 0.3, 0.5, and 1.0 nM) of SA-Atto647N.
The distribution of diffusion coefficient and trajectory range does not change at different concentrations.
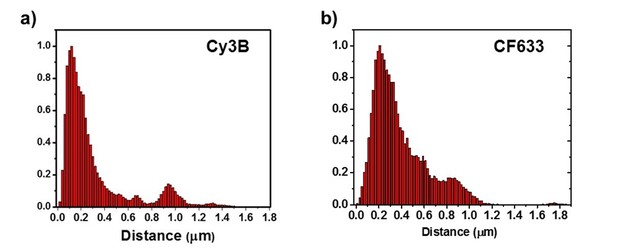
Measured the distance between the receptors and the center of Homer1c.
(a) For Cy3B on fixed neurons, 85% of receptors are localized within 0.5 µm.
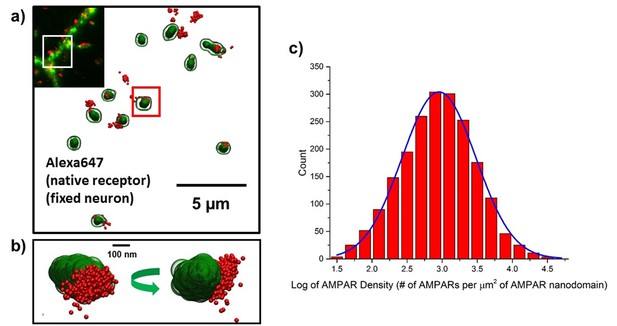
Image AMPA receptors using the antibody of GluA2.
(a) fluorescence image for endogenous AMPA receptors and homer1c. (b) Zoom-in image of one of the spines. (c) histogram of the density of AMPARs in nanodomains. Approximately 900 molecules per µm2 were observed in each domain. 856 of the cluster of AMPARs were analyzed (n=1826 clusters, 11 cells).
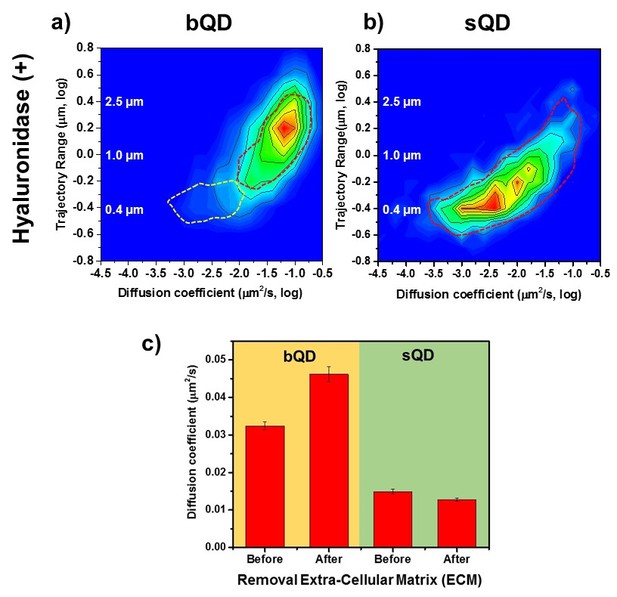
Lateral Diffusion of Synaptic AMPAR is Unaffected by Removal of ECM.
Measurement the diffusion coefficient and trajectory range of the AMPA receptors using bQD-SA and sQD-SA after enzymatically removing extra-cellular matrix (ECM) using Hyaluronidase (100 unit/ml). (a) and (b) are 2D plots for diffusion coefficient and trajectory range measured with big qdots (n=2566 traces, 6 cells, 4 independent dissociation, 1 dissociation per week) and small qdots (n=1103 traces, 6 cells, 4 independent dissociation, 1 dissociation per week), respectively, after removal ECM. Dot lines circles represent the diffusion coefficient and trajectory range of Hyaluronidase (-) (taken from Figure 3a and Figure 4a, respectively). When using big qdots, the population of slow diffusion and short trajectory range disappears with treating with Hyaluronidase. However, with sQDs, there is no significant difference. The color-coding for heat maps is the same as Figure 3f. (c) The average diffusion coefficient, measured by using big qdots, after the removal of the ECM increases 1.4 fold from that at the condition of Hyaluronidase(-). On the contrary, the average diffusion coefficient at Hyaluronidase (+) is almost the same as without treating Hyaluronidase.
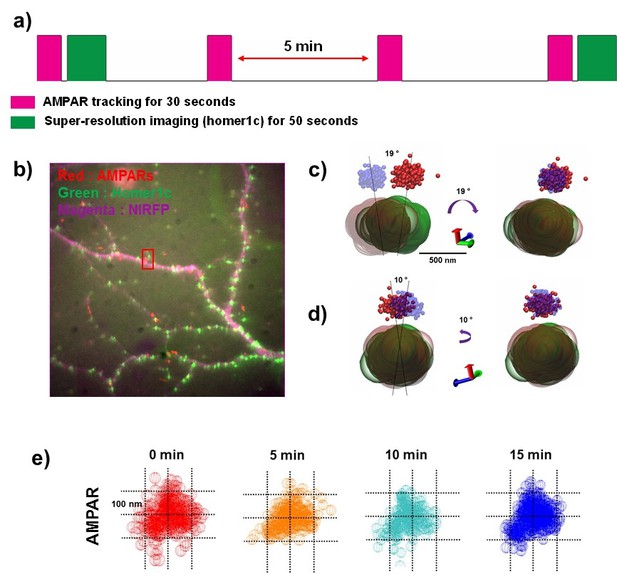
The dynamics of the AMPA receptor domain in synapses.
Example of AMPAR and Homer1c moving very little over 15 min. (a) Schematics of long-term measurement dynamics for the AMPA receptor domain. AMPA traces were recorded for 30 s (50 ms exposure time) every 5 min (0 min ~15 min) and super-resolution images for synapses were taken at the beginning and the end of the 15 min period. (b) fluorescence image for synapses (homer1c-mGeos, green), AMPARs (sQD, red), and cell morphology (free-NIRFP, magenta). (c) and (d) show the difference of the position of an AMPAR and a synapse (Homer1c) from 0 min (AMPAR-red, Homer1c-green) to 15 min (AMPAR-blue, Homer1c-brown) at two different direction. At 0 through 15 min, when AMPAR and Homer1c are rotated 19° in a clockwise direction as shown in c) and 10° in a counterclockwise direction as shown in d), they overlap. (e) shows a stable nano-domain throughout the 15 min period. Red (0 min), orange (5 min), cyan (10 min), and blue (15 min) balls represent the AMPA receptor at various points in the trace of that AMPA receptor.
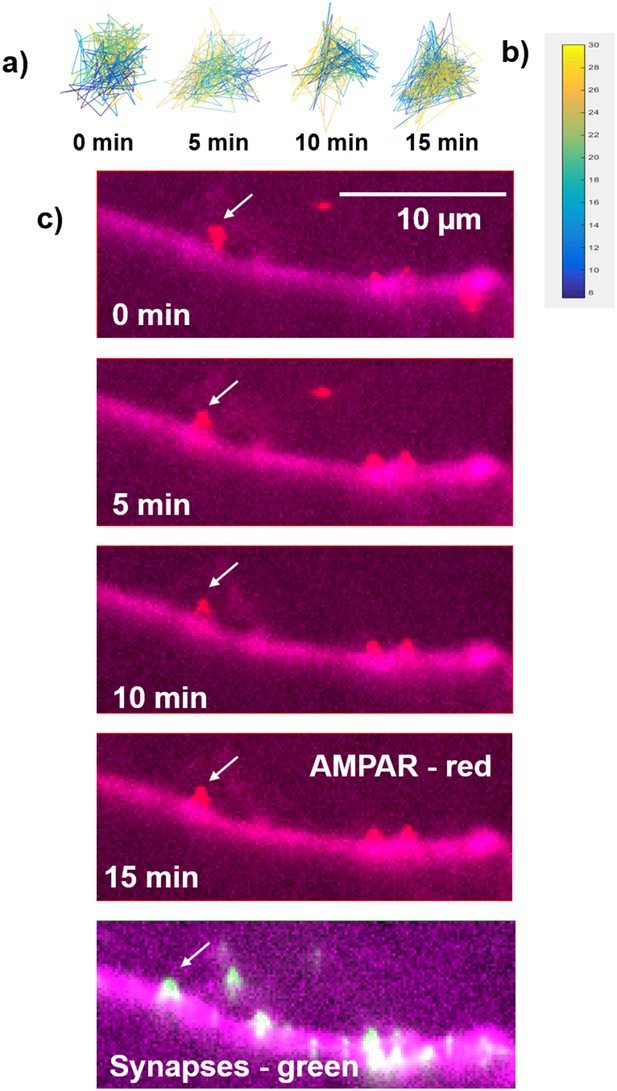
The time trace of nanodomains (Figure 7h) and the time-lapse of fluorescent images (Figure 7).
(a) the time trace of an AMPAR which is shown in Figure 7 and (b) the color coding for time traces (c) Fluorescent images of AMAPRs (sQD, red) and cell morphology (free-NIRFP, magenta) and the image of synpases (homer1c-mGeos, green) with cell morphology image. White arrows represent the receptor and the synapse that is shown in Figure 7.
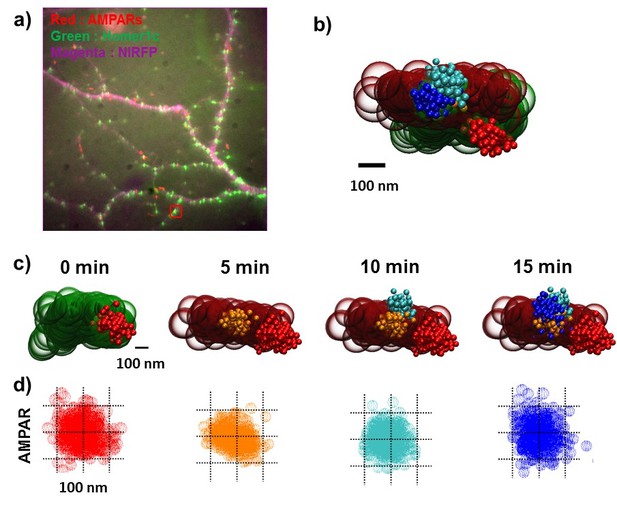
Another type of dynamics of the AMPA receptor domain in synapses.
(a) fluorescence images for synapses (homer1c-mGeos, green), AMAPRs (sQD, red), and cell morphology (free-NIRFP, magenta). (b) and (c) the representative synapse which shows slightly moving nano-domain for 15 min. Initially, an AMPA receptor (Red - 0 min) was localized very edge of spine (green balls-beginning) and then it (orange −5 min, cyan-10 min, and blue-15 min) moved to the center of homer1c cluster (brown-ending). (d) the projection image of the AMPA receptor trace. The nano-domain does not change much over time.
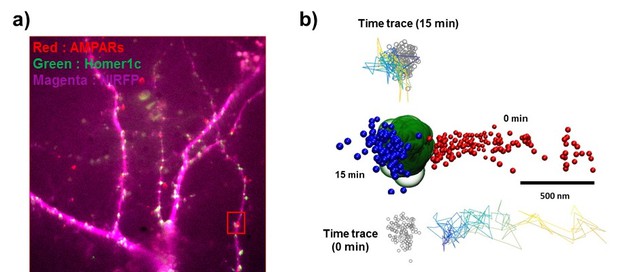
The last type of dynamics of the AMPA receptor domain in synapses.
(a) fluorescence images for synapses (homer1c-mGeos, green), AMAPRs (sQD, red), and cell morphology (free-NIRFP, magenta). (b) the representative AMPA receptor which initially freely diffused near a spine, ending up interacting with a domain in the synapse. The emission of the qdot at 5 and 10 min was too defocused (using the cylindrical lens for 3D imaging) to be detected, so the traces are not shown.
Tables
Summary of AMPA receptor diffusion that are synaptically-bound, measured with various fluorophores and tags.
https://doi.org/10.7554/eLife.27744.013Fluorophore | Size (nm) | Tag | Diffusion coefficient (μm2/s) | Trajectory range (nm) (±width) | Synaptic receptors (%) |
---|---|---|---|---|---|
Big Qdot | ~20 | SA | 10-2.5±0.02 | 390 ± 140 | 5 |
Small Qdot | ~10 | SA | 10-2.41±0.02 | 418 ± 172 | 75 |
GBP | 10-2.53±0.02 | 420 ± 170 | 82 (92) | ||
mSA | 10-2.59 ± 0.02 | 363 ± 144 | 88 (84) | ||
Atto647 | ~5 | SA | 10-2.55 ± 0.02 | 380 ± 156 | 77 (73) |
CF633 | 10-2.70 ± 0.01 | 380 ± 140 | 90 (93) |
-
Note: The first and second number for the synaptic receptors corresponds to the percentage of AMPAR in the lower left hand box and the area under the Gaussian curve for the trajectories, respectively, as outlined in each peaks of Figures 3–5. (The bQD data is for the 5% of all the AMPARs that are bound near a Homer1c, that is in the synapse. See discussion.)