Distinct SoxB1 networks are required for naïve and primed pluripotency
Abstract
Deletion of Sox2 from mouse embryonic stem cells (ESCs) causes trophectodermal differentiation. While this can be prevented by enforced expression of the related SOXB1 proteins, SOX1 or SOX3, the roles of SOXB1 proteins in epiblast stem cell (EpiSC) pluripotency are unknown. Here, we show that Sox2 can be deleted from EpiSCs with impunity. This is due to a shift in the balance of SoxB1 expression in EpiSCs, which have decreased Sox2 and increased Sox3 compared to ESCs. Consistent with functional redundancy, Sox3 can also be deleted from EpiSCs without eliminating self-renewal. However, deletion of both Sox2 and Sox3 prevents self-renewal. The overall SOXB1 levels in ESCs affect differentiation choices: neural differentiation of Sox2 heterozygous ESCs is compromised, while increased SOXB1 levels divert the ESC to EpiSC transition towards neural differentiation. Therefore, optimal SOXB1 levels are critical for each pluripotent state and for cell fate decisions during exit from naïve pluripotency.
https://doi.org/10.7554/eLife.27746.001Introduction
Pluripotent cells have the unique ability to differentiate into every cell type of an adult organism (Nichols and Smith, 2009). During mouse development, the embryo contains pluripotent cells in the epiblast until the onset of somitogenesis (Osorno et al., 2012; Chambers and Tomlinson, 2009). Distinct pluripotent cell types can be isolated in culture from the preimplantation and postimplantation epiblast. Embryonic stem cells (ESCs) (Evans and Kaufman, 1981; Martin, 1981) from preimplantation embryos are termed ‘naïve’ pluripotent cells. Epiblast stem cells (EpiSCs) (Tesar et al., 2007; Brons et al., 2007), commonly isolated from the post-implantation epiblast, are known as ‘primed’ pluripotent cells. Naïve and primed cells differ dramatically in responses to extracellular signals (Nichols and Smith, 2009). ESCs self-renew in response to a combination of leukaemia inhibitory factor (LIF) and either foetal calf serum (FCS), bone morphogenic protein (BMP) or Wnt (Smith et al., 1988; Ying et al., 2003a; ten Berge et al., 2011) and differentiate in response to fibroblast growth factor (FGF) (Kunath et al., 2007; Stavridis et al., 2007). In contrast, FGF together with Nodal/Activin A are required for EpiSCs self-renewal (Vallier et al., 2009; Guo et al., 2009).
Pluripotency is regulated by a pluripotency gene regulatory network (PGRN) (Chambers and Tomlinson, 2009; Festuccia et al., 2013; Wong et al., 2016). While some transcription factors such as Esrrb and T (Brachyury) are associated more closely with naïve or primed pluripotent states respectively (Festuccia et al., 2012; Osorno et al., 2012; Tsakiridis et al., 2014), the core TFs of the PGRN, Nanog, Sox2 and Oct4 (the Pou5f1 gene product, also referred as Oct3/4) are expressed in both naïve and primed pluripotent cells (Niwa et al., 2000; Masui et al., 2007; Avilion et al., 2003; Chambers et al., 2003; Karwacki-Neisius et al., 2013; Osorno et al., 2012; Festuccia et al., 2012; Brons et al., 2007; Tesar et al., 2007). While the role of Sox2 has been extensively characterised in naïve cells (Wong et al., 2016), its role in primed pluripotency is less well known.
Sox2 is a member of a family of twenty Sox TFs (Pevny and Lovell-Badge, 1997; Kamachi and Kondoh, 2013). All SOX proteins contain a High-Mobility-Group (HMG) box DNA-binding domain closely related to the founding member of the Sox family, SRY (Kondoh and Lovell-Badge, 2016). While some SOX proteins contain a transcriptional activation domain, others contain repression domains (Uchikawa et al., 1999; Bowles et al., 2000; Ambrosetti et al., 2000). The paradigm of action for SOX proteins is that they bind to target gene sequences through a DNA-mediated interaction with a partner protein, to specify target gene selection (Kamachi et al., 1999; Reményi et al., 2003; Williams et al., 2004; Kamachi and Kondoh, 2013). In pluripotent cells the principal interaction of SOX2 with OCT4 (Ambrosetti et al., 1997, 2000) is considered to positively regulate expression of many pluripotency-specific genes including Nanog, Oct4 and Sox2 (Tomioka et al., 2002; Chew et al., 2005; Okumura-Nakanishi et al., 2005; Rodda et al., 2005; Kuroda et al., 2005). Loss of SOX2 in ESCs induces trophoblast differentiation, phenocopying OCT4 loss and supporting the idea of a mutually dependent mode of action (Niwa et al., 2000; Masui et al., 2007).
Analysis of sequence conservation within the HMG box has divided the Sox family into eight groups that can be further divided into subgroups based on homology outside the HMG box (Kondoh and Lovell-Badge, 2015; Kamachi, 2016). SOX1, SOX2 and SOX3 belong to the SOXB1 group and also contain transcriptional activation domains (Uchikawa et al., 1999; Ambrosetti et al., 2000; Bowles et al., 2000; Kondoh and Kamachi, 2010; Ng et al., 2012; Kamachi and Kondoh, 2013). SOXB1 proteins bind the same DNA sequence in vitro (Kamachi et al., 1999; Kamachi, 2016). Previous studies demonstrated that SOXB1 factors are co-expressed during embryonic development and can substitute for each other in different biological systems, both in vitro and in vivo (Wood and Episkopou, 1999; Niwa et al., 2016; Adikusuma et al., 2017). Here, we investigate the requirements of naïve and primed pluripotent states for SOXB1 expression. Our results indicate that the essential requirement of SOXB1 function for naïve pluripotent cells extends to primed pluripotent cells. SOX3, which is highly expressed in primed pluripotent cells, functions redundantly with SOX2, rendering SOX2 dispensable in these cells. We further provide evidence that critical SOXB1 levels are required to specify the identity of cells exiting the naïve pluripotent state.
Results
A fluorescent reporter of SOX2 protein expression
To investigate the expression of Sox2 in pluripotent cells, a live cell reporter that retained Sox2 function was prepared by replacing the Sox2 stop codon with a T2A-H2B-tdTomato cassette (Figure 1A; Figure 1—figure supplement 1A). Correctly targeted cells were identified by Southern analysis and are referred to as E14Tg2a-Sox2-tdTomato (TST) cells (Figure 1—figure supplement 1B). Fluorescence microscopy of targeted cells showed a close correlation between SOX2 and tdTomato levels (Figure 1—figure supplement 2). Moreover, tdTomato expression recapitulated the SOX2 expression pattern in chimeric embryos (Figure 1—figure supplement 3). Targeted cells also showed the expected morphological differences when cultured in a combination of LIF plus inhibitors of MEK and GSK3β (LIF/2i), in LIF/FCS, in LIF/BMP or after passaging in Activin/FGF (Figure 1A). These results indicate that TST cells behave normally and provide a useful live cell report of Sox2 expression levels.
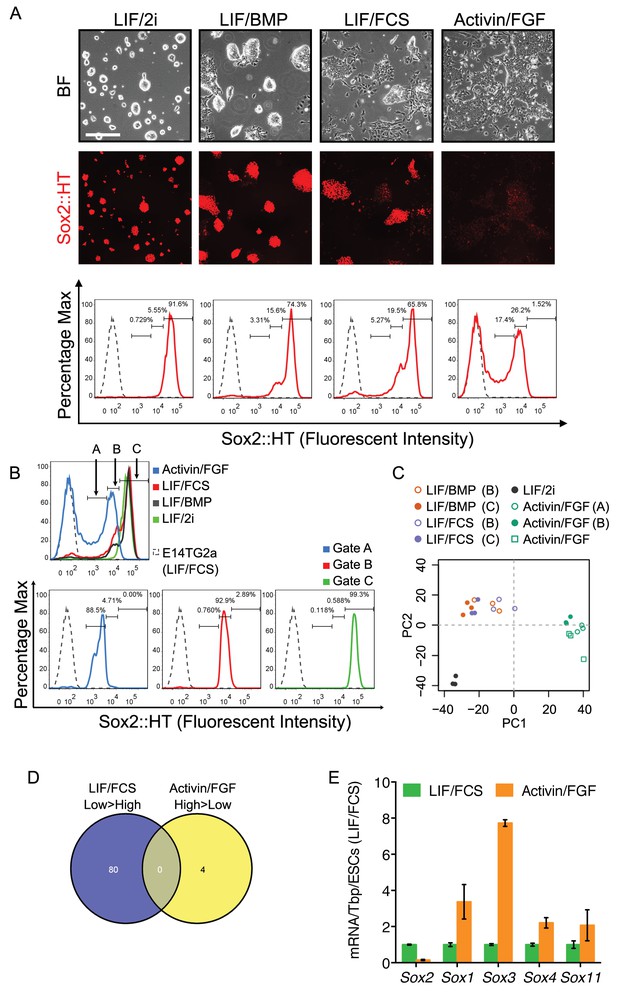
Different roles of Sox2 in preimplantation and postimplantation pluripotency.
(A) Expression of the Sox2-T2A-H2b-tdTomato (Sox2::HT) reporter from the endogenous Sox2 allele in targeted TST18 cells. TST18 cells cultured in LIF/FCS/GMEMβ were replated in LIF/2i/N2B27 or LIF/BMP4/N2B27 for four passages or in Activin/FGF/N2B27 (Activin/FGF) for nine passages, examined microscopically (top) and assessed by flow cytometry (bottom); E14Tg2a cells were represented as a grey dashed line. (B) Three gates (A, B, C) were used to purify cells for microarray analysis. Gate C captured the Sox2::HT level in LIF/2i cultured ESCs. Gate B captured the overlapping Sox2::HT level in LIF/FCS, LIF/BMP and Activin/FGF cultured cells. Gate A captured the lower Sox2::HT level in Activin/FGF. (C) Principal component analysis of cells in different culture conditions, either unsorted or sorted using the gates indicated by brackets. (D) Despite similar Sox2::HT levels, no differentially expressed genes (DEGs, FDR = 0.1) were common to LIF/FCS-low and Activin/FGF-high cell populations. (E) RT-qPCR analysis of the indicated transcripts in ESCs (LIF/FCS, red bars) and EpiSCs (Activin/FGF, cultured for 16 passages, blue bars). Transcript levels were normalized to Tbp and plotted relative to ESCs. Error bars represent standard error of the mean (n = 3 to 4).
TST ESCs were next assessed by fluorescence microscopy and FACS. In LIF/2i, tdTomato expression was high and unimodal (Figure 1A). In LIF/FCS or LIF/BMP the same predominant high-expressing population was present but with a shoulder of reduced expression and a small number of tdTomato-negative cells, which appeared to coincide with morphologically differentiated cells (Figure 1A). In continuous culture in Activin/FGF, tdTomato expression was bimodal with the highest expression levels overlapping the lower expression levels seen in ESCs cultured in LIF/FCS or LIF/BMP (Figure 1A,B).
Gene expression in ESCs and EpiSCs expressing distinct Sox2 levels
ESCs and EpiSCs expressing distinct Sox2 levels were separated by FACS according to the tdTomato level (Figure 1B). Microarray analysis was then used to compare gene expression in cells from different culture conditions expressing similar Sox2 levels and in cells from the same cultures expressing distinct Sox2 levels (Supplementary file 1). Cells expressing the highest tdTomato levels in LIF/2i, LIF/FCS or LIF/BMP cultures were purified using gate C. The mid-level expression gate B enabled purification of the Sox2-low fraction of ESCs cultured in LIF/FCS or LIF/BMP as well as the highest Sox2-expressing EpiSCs. EpiSCs were also purified using the lower expression gate A. Re-sorting confirmed effective purification prior to RNA extraction and analysis (Figure 1B). Compared to the Sox2-high population, Sox2-low EpiSCs had upregulated differentiation markers. Transcripts expressed by Sox2-low EpiSCs differed from those expressed by Sox2-low ESCs (Figure 1—figure supplement 4; Supplementary file 1) suggesting that ESCs and EpiSCs have distinct differentiation propensities. ESCs from both LIF/BMP and LIF/FCS cultures sorted for highest tdTomato expression (gate C) were enriched for naïve pluripotency transcripts with mRNAs common to both (including Nr5a2, Tbx3 and Tcl1) positively correlating to Sox2 in all samples (Figure 1—figure supplement 5). Moreover, principal component analysis indicated that these Sox2-high ESCs cluster together and separately from ESCs cultured in LIF/2i (Figure 1C) as seen by others (Boroviak et al., 2015). More importantly, principal component analysis also showed that EpiSCs and ESCs expressing the same Sox2 level (gate B) were transcriptionally distinct (Figure 1C) and shared no commonly enriched mRNAs (Figure 1D). Thus, Sox2 levels alone do not dictate the distinction between ESC and EpiSC states.
Microarray and quantitative transcript analyses highlighted changes in Sox gene expression levels (Figure 1E, Figure 1—figure supplement 6). Although other Sox gene expression changes occurred, the ability of SOXB1 proteins to function redundantly in ESC self-renewal (Niwa et al., 2016) prompted us to assess the capacity of SOXB1-related proteins to function more widely in pluripotent cells.
A subset of SOX family proteins can functionally replace Sox2 in ESC self-renewal
Recently, Niwa et al. (2016) reported that ESC self-renewal could be maintained in the absence of SOX2 by expression of other SOXB1 and SOXG proteins (Niwa et al., 2016). We addressed the question of functional redundancy with Sox2 in ESCs using an independent Sox2 conditional knockout (SCKO) ESC line (Favaro et al., 2009; Gagliardi et al., 2013). SCKO ESCs have one Sox2 allele replaced by β-geo, the second allele flanked by loxP sites and constitutively express a tamoxifen-inducible Cre recombinase (CreERT2) (Figure 2A). SCKO ESCs were transfected with a plasmid in which constitutive Sox cDNA expression was linked to hygromycin B resistance and were either left untreated or were simultaneously treated with tamoxifen to excise Sox2 (Figure 2A). ESC self-renewal was assessed after 8–10 days of hygromycin selection. Transfection of plasmids encoding transcriptional activator proteins of the SOXB1 family (SOX1, SOX2 or SOX3) enabled similar levels of ESC colony formation in the absence of Sox2 (Figure 2B,C). In contrast, the SOXB2 proteins (SOX14 and SOX21), in which the SOXB DNA-binding domain is linked to transcriptional repression domains, did not direct undifferentiated ESC colony formation (Figure 2C). Of the other SOX proteins tested, only SOX15 showed any capacity to direct undifferentiated ESC colony formation (Figure 2C), as previously described (Niwa et al., 2016), possibly because it has the most similar DNA-binding domain to SOXB proteins (Kamachi and Kondoh, 2013). These results indicate that ESC self-renewal requires the function of a SOX protein with a SOXB-like DNA-binding domain coupled to a transcriptional activating function.
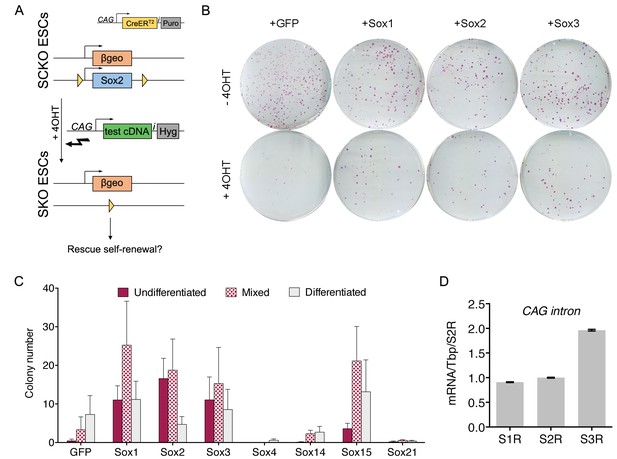
The ESC function of Sox2 can be substituted by SOXB1 and SOXG proteins.
(A) Strategy for testing the ability of candidate Sox cDNAs to rescue ESC self-renewal upon Sox2 deletion. Sox2fl/- (SCKO) ESCs were treated with 4-hydroxy-tamoxifen (4OHT) to induce nuclear localisation of CreERT2 and consequent loxP-mediated Sox2 excision. Simultaneous transfection of test cDNAs linked to hygromycin phosphotransferase via an IRES (i) were tested for Sox2 complementation activity. (B) SCKO ESCs transfected with the indicated cDNAs were cultured in the presence of hygromycin B and in the absence (−4OHT) or presence (+4 OHT) of 4-hydroxyl-tamoxifen for 8 days before being fixed and stained for alkaline phosphatase (AP) activity. (C) Stained colonies were scored based on AP+ (undifferentiated), mixed or AP− (differentiated) morphology. Error bars represent standard error mean (n = 3). (D) RT-qPCR analysis of the rescuing transgenes in Sox1-, Sox2- and Sox3-rescued (S1R, S2R, S3R) ESC populations grown in the presence of 4-hydroxyl-tamoxifen as described in Figure 2A,B. RT-qPCR was performed using common primers designed across the CAG intron upstream of the rescuing transgene. Values were normalised over Tbp and expressed relative to S2R cells. Error bars represent the standard error of the mean (n = 3).
To further assess the ability of SOX proteins to sustain ESC self-renewal, we attempted to expand transfected cell populations. PCR analysis confirmed deletion of the Sox2 conditional allele (400 bp) from expanded cell populations expressing SOX1, SOX2 or SOX3 (Figure 2—figure supplement 1). Furthermore, SoxB1-rescued (S1R and S3R) SKO ESCs retained OCT4 and NANOG expression, similar to SCKO and Sox2-rescued SKO (S2R) ESCs (Figure 2—figure supplement 2). The transgene expression levels in ESC populations rescued by Sox1, 2 or 3 were assessed by quantitative transcript analysis using common primers across the CAG intron. This demonstrated that the Sox3 transgene mRNA was expressed at a higher level than the Sox1 or Sox2 transgenes (Figure 2D). These results confirm the findings of Niwa et al. in an independent cell line (Niwa et al., 2016).
Sox3 is dispensable for both naïve and primed pluripotency
Sox3 transcripts are present in ESCs at lower levels than Sox2 (Figure 3—figure supplement 1) and a previous report has suggested that Sox3 is dispensable for ESC self-renewal (Rizzoti et al., 2004; Rizzoti and Lovell-Badge, 2007). To directly assess this possibility, and as a first step to determining whether Sox3 is required in EpiSCs, CRISPR/Cas9 was used to delete the Sox3 gene from male E14Tg2a ESCs (Hooper et al., 1987; Doetschman et al., 1987) using two small guide RNAs (sgRNA1 and sgRNA2) (Figure 3A). After targeting, E14Tg2a ESCs were plated at low density and single clones were isolated. PCR genotyping using primers flanking the predicted Cas9 cut sites (Figure 3A) identified two clones (S3KO8 and S3KO35) in which Sox3 had been deleted (Figure 3B). Replating at clonal density indicated that both clones retained an efficient self-renewal ability (Figure 3C). Moreover, both clones had unchanged levels of Nanog, Oct4 and Sox2 mRNAs (Figure 3D). These findings indicate that Sox3 is dispensable for ESC self-renewal, confirming previously unpublished data (Rizzoti et al., 2004).
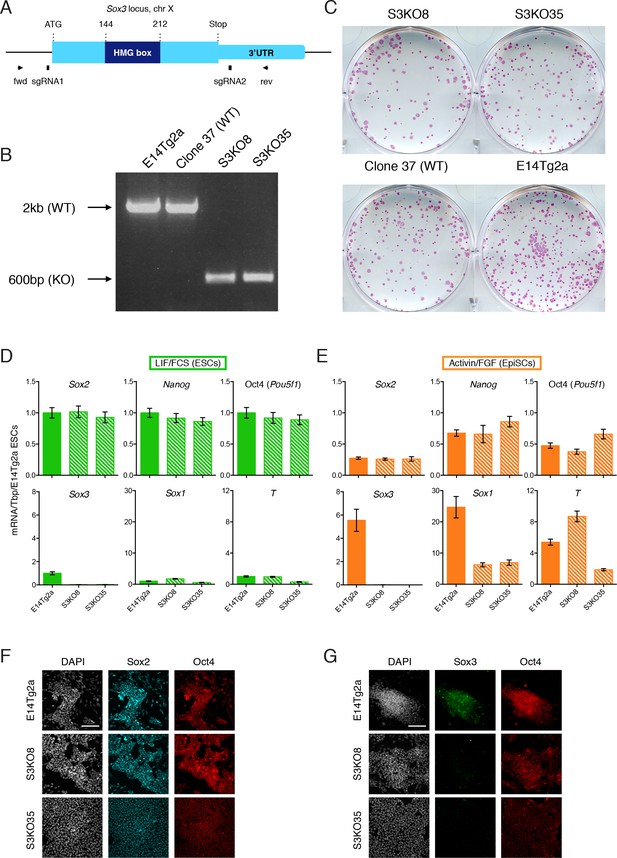
Sox3 is dispensable for pluripotent cell maintenance.
(A) Schematic representation of the Sox3 locus on the mouse X chromosome showing the CRISPR/Cas9 strategy for the generation and genotyping of Sox3 knockout ESCs. The positions of sgRNA1 and sgRNA2 used for the deletion of the Sox3 locus are indicated alongside the positions of PCR primers used for genotyping. (B) PCR genotyping of parental ESCs (E14Tg2a), a wild-type clone (37) and two Sox3 knockout clones (S3KO8, S3KO35) after CRISPR/Cas9 targeting of the Sox3 locus. Band sizes for the WT (~2 kb) and targeted (~600 bp) alleles are shown. (C) Alkaline phosphatase staining in S3KO8, S3KO35 and WT (Clone 37 and E14Tg2a) ESCs grown at clonal density for 7 days. (D–E) RT-qPCR analysis of the indicated transcripts in wild-type E14Tg2a and Sox3-/Y (S3KO8, S3KO35) ESCs (D) and EpiSCs (E) derived by serial passaging (>10 passages) in Activin/FGF conditions. Error bars represent standard error of the mean (n = 3). (F-G) Immunofluorescence staining of SOX2 (F), SOX3 (G) and OCT4 proteins in E14Tg2a and Sox3-/Y EpiSCs (S3KO8, S3KO35). Scale bar, 100 μm.
Next, the requirement of SOX3 for primed pluripotency was determined by examining the ability of the above Sox3 knockout ESC clones, together with parental E14Tg2a ESCs, to be converted to EpiSCs by serial passaging in Activin and FGF (Guo et al., 2009). Quantitative transcript analysis at passage 12 indicated similar levels of Sox2, Oct4 and Nanog mRNA expression in wild-type and Sox3 knockout EpiSCs (Figure 3E). In contrast, Sox1 mRNA levels were reduced in both Sox3 knockout clones (Figure 3E) and T (Brachyury) was more variably expressed (Figure 3E). The ability of EpiSCs to self-renew in the absence of SOX3 was maintained over 25 passages without affecting SOX2 and OCT4 protein expression or EpiSC morphology (Figure 3F,G). These data demonstrate that both naïve and primed pluripotent cells can self-renew in the absence of Sox3.
Sox2 is dispensable for the maintenance of primed pluripotency
To investigate whether primed pluripotency can be maintained in the absence of SOX2, SCKO EpiSCs were derived by in vitro culture of SCKO ESCs (Guo et al., 2009). SCKO EpiSCs were then transfected with a plasmid encoding both Cre recombinase and tdTomato (Figure 4A), since the CAG CreERT2 transgene (Figure 2A) had been silenced during the EpiSC transition. After 12–24 hr, tdTomato-positive EpiSCs were sorted by FACS and re-plated at low cell density to allow expansion of single clones. PCR genotyping of expanded EpiSC clones revealed that the loxP-flanked Sox2 allele had been excised generating Sox2-/- (SKO) clones (Figure 4A). Immunoblot analysis confirmed that these SKO clones did not express SOX2 protein and that Sox2fl/- EpiSCs expressed SOX2 at 50% the level of Sox2+/+ EpiSCs (Figure 4B). Moreover, NANOG protein levels were similar to parental SCKO EpiSCs or wild-type E14Tg2a EpiSCs (Figure 4B). Sox2-/- EpiSCs retained an undifferentiated morphology (Figure 4C) and immunostaining confirmed the absence of SOX2 and continued OCT4 expression compared to parental SCKO EpiSCs (Figure 4D). Quantitative transcript analysis of Oct4, Nanog and Sox2 , indicated that, while Sox2 mRNA was absent from Sox2-/- EpiSCs, both Oct4 and Nanog mRNA levels were unaffected (Figure 4E). This suggests that the core pluripotency gene regulatory network remains active even in the absence of SOX2 and that, in contrast to the situation with naïve pluripotency, primed pluripotency is not critically dependent upon Sox2.
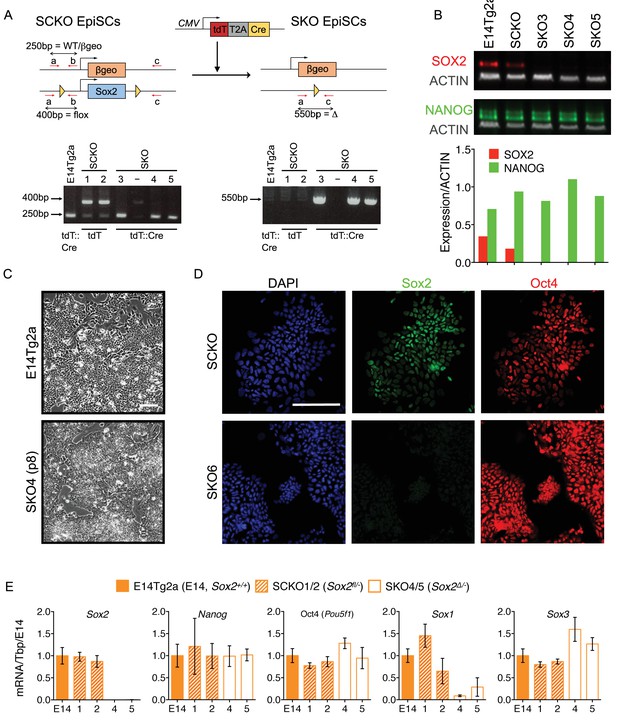
Sox2 is dispensable for the maintenance of EpiSCs.
(A) Strategy for Sox2 deletion in EpiSCs. Sox2fl/- (SCKO) EpiSCs were transfected with pCMV-tdTomato-T2A-Cre. After 12–24 hours, cells were sorted for tdTomato expression and re-plated in the presence of ROCK inhibitor. Clones were picked and expanded before genotyping by PCR as indicated in Figure 2—figure supplement 1 . SCKO EpiSCs were also transfected with pCMV-tdTomato (lacking Cre). Expanded clones were numbered as indicated. (B) Immunoblot analysis of E14Tg2a, Sox2fl/- (SCKO) and Sox2-/- (SKO) EpiSCs showing that SOX2 protein (red) is reduced in SCKO and absent in SKO EpiSCs. NANOG protein levels (green) were unaffected. Protein levels (normalized to the βactin level) are graphed below. (C) Bright-field morphology of E14Tg2a and SKO4 EpiSCs after 2 weeks (8 passages) in culture. Scale bar, 100 μm. (D) Immunofluorescence staining of SOX2 and OCT4 in SCKO and SKO6 EpiSCs. Scale bar, 100 μm. (E) RT-qPCR analysis of the indicated transcripts in E14Tg2a, Sox2fl/- (SCKO) and Sox2-/- (SKO) EpiSCs. Clone numbers are indicated. Error bars represent the standard error of the mean (n = 2 to 3).
The ability of SOXB1 proteins to substitute for one another in ESCs raised the hypothesis that functional redundancy between SOX2 and SOX3 may be responsible for the maintenance of pluripotency in primed EpiSCs. Expression of both Sox1 and Sox3 mRNAs was increased in EpiSCs compared to ESCs (Figure 1E). Examination of Sox2-/- EpiSCs showed that while Sox1 mRNA expression was reduced, Sox3 mRNA levels were elevated compared to control cells (Figure 4E). This suggests that Sox3 expression might functionally compensate for the lack of Sox2 in Sox2-/- EpiSCs, as is the case in Sox2-null E14.5 forebrain, where a 2-fold increase in Sox3 mRNA was detected (Miyagi et al., 2008). Alternatively, SOXB1 proteins may be irrelevant for EpiSC self-renewal. To distinguish between these possibilities we developed an approach to gene disruption using CRISPR/Cas9.
Testing the functional effects of Sox ORF disruption using CRISPR/Cas9
To examine the functional dependence of cells on Sox genes, a CRISPR/Cas9 approach was developed and tested initially using Sox2. Insertions or deletions (indels) into the Sox2 open reading frame (ORF) were introduced immediately upstream of the sequence encoding the SOX2 HMG domain (Figure 5—figure supplement 1A). Out-of-frame indels in this position are expected to abolish the DNA-binding ability of any aberrant protein that might still be produced. The frequency and length of indels induced by CRISPR/Cas9-mediated targeting of the endogenous Sox2 locus were investigated by TIDE (Tracking of Indels by Decomposition) analysis (Brinkman et al., 2014). Sox2fl/- SCKO ESCs that constitutively express Sox1, Sox3 or GFP (generated in Figure 2B in the absence of tamoxifen) were analysed. If, as expected, Sox1 or Sox3 can rescue self-renewal, then such cells would carry both in-frame and out-of-frame (deleterious) indels (Figure 5—figure supplement 1B). In contrast, if ESC self-renewal relies on the remaining Sox2 allele, as anticipated in the case of SCKO ESCs constitutively expressing GFP, then cells carrying deleterious indels should be eliminated from the population. In this case, the only modifications present would be non-deleterious in-frame indels (Figure 5—figure supplement 1B). SCKO ESCs expressing Sox1, Sox3 or GFP were transfected with a plasmid encoding the sgRNA and eCas9. Cells were selected, genomic DNA isolated, PCR amplified, sequenced and analysed by TIDE. The population of SCKO ESCs expressing GFP contained Sox2 loci with no out-of-frame indels detected (Figure 5—figure supplement 2). In contrast, SCKO ESCs constitutively expressing Sox1 or Sox3 tolerated out-of-frame, deleterious indels at Sox2 (size +1,–10, −13) (Figure 5—figure supplement 2). These results show that indel analysis can be applied to study functional redundancy between SOXB1 group members in the maintenance of pluripotent cells.
Sox2 and Sox3 are functionally redundant for the maintenance of EpiSCs
Having established the utility of CRISPR/Cas9-mediated indel induction for gene function analysis, a similar strategy was applied to Sox2-/- EpiSCs to determine whether SOXB1 proteins operate in a functionally redundant way to maintain EpiSCs. Since Sox2-/- EpiSCs showed an increase in Sox3 mRNA expression upon deletion of the second Sox2 allele, we focussed on Sox3, which is X-linked and thus present in only one copy in these male EpiSCs (Sox3+/Y). Two sgRNAs (sgRNA1 and 2) were designed to independently target the Sox3 ORF immediately upstream of the sequence encoding the HMG DNA-binding domain and tested individually (Figure 5A). TIDE analysis (Figure 5B) showed that in control EpiSCs expressing endogenous Sox2 (E14Tg2a and SCKO) both in frame and out of frame indels within Sox3 were detected using either sgRNA (Figure 5C). In contrast, two independent Sox2-/- EpiSC lines (SKO1, SKO6) retained only in frame deletions that did not disrupt the SOX3 HMG box (Figure 5C). Together with earlier results on Sox2-/- EpiSCs (Figure 4) and Sox3-/Y EpiSCs (Figure 3), these data indicate that EpiSCs lacking either Sox2 or Sox3 alone can be maintained, while cells lacking both SOX2 and SOX3 proteins are lost. Therefore, EpiSC self-renewal requires SOXB1 function, and this can be provided by the expression of either endogenous Sox2 or Sox3.
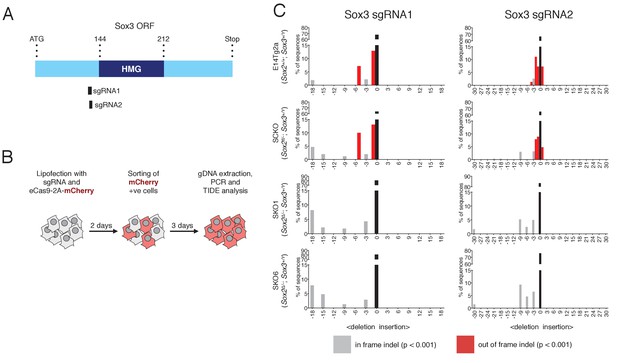
Sox3 indel analysis in Sox2-/- EpiSCs.
(A) Schematic representation of the Sox3 ORF with the HMG box highlighted in dark blue. The amino-acid positions of the HMG box relative to the start (ATG) codon of the ORF are shown. The positions of the sgRNAs 1 and 2 used are represented as black bars. (B) Experimental design for Sox3 indel analysis in Sox2-/- EpiSCs. (C) Indel analysis performed using the TIDE tool (https://tide-calculator.nki.nl/) in two Sox2-/- EpiSC lines (SKO1 and SKO6), in the parental Sox2fl/- (SCKO) EpiSCs and in control E14Tg2a EpiSCs using Sox3 sgRNAs 1 and 2. Histograms represent indel frequency and size. Black bars indicate the frequency of unmodified (WT) alleles; grey bars indicate significant in frame indels and red bars indicate significant out of frame indels (p<0.001). Non-significant (n/s, p≥0.001) indels are not shown.
Modulating SOXB1 levels affects differentiation and can prevent capture of primed pluripotency
Since Sox2 and Sox3 transcript levels change during ESC to EpiSC differentiation, this raised the question of their importance for attainment of a primed pluripotent state. ESCs that delete Sox2 differentiate to trophectoderm (Masui et al., 2007), while ESCs that continue expressing high SOX2 protein levels during differentiation are biased towards neural fates (Zhao et al., 2004). To assess the effect of increasing the SOXB1 concentration upon differentiation, we examined three clones overexpressing either SOX2 (Figure 6) or SOX3 (Figure 6—figure supplement 1), generated using the approach outlined in Figure 2A. When placed in an EpiSC differentiation protocol, both SOX2- and SOX3-overexpressing clones showed reduced expression of Oct4, Nanog and Nr5a2 transcripts as well as increased expression of Sox1, Mash1 (the Ascl1 gene product) and Pax6 transcripts (Figure 6A; Figure 6—figure supplement 1). Examination of SOX2-overexpressing clones in Activin/FGF showed neural-like cellular morphology (Figure 6B) and βIII-tubulin-positive axonal processes (Figure 6C). These findings indicate that while high SOXB1 levels are tolerated by ESCs, a decreased dosage of SOXB1 is essential for ESCs to transit effectively to an EpiSC state and avoid ectopic neural differentiation.
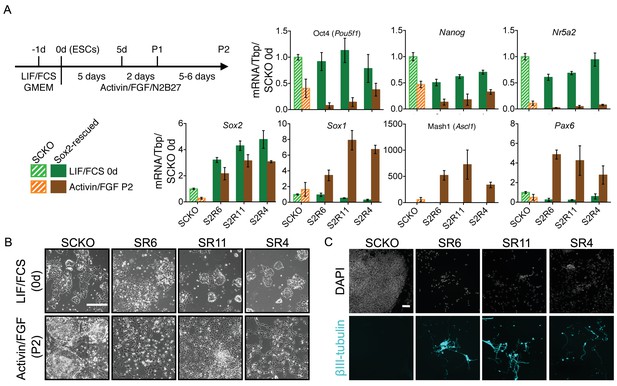
Increased SOXB1 levels skew ESC differentiation towards the neural lineage.
(A) Schematic diagram showing the experimental plan. RT-qPCR analysis of the indicated transcripts in SCKO cells stably transfected and rescued with a Sox2 transgene (12–24 hr prior to a 24 hr treatment with 4OHT) (termed Sox2-rescued, S2R cells). Cells were grown in LIF/FCS conditions or differentiated in Activin/FGF conditions for 13 days (P2). Transcript levels were normalized to Tbp and plotted relative to SCKO ESCs. Error bars represent standard error of the mean (n = 3 to 5). (B) Bright field images of the indicated cells maintained in LIF/FCS or differentiated in Activin/FGF conditions (P2) . Scale bar, 100 μm. (C) Immunofluorescence staining of the neural marker βIII-tubulin (cyan) in cells differentiated in Activin/FGF conditions (P2); DAPI represented in grey. Scale bar, 100 μm.
The SoxB1 requirements during neural differentiation of ESCs were next assessed. Initially, we compared Sox2+/+ ESCs previously cultured in LIF/FCS or LIF/2i during neural differentiation induced by culture in N2B27 medium (Ying and Smith, 2003; Ying et al., 2003a). Our results indicate a more rapid induction of a Sox1-GFP reporter (Aubert et al., 2003) and Sox1 mRNA from LIF/FCS cultures than from LIF/2i cultures (Figure 7A). The Sox1-GFP kinetics from 2i/LIF cultures observed here agree with those reported (Marks et al., 2012), although this study noted a slower induction of Sox1-GFP from FCS/LIF cultures. However, as the timing of Sox1-GFP induction from FCS/LIF cultures in previous studies from the same group (Ying et al., 2003a) was consistent with the timings reported here, further differentiation experiments were initiated from LIF/FCS. Expression of pluripotency markers Nanog and Nr5a2 was decreased in the neural differentiation protocol, although with lower efficiency in Sox2fl/- ESCs than in control Sox2+/+ cells, while Fgf5 was induced in both (Figure 7B). Strikingly, Sox1, Mash1 and Pax6 mRNAs were induced in Sox2+/+ but not Sox2fl/- cells (Figure 7B), and an increase in Sox3 was not sustained. These data indicate that a SOXB1 level above that present in Sox2fl/- cells is required to enable ESCs to undergo neural differentiation in vitro. Furthermore, the expression of Fgf5 suggests that Sox2fl/- cells attain some aspects of an early post-implantation identity (Figure 7B). This contrasts with the neural differentiation observed in Sox2+/- mouse embryos (Avilion et al., 2003; Rizzoti and Lovell-Badge, 2007; Favaro et al., 2009), suggesting that compensatory mechanisms other than SOXB1 redundancy exist in the embryo.
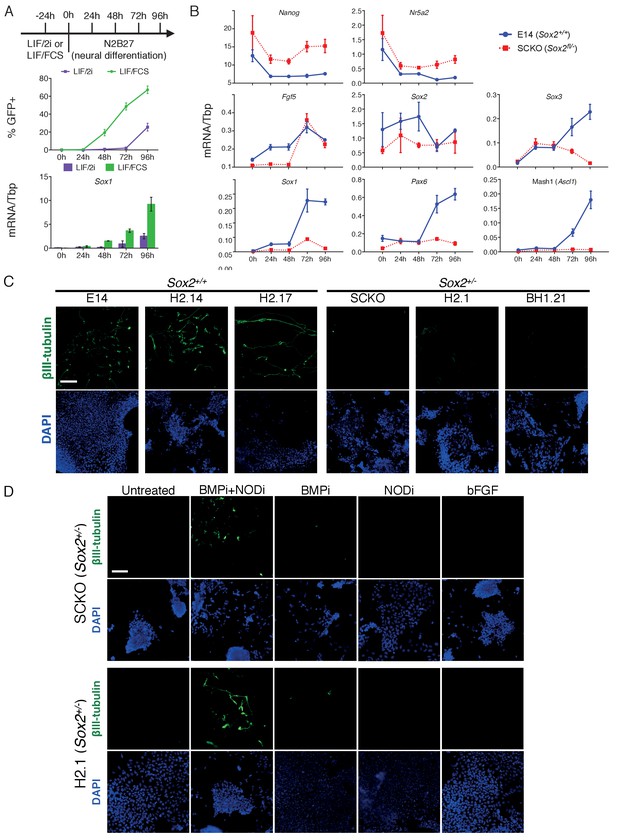
Decreased SOX2 levels prevent ESC differentiation into neurons.
(A) (TOP) Schematic diagram showing experimental plan for neural differentiation. (MIDDLE) Sox1-GFP (Aubert et al., 2003) expression in 46C cells cultured in LIF/FCS (green) or LIF/2i (purple) and for the indicated number of hours in N2B27 neural differentiation medium were assessed by flow cytometry. The positive (+) gate was set above the GFP expression level observed in 46C ESCs. The error bars indicate the standard error of the mean (n = 4). (BOTTOM) RT-qPCR analysis of Sox1 mRNA level in 46C cells during neural differentiation. Error bars indicate the standard error of the mean (n = 2). (B) RT-qPCR analysis of the indicated transcripts in differentiating E14Tg2a (E14, Sox2+/+) and SCKO (Sox2fl/−) cells. Transcript levels were normalized to Tbp. Error bars represent standard error of the mean (n = 3). (C) Immunofluorescence staining of the neural marker βIII-tubulin (green) in Sox2+/+ E14Tg2a (E14), H2.14 and H2.17 ESCs, and in Sox2+/- SCKO, H2.1 and BH1.21 ESCs differentiated in N2B27 medium for 4 days; DAPI represented in blue. Scale bar, 100 μm. (D) Immunofluorescence staining of the neural marker βIII-tubulin (green) in Sox2+/-and H2.1 ESCs differentiated in N2B27 medium for 4 days in the presence or in the absence of the LDN-193189 BMP inhibitor (BMPi), of the SB-431542 Nodal inhibitor (NODi) and of recombinant bFGF; DAPI represented in blue. Scale bar, 100 μm.
To eliminate the possibility that the neural differentiation defect in SCKO cells resulted from a defect unrelated to Sox2, CRISPR/Cas9 was used to introduce indels in the Sox2 ORF in E14Tg2a ESCs. Using independent sgRNAs, two Sox2+/- clones were isolated. Clone H2.1 carried an 8 bp deletion on one Sox2 allele; clone BH1.21 carried a 10 bp deletion on one allele and a 3 bp deletion on the other allele (Figure 7—figure supplement 1A). Two additional clones in which the Sox2 alleles were not modified (H2.14, H2.17) were used as controls. The −8 and −10 deletions cause frame-shifts introducing stop codons, while the −3 bp deletion is likely to be functionally neutral as it occurs N-terminal to the HMG domain (Figure 7—figure supplement 1B). Placing Sox2+/+ ESCs (H2.14, H2.17 and E14Tg2a) in a neural differentiation protocol produced βIII-tubulin positive neurons (Figure 7C). In contrast, no βIII-tubulin positive cells were detected from parallel treatments of H2.1, BH1.21 and SCKO Sox2+/- (Figure 7C). This establishes that the lack of a functional Sox2 allele impairs effective neural differentiation of ESCs.
Neural differentiation of pluripotent cells is stimulated by FGF (Ying et al., 2003a; Ying and Smith, 2003) and inhibited by both BMP and Nodal/Activin (Ying et al., 2003b; Vallier et al., 2004; 2009; Guo et al., 2009). To determine whether perturbations in these pathways could overcome the neural differentiation defect of Sox2+/- ESCs, H2.1 and SCKO cells were placed in the neural differentiation protocol supplemented with either recombinant bFGF or inhibitors of BMP or Nodal. Additional FGF, or inhibition of Nodal alone, was without effect, while BMP inhibition resulted in only a few βIII-tubulin-positive cells (Figure 7D). However, simultaneous BMP and Nodal inhibition enabled Sox2+/- ESCs to form βIII-tubulin-positive cells (Figure 7D). These results indicate that a reduction in SOX2 levels in ESCs enhances the response of cells to endogenous BMP and Nodal signalling, preventing effective neural differentiation.
To further investigate the effects of modulating SoxB1 gene dosage upon ESC differentiation, Sox3-/Y ESCs were generated by CRISPR/Cas9 mediated gene deletion from heterozygous Sox2fl/- ESCs (SCKO). PCR genotyping identified two Sox2fl/-; Sox3-/Y ESC clones (#36 and #37) (Figure 8A). Quantitative transcript analysis showed that while Sox2fl/- ESCs had an expected reduction in Sox2 mRNA and unchanged levels of Sox1 and Sox3 mRNAs compared to E14Tg2a ESCs, deletion of Sox3 from Sox2fl/- ESCs increased Sox1 and surprisingly, also Sox2 mRNA to a level similar to that present in E14Tg2a ESCs (Figure 8B). These data suggest cross-regulatory interactions between SoxB1 members in which SOX3 protein represses Sox1 and Sox2, either directly or indirectly, in ESCs (Figure 8B). However, as Sox3 deletion in Sox2+/+ ESCs did not increase Sox1 and Sox2 mRNA levels (Figure 3D) this suggests that the repressive effect of SOX3 on Sox2 is sensitive to the SOX2 protein concentration.
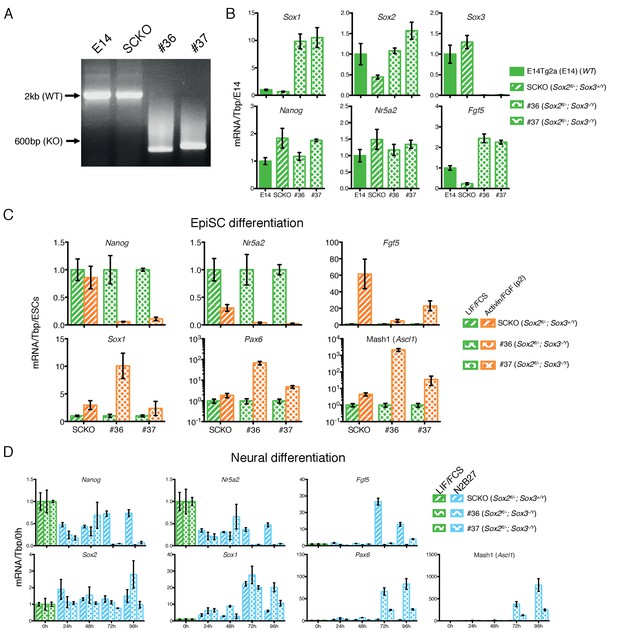
Sox2/Sox3 requirements during EpiSCs differentiation.
(A) Genotyping analysis of the Sox3 locus in E14Tg2a (E14, Sox2+/+), SCKO (Sox2fl/-) ESCs (∼2 kb band) and in two Sox2fl/-; Sox3-/Y ESC clones (#36 and #37) derived after deletion of the Sox3 locus (∼600 bp band). Gene targeting was performed following the strategy depicted in Figure 3A. (B) RT-qPCR analysis of the indicated transcripts in E14Tg2a ESCs (E14, Sox2+/+), SCKO ESCs (Sox2fl/-) and in two Sox2fl/-; Sox3-/Y ESC clones (#36 and #37). mRNA levels were normalised over Tbp and plotted relative to E14Tg2a. Error bars indicate the standard error of the mean (n = 3). (C) RT-qPCR analysis of the indicated transcripts in SCKO cells (Sox2fl/-) and in two Sox2fl/-; Sox3-/Y; Sox2fl/- clones (#36 and #37) grown in LIF/FCS condition and in Activin/FGF conditions for 2 passages (P2). mRNA levels were normalised to Tbp and plotted relative to ESCs (LIF/FCS). Error bars indicate the standard error of the mean (n = 3). (D) RT-qPCR analysis of the indicated transcripts in SCKO cells (Sox2fl/-) and in two Sox2fl/-; Sox3-/Y clones (#36 and #37) grown in LIF/FCS condition and in neural differentiation medium (N2B27) for the indicated number of hours. mRNA levels were normalised to Tbp and plotted relative to ESC (LIF/FCS). Error bars indicate the standard error of the mean (n = 3).
Next, the ability of Sox2fl/-; Sox3-/Y ESCs to transition to primed pluripotency was assessed by passaging in Activin/FGF. Whereas Sox2fl/- EpiSCs could be successfully established and maintained (Figure 4), Sox2fl/-; Sox3-/Y cells displayed a differentiated morphology within two passages. Quantitative transcript analysis showed that in comparison to Sox2fl/- cells, Sox2fl/-; Sox3-/Y cells induced less Fgf5 but more Mash1 and Pax6 (Figure 8C). These data suggest that while primed pluripotency can be maintained in the absence of either Sox2 or Sox3, the transition from a naïve ESC state to a primed EpiSC state does not occur effectively when Sox3 is absent and the Sox2 gene dosage is halved. Notably, compared to Sox2fl/- ESCs, Sox2fl/-; Sox3-/Y ESCs have the same Sox2 mRNA level as Sox2+/+ ESCs and Sox1 mRNA is increased >10 fold (Figure 8B). Such an increase in Sox1 mRNA expression is sufficient to enforce neural differentiation following LIF withdrawal (Zhao et al., 2004).
The increased levels of Sox1 and Sox2 mRNAs in LIF/FCS (Figure 8B), together with the increased levels of neural differentiation markers during EpiSC induction of Sox2fl/-; Sox3-/Y ESCs (Figure 8C), prompted us to examine the behaviour of Sox2fl/-; Sox3-/Y ESCs during neural differentiation. While Sox2+/- ESCs did not effectively undergo neural differentiation (Figure 7), deletion of Sox3 from Sox2fl/- ESCs was sufficient to rescue neural differentiation as judged by induction of Sox1, Mash1 and Pax6 mRNAs and of the βIII-tubulin protein (Figure 8D, Figure 8—figure supplement 1). This is likely a secondary consequence of the increase in Sox2/Sox1 mRNA expression in Sox2fl/- ESCs resulting from Sox3 deletion (Figure 8B). The two Sox2+/+; Sox3-/Y ESC clones (Figure 3A) were able to differentiate into neural cells with similar efficiency to parental cells as shown by induction of Sox1, Mash1 and Pax6 mRNAs (Figure 8—figure supplement 2) and appearance of βIII-tubulin-positive cells (Figure 8—figure supplement 1).
These data demonstrate that altering the SoxB1 genetic composition by deletion of Sox3 and elimination of one functional Sox2 allele induces a transcriptional de-regulation of the remaining SoxB1 alleles. The increased mRNA levels of Sox1 and Sox2 are then sufficient to rescue neural differentiation of Sox2fl/- ESCs but also impair the ability of Sox2fl/-; Sox3-/Y ESCs to be captured as primed EpiSCs.
Discussion
Our examination of SoxB1 function in pluripotent cells extends previous findings that SOXB1 proteins can act redundantly in ESCs (Niwa et al., 2016) and during somatic cell reprogramming (Nakagawa et al., 2008) by showing that in EpiSCs, SOX2 and SOX3 proteins are functionally redundant within an altered PGRN. Functional redundancy implies that we can consider the total SOXB1 complement as a key factor in the outcomes selected by cells genetically engineered to express varying combinations of functional SoxB1 alleles. In particular, a broad range of SOXB1 levels are tolerated by ESCs. However, upon exit from naïve pluripotency, low SOXB1 levels permit entry to the EpiSC state, while high levels enforce neural differentiation (Figure 9). We have also uncovered cross-regulatory relationships between Sox3 and the other SoxB1 members, that we hypothesise act to maintain an adequate SOXB1 level.
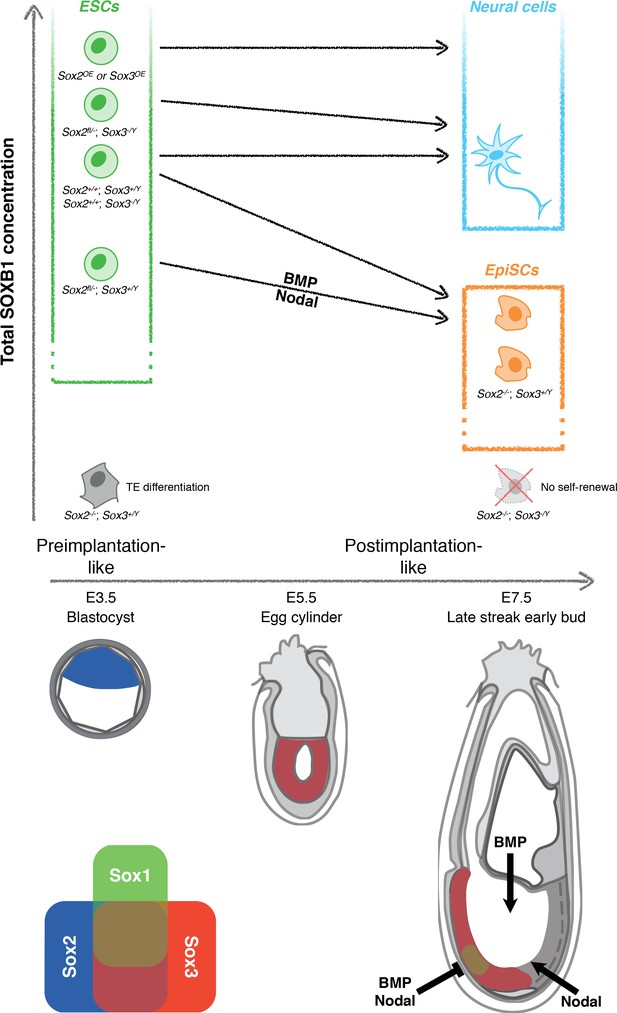
Dependence of cell fate potential of ESCs on the total SOXB1 concentration.
The overall SOXB1 concentration inferred from SoxB1 transcript levels in different SoxB1 mutant cell lines. Naïve ESCs self-renew in a wide range of SOXB1 concentrations. However, only ESCs with approximately wild-type SOXB1 levels can differentiate towards both primed EpiSCs and neural cells. ESCs with increased SOXB1 concentrations are poised towards neural differentiation, preventing their capture as primed EpiSCs in FGF/ActivinA. Decreased SOXB1 concentrations are insufficient to enable neural differentiation due to increased activity of neural antagonists (BMP and Nodal). A further reduction in SOXB1 is tolerated in the primed state due to SOX2/SOX3 functional redundancy but complete loss of the predominant SoxB1 forms is incompatible with self-renewal of both naïve and primed pluripotent cells. Depicted below are diagrams of pre- (E3.5) and postimplantation (E5.5 and E7.5) mouse embryos indicating the published expression patterns of SoxB1 mRNAs (Wood and Episkopou, 1999; Uchikawa et al., 2011; Avilion et al., 2003; Cajal et al., 2012), and the areas of BMP/Nodal signalling and inhibition during gastrulation (Constam and Robertson, 2000; Bachiller et al., 2000; Kinder et al., 2001; Levine et al., 2006; Pereira et al., 2012; Norris et al., 2002; Lawson et al., 1999; Perea-Gomez et al., 2002).
SOXB1 function in primed pluripotent cells is provided by SOX2 and SOX3
In ESCs Sox2 mRNA is expressed at much higher levels than either Sox1 or Sox3, and thus, in spite of redundancy, Sox2 can be considered to provide the dominant SOXB1 function in ESC pluripotency. However, even though Sox2 is also the most abundant SoxB1 transcript in EpiSCs, functional properties of the PGRN have changed. While Sox2 mRNA levels in EpiSCs are lower than in ESCs, Sox3 levels are increased, and SoxB1 function is provided redundantly by Sox2 and Sox3. Sox1 mRNA levels are also increased in these cells. The ability of Sox1 or Sox3 to substitute functionally for Sox2 in ESCs might suggest that Sox1 could also function redundantly with Sox2 to maintain EpiSCs. However, Sox1 may be less relevant to pluripotency as, unlike Sox2 and Sox3, which are widely expressed in the pluripotent postimplantation epiblast, Sox1 expression is uniquely associated with neural fate in the epiblast (Wood and Episkopou, 1999; Uchikawa et al., 2011; Cajal et al., 2012; Figure 9). Indeed, Sox1 expression in EpiSC populations is associated with neural committed cells (Tsakiridis et al., 2014).
SOXB1 redundancy in vivo
In the seminal Sox2 deletion study (Avilion et al., 2003), Sox2-/- embryos fail to develop a postimplantation epiblast. This was hypothesised to be due to the fact that neither Sox1 nor Sox3 were expressed sufficiently at the time of embryonic failure and therefore no redundantly acting SOXB1 protein could compensate for the SOX2 absence. Additional instances of potential SoxB1 redundancy have been reported in vivo. Replacement of an endogenous Sox2 allele with a Sox1 ORF produced no phenotype, suggestive of functional interchangeability (Ekonomou et al., 2005). SOXB1 redundancy is likely to be evolutionarily conserved since in the chick, Sox2 and Sox3 both promote development of ectoderm and neurectoderm at gastrulation, although interestingly, in this case Sox3 expression occurs before Sox2 (Acloque et al., 2011). Moreover, while genetic knock-ins have shown that placement of Sox2 ORF at the Sox3 locus can rescue pituitary and testes phenotypes caused by Sox3 deletion (Adikusuma et al., 2017), the effects on pluripotent cells were not assessed. It is therefore interesting that while Sox3-/Y mice can be viable, on a 129 genetic background they exhibit gastrulation-stage lethality (Rizzoti and Lovell-Badge, 2007; Adikusuma et al., 2017). This suggests that aspects of the regulation of SoxB1 expression that we show here to be important in vitro could also contribute to strain-specific differences in the timing or regulation of SOXB1 activity in vivo. Further studies will be required to establish the extent to which SOXB1 proteins can substitute genetically for one another in pluripotent cells in vivo.
Low SOXB1 levels permit entry to the EpiSC state
Our results indicate that the total SoxB1 transcript level in ESCs needs to be reduced to enable entry into the EpiSC state rather than neural differentiation (Figure 9). Sox2+/- ESCs placed in a neural differentiation protocol showed a reduced down-regulation of pluripotency markers (Nanog and Nr5a2) and failed to induce markers of neural differentiation. Surprisingly however, deletion of Sox3 from SCKO cells to produce Sox2fl/-; Sox3-/Y ESCs restored neural differentiation capacity (Figure 8D). Moreover, placement of Sox2fl/-; Sox3-/Y ESCs in an EpiSC differentiation protocol resulted in a skewing of differentiation towards a neural identity as indicated by loss of Nanog, Nr5a2 and Fgf5 transcripts, and increase in mRNAs for neural differentiation markers (Figure 8C). This paradoxical behaviour may be explained by the fact that both Sox1 and Sox2 mRNAs increase upon elimination of Sox3 from Sox2fl/- ESCs (Figure 8B). Interestingly, reciprocal repression of Sox3 by SOX2 also occurs in EpiSCs (Figure 4E). Unravelling the regulatory relationships between SoxB1 genes is a relevant point for future studies.
High SOXB1 levels enforce neural differentiation
Previous results have shown that elevating SOX1 or SOX2 expression in differentiating ESCs promotes neural differentiation (Zhao et al., 2004). In the present study, we showed that ESCs with either elevated SOX2 or SOX3 self-renew efficiently. However, when these cells were placed in an EpiSC differentiation protocol, differentiation was skewed towards a neural identity despite the presence of FGF and neural-antagonising Activin signals (Vallier et al., 2004, 2009). This indicates that enforced expression of SOXB1 proteins overrides the signalling system that captures primed pluripotent cells in vitro.
Parallels between SOXB1 function during in vitro and in vivo neurogenesis
The dynamics of ESC differentiation in vitro towards neural and EpiSC states show interesting parallels with early development in vivo (Figure 9). While ESCs and pre-implantation embryos express only Sox2, early post-implantation embryos, and differentiating ESCs, express Sox2 and Sox3 but not Sox1 (Wood and Episkopou, 1999; Uchikawa et al., 2011; Cajal et al., 2012). At gastrulation stages, which are transcriptomically similar to EpiSCs (Kojima et al., 2014), Sox2 and Sox3 are expressed widely in the epiblast. As noted above, Sox1 expression is restricted to a subdomain within the Sox2/Sox3-positive epiblast that overlaps extensively with the prospective brain (Wood and Episkopou, 1999; Cajal et al., 2012), where early neural differentiation occurs in vivo. Thus, the region expressing all three SOXB1 members might undergo neural induction as a consequence of expressing high levels of SOXB1 proteins, while the rest of the epiblast experiences lower SOXB1 levels, enabling pluripotency to extend through gastrulation (Osorno et al., 2012).
An interplay between SOXB1 function and anti-neural signals
Inhibition of both BMP and Nodal signalling rescues the neural differentiation ability of Sox2+/- ESCs. These results suggest that Sox2+/- ESCs can initiate exit from naïve pluripotency but cannot complete neural differentiation due to enhanced responses of cells to endogenous anti-neuralising signalling by BMP and Nodal. The viability of Sox2+/- mice (Avilion et al., 2003; Rizzoti and Lovell-Badge, 2007; Favaro et al., 2009) indicates that the in vivo environment is able to overcome these anti-neural signals. In wild-type embryos, the prospective brain is shielded from Nodal and BMP signalling by secreted inhibitors of these pathways, including Cer1, Lefty1/2 (Perea-Gomez et al., 2002), Chrd and Nog (Bachiller et al., 2000) (Figure 9). Removal of either Nodal or BMP inhibition leads to absence of anterior neural tissue. Interestingly, the cells that express these inhibitors (including the anterior visceral endoderm and node) do not express SOXB1 proteins and are therefore likely to be functionally unaltered in Sox2+/- embryos. The observation that the reduced SOXB1 concentration in Sox2+/- pluripotent cells is compatible with neural differentiation, provided that endogenous anti-neuralising signals are blocked, indicates that to fully understand how the choice between neural and primed pluripotency is made, it will be necessary to elucidate how the signalling environment connects to the SOXB1-driven transcriptional programme.
Materials and methods
Cell culture
Request a detailed protocolFor a complete list of cell lines, their name, their genotypes and their original characterisation, see Supplementary file 2. All the cell lines used in this study were regularly tested for contaminations and were mycoplasma negative.
ESCs grown in LIF/FCS conditions were cultured on dishes coated with 1% gelatin (Sigma-Aldrich, St. Louis, USA) and in GMEM medium (Sigma-Aldrich, St. Louis, USA) supplemented with 1x non-essential aminoacids (Life Technologies, Waltham, USA), 1 mM sodium pyruvate (Life Technologies, Waltham, USA), 2 mM glutamine (Life Technologies, Waltham, USA), 100 U/ml human LIF (Nichols et al., 1990), 10% ESC-grade FCS (APS, UK) and 100 μM β-mercaptoethanol (Life Technologies, Waltham, USA). G418 (200 μg/mL, Sigma-Aldrich, St. Louis, USA) was supplemented to maintain SCKO ESCs.
To adapt ESCs into LIF/2i/N2B27 (Ying et al., 2008) (LIF/2i) or LIF/BMP4/N2B27 (Ying et al., 2003b) (LIF/BMP) conditions, ESCs were replated in LIF/FCS on gelatin-coated plates for 24 hr before changing the culture media to N2B27 medium (Ying and Smith, 2003) supplemented with 100 U/ml LIF, 1 μM PD0325901 (Stemgent, Cambridge, USA) and 3 μM CHIR99021 (Stemgent, Cambridge, USA) (LIF/2i) or 10 ng/ml BMP4 (Life Technologies, Waltham, USA) (LIF/BMP). Cells were passaged for at least four passages before using for analysis.
EpiSCs were derived from LIF/FCS-cultured ESCs as described previously (Guo et al., 2009; Osorno et al., 2012) and cultured on dishes pre-coated with 7.5 μg/ml fibronectin (Sigma-Aldrich, St. Louis, USA) without feeders in N2B27 medium (Ying and Smith, 2003) ActivinA (20 ng/ml, Peprotech, UK), bFGF (10 ng/ml, Peprotech, UK). In brief, ESCs were plated at a density of 3 × 103 cells/cm2; the equivalent of 30 × 103 cells per well of a six well plate. Medium was changed to Activin/FGF conditions 24 hr after replating. Cells were passaged after 4–5 days in a 1:20 dilution for the first 8–10 passages. Stable EpiSC lines were propagated by passaging in a 1:10-1:20 dilution.
For neural differentiation, ESCs were cultured with minor modifications as previously described (Ying et al., 2003a; Ying and Smith, 2003). Briefly, ESCs were replated in LIF/FCS on gelatin-coated plates for 24 hr before changing the culture media to N2B27 medium (Ying and Smith, 2003) only and allowed to grow for the indicated amount of time. When indicated, cells were differentiated in the presence of bFGF (10 ng/ml, Peprotech, UK), LDN-193189 (100 nM, Stemgent, Cambridge, USA) and/or SB-431542 (10 μM, Merck, Germany).
Sox2 deletion in ESCs and EpiSCs
Request a detailed protocolSox2 deletion in SCKO ESCs was performed similarly to previously described (Gagliardi et al., 2013; Favaro et al., 2009). In brief, 107 SCKO ESCs grown in LIF/FCS conditions were transfected using Lipofectamine 3000 (Life Technologies, Waltham, USA) with 6–15 μg of transgene-expressing plasmid the indicated test cDNA before replated in LIF/FCS at a density of 1.5 × 106 per 10 cm dish cultured overnight in the presence or in the absence of 4-hydroxytamoxifen (1 μM, Sigma-Aldrich, St. Louis, USA). Medium was changed 12 to 24 hr later to LIF/FCS medium supplemented with Hygromycin B (150 μg/mL, Roche, Switzerland). Transfected cells were cultured for 8 to 10 days before they were stained for alkaline phosphatase (AP) activity (Sigma-Aldrich, St. Louis, USA). Stained colonies were scored based on the presence of AP-positive cells within the colony. In parallel, unstained populations were expanded to generate cell lines before genotyping by PCR.
SCKO EpiSCs were transfected with ptdTomato-T2A-Cre by lipofection (Lipofectamine 2000, Life Technologies, Waltham, USA). After 12–24 hours cells were sorted for tdTomato expression and replated in the presence of ROCK inhibitor (Y-27632, Merck, Germany) for 24 hr before medium was changed to remove ROCK inhibitor. Clones were expanded before genotyping by PCR.
CRISPR/Cas9 deletion of the Sox3 gene
Request a detailed protocolTwo sgRNAs were designed upstream of the ATG (sgRNA1) and downstream of the stop codon (sgRNA2) using an online CRISPR Design Tool (http://crispr.mit.edu/) and subsequently cloned in the BbsI-linearised pSpCas9(BB)−2A-GFP plasmid (Addgene 48138) as previously described (Ran et al., 2013). sgRNA sequences used in this study are listed in Supplementary file 3. 106 E14Tg2a ESCs were co-transfected with 1 μg of sgRNA1-encoding plasmid and 1 μg of sgRNA2-encoding plasmid using Lipofectamine 3000 (Life Technologies, Waltham, USA) following the manufacturer’s instructions. 24 hr after transfection, GFP-positive cells were sorted by FACS and replated at clonal density to allow the isolation of single colonies. After 8–10 days, single ESC colonies were expanded and the Sox3 locus was genotyped by PCR.
Indel induction and TIDE analysis
Request a detailed protocolSgRNAs targeting the Sox2 or Sox3 ORF immediately upstream of the sequence encoding for the HMG box were designed using an online CRISPR Design Tool (http://crispr.mit.edu/). The Sox2 sgRNA was subsequently cloned in the BbsI-linearised pSpCas9(BB)−2A-Puro (PX459) V2.0 plasmid (Addgene 62988) as previously described (Ran et al., 2013). The resulting plasmid, or the empty vector control, were then transfected using Lipofectamine 3000 (Life Technologies, Waltham, USA) into SCKO ESCs constitutively expressing either SOX1, SOX3 or GFP. After 24 hr, ESCs were selected with 1.5 μg/ml of puromycin (Life Technologies, Waltham, USA) for 24 hr to enrich for transfected cells, and then expanded for 72 hr. For Sox3 indel analysis, two individual sgRNAs were cloned in BbsI-linearised pSpCas9(BB)−2A-mCherry that was obtained by fusing a 2A-mCherry cassette to the Cas9 CDS of the eSpCas9(1.1) plasmid (Addgene 71814). 106 E14Tg2a (Sox2+/+), SCKO (Sox2fl/-), SKO1 (Sox2-/-) and SKO6 (Sox2-/-) EpiSCs were then transfected with 1 μg of sgRNA-containing plasmids or empty vector using Lipofectamine 3000 (Life Technologies, Waltham, USA). 48 hr after transfection, mCherry-positive cells were FACS sorted, replated and expanded for 72 hr. Genomic DNA (gDNA) was extracted using the DNeasy Blood and Tissue kit (Qiagen, Germany) following the manufacturer’s instructions. gDNA was PCR amplified using the Q5 HotStart Polymerase (NEB, Ipswich, USA) and primers flanking either the Sox2 sgRNA or Sox3 sgRNAs recognition sites. Primers and sgRNAs used in this study are listed in Supplementary file 3. PCR amplicons were purified and submitted for Sanger sequencing using the same forward primer that they had been generated with. Sanger sequencing electropherograms were then submitted for indel analysis with the TIDE tool (https://tide-calculator.nki.nl/, [Brinkman et al., 2014]) using amplicons obtained from cells transfected with empty vector as reference sequences. Indel analysis was performed using the default TIDE settings and a window of 27 bp for SCKO ESCs and Sox2 indel induction, and a window of 30 bp for EpiSCs and Sox3 indel induction. Indels with p value >0.001 were scored as statistically significant.
PCR genotyping
Request a detailed protocolGenomic DNA (gDNA) was extracted from cells using DNeasy kits (Qiagen, Germany) according to the manufacturer’s recommended protocol. 100 ng of gDNA was used per PCR reaction. Primers used in this study are listed in Supplementary file 3.
Plasmid constructs
Request a detailed protocolA mouse genomic BAC containing 5 kb on either side of the Sox2 stop codon (Source bioscience bMQ314D22) was shredded by pSC101-BAD-gbaA mediated recombineering using the pACYC177 backbone comprising the p15-origin and β-lactamase gene to produce pSox2-10kb. To construct the Sox2-T2A-H2B-tdTomato-IRES-Neo cassette, 89 bp and 168 bp immediately upstream and downstream of, and excluding the Sox2 stop codon were PCR amplified to serve as homology arms for recombineering. The 89 bp upstream fragment has a 5’ Xho I site and a GSG-T2A sequence at the 3’ end containing an in-frame Fse I site that preserves the T2A Gly-Pro residues and is followed by Not I, Pac I, Asc I, San DI, Bam HI, Afl II, Nhe I, Cla I and Xho I sites. The sequence of this fusion is shown below:
GGCTCCGGAGAGGGCAGAGGAAGTCTGCTAACATGCGGTGACGTCGAGGAGAATCCTGGGCCGGCCGCGGCCGCTTAATTAAGGCGCGCCGGGACCCGGATCCGCTTAAGGCTAGCATCGATTCTCGAG
The 89 bp fragment was cloned into a PCR amplified, pUC19-derived 2 kb minimal vector comprising β-lactamase gene and Col E1 origin with 150nt flanking sequences and a single Xho I site (pL). Individual features were PCR-amplified, flanked with unique sites and cloned as follows: H2B-tdTomato was cloned in-frame between Fse I and Not I, using TAA stop codon from Pac I; Gtx-IRES was cloned between Pac I and Asc I; neomycin phosphotransferase (Npt) was cloned between San DI and Bam HI, using the TAA stop codon from Afl II. The 168 bp downstream fragment is flanked with Nhe I at the 5’ side and Cla I at the 3’ end and was cloned between Nhe I and Cla I (pL-Sx-5HTiN3).
To insert a selection cassette for use in recombineering and targeting, a linker was made by annealing the following two oligonucleotides:
TCGAGCTTAAGGTCGACAGATCTCGATCGGCTAGCC
TCGAGGCTAGCCGATCGAGATCTGTCGACCTTAAGC
The linker was cloned into the Xho I site of a pTOPO-BluntII-derived, Zeocin-resistant version of pL (pZ-Linker). A 3.6 kb Bam HI fragment containing PGK-EM7-Npt-pA and MC1-HSVtk-pA flanked by FRT sites (FNF) was subcloned from pBS-M179 (a kind gift from Dr. Andrew Smith) into the Bgl II site of pZ-Linker (pZ-FNF), destroying the Bam HI and Bgl II sites. This places an Afl II site on one side and Nhe I site on the other side of the FNF cassette and these are used to subclone into pL-Sx-5HTiN3 to make pL-Sx-5HTiNFNF3.
To make the targeting vector, a 7.1 kb Xho I-Cla I fragment from pL-Sx-5HTiNFNF3 was transfected into E. coli containing pSox2-10kb and pSC101-BAD-gbaA to replace the Sox2 stop codon by recombineering. Successful kanamycin-resistant recombinants carrying a 20 kb targeting vector (pSox2AHTiN-FNF-10kb) were amplified at 37°C to restrict pSC101-BAD-gbaA replication and identified by diagnostic Bam HI digest.
The targeting construct was linearised, electroporated into E14Tg2a ESCs and G418 resistant colonies expanded and genotyped by southern blot analysis as described below. The FRT-flanked cassette was removed by transiently transfecting pPGK-FlpO into verified E14TG2a-derived Sox2-tdTomato ESC clone 18 (TST18).
Overexpression constructs were generated by cloning open reading frames (ORFs) of indicated Sox genes, preceeded upstream by the Kozak consensus sequence (GCCGCCACC), into pPyCAG-IRES-Hyg vector between the XhoI and NotI sites (Chambers et al., 2003).
Southern blot analysis
Request a detailed protocol40 µg of genomic DNA were digested with Eco RI (5’ probe analysis) or with Hind III (3’ and internal probe analysis) and assessed by Southern blot analysis using probes synthesised by PCR from the oligonucleotides indicated in Supplementary file 3.
RNA level quantification
Request a detailed protocolTotal RNA was purified from cells using RNeasy mini kits (Qiagen, Germany) following the manufacturer’s protocol. cDNA was prepared from 1 μg of total RNA using SuperScript III reverse transcription kits (Life Technologies, Waltham, USA) according to the recommended protocol and the final cDNA solution was diluted 1:10 prior to use. 2 μl of cDNA solution was used per reaction with the Takyon Sybr Assay (Eurogentec, Belgium). Primers used in this study are listed in Supplementary file 3.
Microarray gene expression analysis
Request a detailed protocolTotal RNA (127 ng/sample) from three independently replicated experiments was converted into biotin-labelled cRNA using the Illumina TotalPrep RNA amplification kit (Ambion, Cambridge, USA). Microarray hybridization reactions were performed on a Mouse WG-6v2 BeadChip (Illumina). Raw data were normalised in R using the beadarray (Dunning et al., 2007), limma (Smyth, 2005) and sva (Leek and Storey, 2007) packages from the Bioconductor suite (Gentleman et al., 2004). Briefly, low-quality probes were removed from the input and data were then quantile-normalized. ComBat was used to account for batch effects (Leek and Storey, 2007) between microarrays run at different dates. Differential expression in the log2-transformed data was assessed with the limma algorithms (Smyth, 2005). Probes were considered differentially expressed if they showed a FDR-adjusted p-value of ≤ 0.1 and an absolute log2 fold change ≥ log2(1.5). Primary analysis results were uploaded to GeneProf (Halbritter et al., 2011) and mapped to Ensembl-based reference genes, collapsing multiple probes for the same gene by picking the most responsive probe (i.e., the probe with the highest absolute fold change across all pair-wise comparisons). Data are available in Supplementary file 1. Microarray data have been submitted to the Gene Expression Omnibus (GEO) under accession code GSE99185.
Immunofluorescence staining
Request a detailed protocolCells were fixed with 4% PFA (10 mins, RT) and permeabilised using PBS/0.1% (v/v) Triton-X100 (PBSTr) for 10 mins, before quenching with 0.5M Glycine/PBSTr (15 mins). Non-specific antigens were blocked using 3% (v/v) donkey serum/1% (v/v) BSA/PBSTr (1 hr, RT) before incubating with primary antibody in blocking solution (4°C, overnight). Cells were washed with PBSTr before incubating with donkey-raised secondary antibodies conjugated with Alexa-488,–568 or −647 in blocking solution (1–2 hr, RT). DAPI (1–2 μg/mL, Molecular Probes) in PBS was added to cells for at least 30 mins before imaged using the Olympus IX51 inverted fluorescent microscope. Primary antibodies used were: α-SOX2 (1:400; Abcam ab92494, UK ), α-NANOG (1:500; eBioscience 14–5761, Cambridge, USA) and α-OCT4 (1:400; Santa Cruz Biotechnology sc8628, Santa Cruz, USA), α-SOX3 antibody (1:400; Abcam ab42471, UK ), α-βIII-tubulin (1:1500; Covance MMS-435P, Princeton, USA ).
Immunoblotting analysis
Request a detailed protocolCells were lysed with lysis buffer comprising 50 mM Tris pH 8.0, 150 mM NaCl supplemented with fresh 0.5% NP-40, 0.5 mM DTT, 1 × protease inhibitors cocktail (Roche, Switzerland) and 1.3 μl of Benzonase (Novagen, Germany) (1 hr, 4°C). Samples were prepared by boiling 40 μg of total protein extract with Laemmli buffer (Life Technologies, Cambridge, USA) . Samples were analysed using Bolt 10% Bis-Tris +SDS PAGE (Life Technologies, Cambridge, USA) and electroblotted onto 0.2 µm pore Whatman-Protran nitrocellulose membranes (Capitol Scientific, Austin, USA) in transfer buffer comprising 25 mM Tris/0.21M glycine/20% methanol. Membranes were blocked using 5% (w/v) low-fat milk in 0.01% (v/v) Tween-20/PBS (PBSTw) before incubating with primary antibody in blocking solution . Membranes were washed with PBSTw before incubating with donkey-raised secondary antibodies conjugated with IRDye 800CW (1:10000; LI-COR 926–32213, Lincoln, USA) and HRP-conjugated α-βActin (1:10000; Abcam ab20272, UK) antibody. HRP-staining was developed using a Super-signal West Pico kit (Pierce, Cambridge, USA) before imaging the membranes using LI-COR Odyssey Fc imager. The primary antibodies used were: α-Sox2 (1:1000; Abcam ab92494, UK) and α-Nanog (1:2000; Bethyl Laboratories A300-397A, Montgomery, USA).
Embryo manipulation
Request a detailed protocolMice were maintained on a 12 hr light/dark cycle. All animals were maintained and treated in accordance with guidance from the UK Home Office. Embryonic day (E)0.5 was designated as noon on the day of finding a vaginal plug. Morula aggregations, blastocyst injections and embryo transfer were performed using standard procedures. Chimeric, gastrulation-stage embryos were collected at E7.5 and imaged using an inverted fluorescence microscopy. Chimeric E9.5 embryos were fixed with 4% PFA at 4°C for 3 hr and cryosectioned as described before (Wymeersch et al., 2016). Sections were stained for GFP as described above.
Data availability
-
Distinct SoxB1 networks are required for naïve and primed pluripotencyPublicly available at the NCBI Gene Expression Omnibus (accession no: GSE99185).
References
-
Multipotent cell lineages in early mouse development depend on SOX2 functionGenes & Development 17:126–140.https://doi.org/10.1101/gad.224503
-
Easy quantitative assessment of genome editing by sequence trace decompositionNucleic Acids Research 42:e168.https://doi.org/10.1093/nar/gku936
-
The transcriptional foundation of pluripotencyDevelopment 136:2311–2322.https://doi.org/10.1242/dev.024398
-
Reciprocal transcriptional regulation of Pou5f1 and Sox2 via the Oct4/Sox2 complex in embryonic stem cellsMolecular and Cellular Biology 25:6031–6046.https://doi.org/10.1128/MCB.25.14.6031-6046.2005
-
Tissue-specific requirements for the proprotein convertase furin/SPC1 during embryonic turning and heart loopingDevelopment 127:245–254.
-
beadarray: R classes and methods for Illumina bead-based dataBioinformatics 23:2183–2184.https://doi.org/10.1093/bioinformatics/btm311
-
Hippocampal development and neural stem cell maintenance require Sox2-dependent regulation of ShhNature Neuroscience 12:1248–1256.https://doi.org/10.1038/nn.2397
-
The role of pluripotency gene regulatory network components in mediating transitions between pluripotent cell statesCurrent Opinion in Genetics & Development 23:504–511.https://doi.org/10.1016/j.gde.2013.06.003
-
Sox289–106, Chapter 6 – Evolution of Sox2 and Functional Redundancy in Relation to Other SoxB1 Genes, Sox2.
-
The organizer of the mouse gastrula is composed of a dynamic population of progenitor cells for the axial mesodermDevelopment 128:3623–3634.
-
SOX-partner code for cell specification: Regulatory target selection and underlying molecular mechanismsThe International Journal of Biochemistry & Cell Biology 42:391–399.https://doi.org/10.1016/j.biocel.2009.09.003
-
Octamer and Sox elements are required for transcriptional cis regulation of Nanog gene expressionMolecular and Cellular Biology 25:2475–2485.https://doi.org/10.1128/MCB.25.6.2475-2485.2005
-
Bmp4 is required for the generation of primordial germ cells in the mouse embryoGenes & Development 13:424–436.https://doi.org/10.1101/gad.13.4.424
-
Generation of induced pluripotent stem cells without Myc from mouse and human fibroblastsNature Biotechnology 26:101–106.https://doi.org/10.1038/nbt1374
-
Deciphering the Sox-Oct partner code by quantitative cooperativity measurementsNucleic Acids Research 40:4933–4941.https://doi.org/10.1093/nar/gks153
-
Establishment of germ-line-competent embryonic stem (ES) cells using differentiation inhibiting activityDevelopment 110:1341–1348.
-
Naive and primed pluripotent statesCell Stem Cell 4:487–492.https://doi.org/10.1016/j.stem.2009.05.015
-
The Foxh1-dependent autoregulatory enhancer controls the level of Nodal signals in the mouse embryoDevelopment 129:3455–3468.
-
Oct-3/4 and Sox2 regulate Oct-3/4 gene in embryonic stem cellsThe Journal of Biological Chemistry 280:5307–5317.https://doi.org/10.1074/jbc.M410015200
-
Sox genes find their feetCurrent Opinion in Genetics & Development 7:338–344.https://doi.org/10.1016/S0959-437X(97)80147-5
-
Genome engineering using the CRISPR-Cas9 systemNature Protocols 8:2281–2308.https://doi.org/10.1038/nprot.2013.143
-
SOX3 is required during the formation of the hypothalamo-pituitary axisNature Genetics 36:247–255.https://doi.org/10.1038/ng1309
-
Transcriptional regulation of nanog by OCT4 and SOX2Journal of Biological Chemistry 280:24731–24737.https://doi.org/10.1074/jbc.M502573200
-
Bioinformatics and Computational Biology Solutions Using R and Bioconductor397–420, limma: Linear Models for Microarray Data, Bioinformatics and Computational Biology Solutions Using R and Bioconductor, New York, Springer-Verlag.
-
Embryonic stem cells require Wnt proteins to prevent differentiation to epiblast stem cellsNature Cell Biology 13:1070–1075.https://doi.org/10.1038/ncb2314
-
Identification of Sox-2 regulatory region which is under the control of Oct-3/4-Sox-2 complexNucleic Acids Research 30:3202–3213.https://doi.org/10.1093/nar/gkf435
-
B1 and B2 Sox gene expression during neural plate development in chicken and mouse embryos: universal versus species-dependent featuresDevelopment, Growth & Differentiation 53:761–771.https://doi.org/10.1111/j.1440-169X.2011.01286.x
-
Sox2163–185, Chapter 10 – SOX2-Dependent Regulation of Pluripotent Stem Cells, Sox2.
-
Defined conditions for neural commitment and differentiationMethods in Enzymology 365:327–341.https://doi.org/10.1016/S0076-6879(03)65023-8
-
Conversion of embryonic stem cells into neuroectodermal precursors in adherent monocultureNature Biotechnology 21:183–186.https://doi.org/10.1038/nbt780
-
SoxB transcription factors specify neuroectodermal lineage choice in ES cellsMolecular and Cellular Neuroscience 27:332–342.https://doi.org/10.1016/j.mcn.2004.08.002
Article and author information
Author details
Funding
Medical Research Council
- Andrea Corsinotti
- Frederick CK Wong
- Florian Halbritter
- Douglas Colby
- Nicholas P Mullin
- Valerie Wilson
- Ian Chambers
Biotechnology and Biological Sciences Research Council
- Elisa Hall-Ponsele
- Ian Chambers
Biotechnology and Biological Sciences Research Council (Japan partnering award)
- Ian Chambers
The funders had no role in study design, data collection and interpretation, or the decision to submit the work for publication.
Acknowledgements
We thank Emily Erickson and Christine Watson (Cambridge) for suggesting the use of TIDE analysis; CRM Animal House, Microscopy Facility and FACS Facility staff for assistance; Donal O’Carroll for comments on the manuscript. This research is supported by grants from the Medical Research Council (IC and VW) and the Biotechnological and Biological Sciences Research Council of the United Kingdom (IC) and by a Japan Partnering Award from the Biotechnological and Biological Sciences Research Council (IC). FCKW was supported by an MRC studentship and a Centenary Award; EM received an award from the International Association for the Exchange of Students for Technical Experience.
Ethics
Animal experimentation: Animal experiments were performed under the UK Home Office project license PPL60/4435, approved by the Animal Welfare and Ethical Review Panel of the MRC Centre for Regenerative Medicine and within the conditions of the Animals (Scientific Procedures) Act 1986.
Copyright
© 2017, Corsinotti et al.
This article is distributed under the terms of the Creative Commons Attribution License, which permits unrestricted use and redistribution provided that the original author and source are credited.
Metrics
-
- 1,953
- views
-
- 307
- downloads
-
- 24
- citations
Views, downloads and citations are aggregated across all versions of this paper published by eLife.
Citations by DOI
-
- 24
- citations for umbrella DOI https://doi.org/10.7554/eLife.27746