Detection of human disease conditions by single-cell morpho-rheological phenotyping of blood
Figures
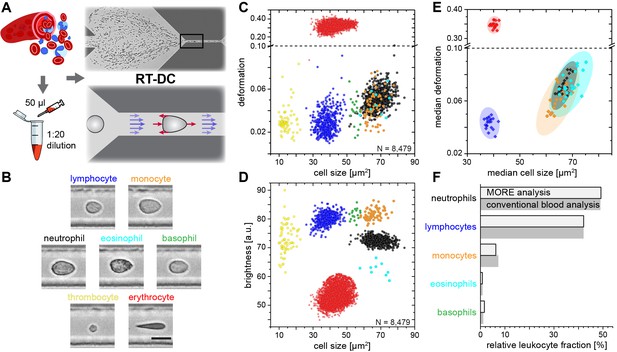
Single-cell, morpho-rheological phenotyping of blood.
(A) Analysis of whole, diluted blood. Hydrodynamic shear forces (red arrows) induce deformation of cells passing through a microfluidic channel (20 × 20 µm2) at speeds of more than 30 cm/s (blue arrows). (B) Representative images of blood cell types acquired. Scale bar is 10 µm. Images are analyzed for cell size as well as (C) cell deformation and (D) average cell brightness. Each dot represents one of N measurement events. (E) Normal range of deformation and size of cell populations from healthy donors. Each diamond represents the median of one donor; transparent ellipses indicate 95% confidence areas. (F) Comparison of MORE cell counts with conventional blood count.
-
Figure 1—source data 1
Source data for Figure 1.
- https://doi.org/10.7554/eLife.29213.010
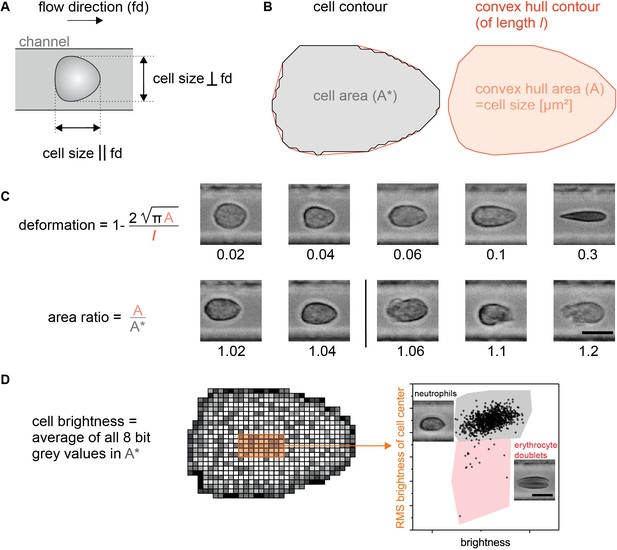
Definition of RT-DC parameters and illustration of gates.
(A) Gating by cell dimensions in µm during RT-DC measurements. A minimum for the size of a cell parallel and perpendicular to the flow direction can be set; so can independent values for the maximum in both directions. Setting the minimum to 5 µm pre-excludes erythrocytes and most thrombocytes and focuses the measurement on leukocytes only. Due to their strong deformation in the channel, erythrocytes have a typical size of only 3 µm perpendicular to the flow. (B) The relation between the detected cell contour and the convex hull contour. The convex hull is used for contour smoothing in the calculation of the deformation parameter (see C) as well as for excluding strongly irregular cells whose shape is not primarily the result of the deforming hydrodynamic forces in the channel. This exclusion is mediated by a limit for the area ratio between the convex hull area and the cells original area (see also C). (C) Calculation of deformation and area ratio as well as five examples of cells per parameter to illustrate the differences. A deformation of 0 and an area ratio of 1 would belong to a perfectly smooth circle. The typical upper limit for the area ratio is set to 1.05, e.g. for all leukocytes. (D) Cell brightness analysis. When gating for leukocyte subpopulations the mean brightness of all pixel values within the cell contour is used. In addition, the root mean square (RMS) value of the pixel values in a 5 × 9 pixel area around the cell’s center is used. This allows to get rid of possible erythrocyte doublets. Note: The main brightness difference between neutrophils and monocytes is found in a region close to the cell’s contour. Also other parameters calculated from the pixel values within the contour, such as the standard deviation, reveal differences between the cell types but are not used in this work.
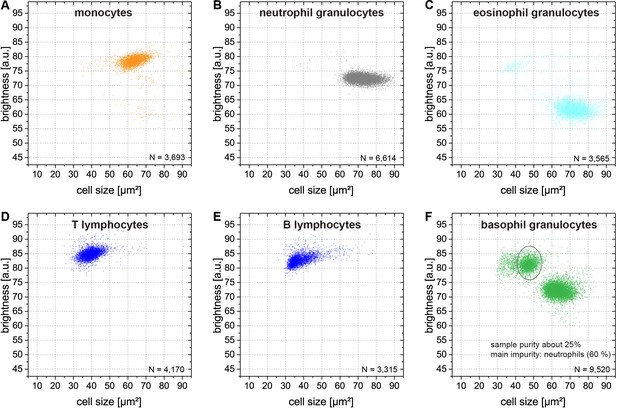
Brightness and cell size of purified leukocyte subpopulations in MORE analysis.
(A) Monocytes (>70% pure, as determined by FACS analysis). (B) Neutrophils (>95% pure). (C) Eosinophils (>90% pure). (D) T lymphocytes (>95% pure). (E) B lymphocytes (>90% pure). (F) Basophils, indicated by the grey ellipse (25% pure, impurities: 63% neutrophils, 12% natural killer cells; concurrently assessed by FACS and MORE analysis). While monocytes, neutrophils, and eosinophils overlap by cell size in the range of 55 to 90 µm2 the cell types are well separated by their cell image brightness without overlap. The brightness of lymphocytes is similar to the brightness of monocytes but cell size is separating both populations without overlap.
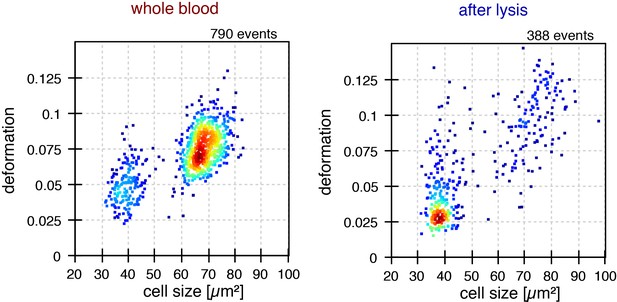
Effect of red blood cell lysis on morpho-rheological properties of leukocytes.
Prior to flow cytometric analysis and many other in vitro assays targeting peripheral blood or lymphoid tissue suspensions, RBC are commonly removed. To test the effect of the RBC lysis procedure on leukocyte size and deformation we prepared samples according to the manufacturer’s advice (BD Pharm Lyse 555899). Besides using the lysing solution, the procedure comprises gentle vortexing and two centrifugation steps (200 g for 5 min), which might all affect the cells. The resulting cell pellet after RBC lysis was suspended in 50 µl autologous serum and 950 µl measurement buffer. As control, 50 µl whole blood of the same donor was suspended in 950 µl measurement buffer. Comparison of the morpho-rheological features of leukocytes showed that the RBC lysis procedure inverted the relative amounts of lymphocytes and myeloid cells, yielded lymphocytes that were less deformed, and myeloid cells that were larger and more deformed. Therefore, we consistently used whole blood for analysis, rather than pre-analytical RBC lysis, which also saves time and cost.
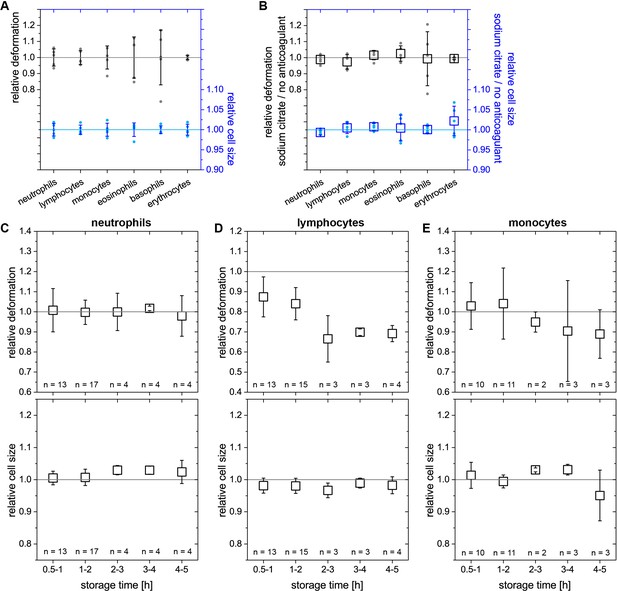
Stability of results with anti-coagulant and storage time.
(A) Variation of deformation (left axis, black) and cell size (right axis, blue) of leukocytes and erythrocytes in the experimental procedure of MORE blood analysis. Blood of the same donor was repeatedly drawn and measured (n = 5 repeats, individual median values as small dots, values normalized by the means thereof, whiskers show ±standard deviation). (B) Possible effects of the anticoagulant sodium citrate on the MORE analysis of blood cell deformation and size were investigated by comparing measurements of sodium citrate blood with freshly drawn blood directly (within 2 min) diluted in the measurement buffer. Neither deformation (left axis, black) nor cell size (right axis, blue) were affected by the anticoagulant for any leukocytes or erythrocytes (n = 5 donors, individual median values as small dots, means thereof as open squares). (C–E) Sodium citrate storage effects at room temperature over time regarding deformation (top panel) and cell size (bottom panel) of the majority of leukocytes (>95%) – neutrophils, lymphocytes, and monocytes. Relative values were calculated against the respective initial measurement directly (within 20 min) post blood drawing. Within the first two hours stable results are obtained with the single exception for the deformation of lymphocytes. But also lymphocytes deformation remains stable within 30 to 120 min post blood drawing after an initial drop of about 10–15% (mean values of individual median values as open squares). Of note, in pathological conditions deformation and size change after drawing blood, so that the amount of change itself could actually be a diagnostic parameter. An advantage of RT-DC is that leukocyte mechanics can be measured within 15 min after blood drawing, which is not possible with other techniques where significant time is spent on separation/preparation. All error bars represent the standard deviation.
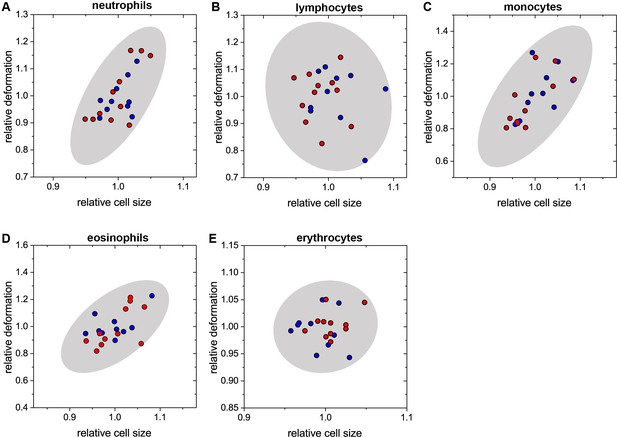
Inter-donor variation of deformation and cell size.
Values shown are median values for each donor’s individual cell types, normalized by the mean for all 21 donors (10 male – blue; 11 female – red) for each respective cell type. See also Main Text Figure 1E for absolute values. Relative values are used for better illustration of the variance within the different cell types: (A) neutrophils; (B) lymphocytes; (C) monocytes; (D) eosinophils; (E) erythrocytes. Each panel contains the gray 95% confidence ellipse displaying the norm for the respective cell types. Comparison of the male and female groups shows no significant difference for any of the cell types.
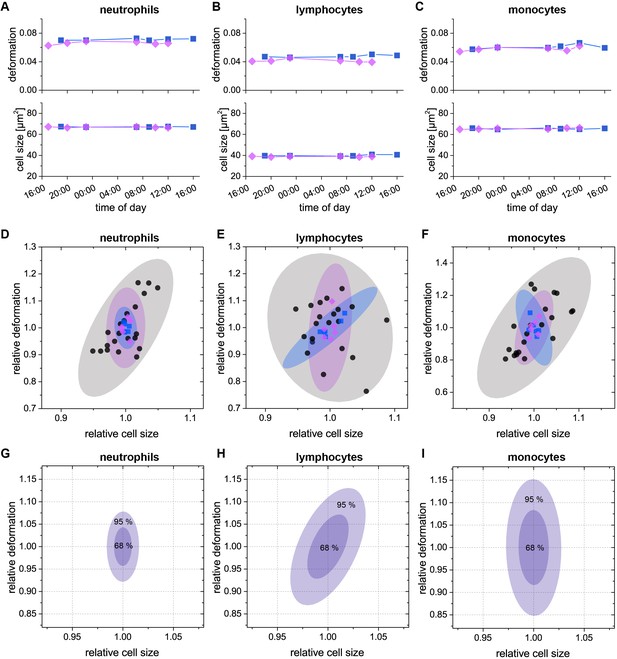
Intra-day variation of deformation and cell size.
In a 24 hr time course we monitored the intra-day variation of the median values of deformation and cell size for (A) neutrophils; (B) lymphocytes; (C) monocytes and so, typically, more than 95% of all leukocytes (two donors; donor 1: blue, donor 2: magenta). The time course started in the afternoon/evening of the first day and ended at noon/afternoon of the following day. For the first measurement in the morning, blood was drawn from the donor prior to getting up. Variation in deformation was small and even less in cell size and compared in range with our experimental uncertainty as determined in Figure 1—figure supplement 4A. The small variance is illustrated in (D–F) by comparing the individual donor intra-day variation to the inter-donor variation from Figure 1—figure supplement 5. For each donor, the relative deformation and cell size values were obtained by normalization to their respective mean values of the 24 hr time course. In addition to the data points (same color and shape coding as in A-C) the 95% confidence ellipses are shown as shadows in the same color. The small intra-day variation of size and deformation led us to conclude that the variation within physiological blood sugar, electrolyte, and hormone levels as well as daily cell count variation did not call for special measures regarding the drawing of blood for MORE analysis, e.g., at a certain time of day, or fasting. (G–I) 68% (inner shade) and 95% (outer shade) confidence regions of a single measurement for (G) neutrophils; (H) lymphocytes; and (I) monocytes. The single measurement confidence is based on 12 repeated measurements in total for the two donors in (A–C). Therefore, the confidence regions reflect possible variations for the individual cell types on a technical level, such as small differences in sample handling, as well as on the biological level, such as intra donor variations.
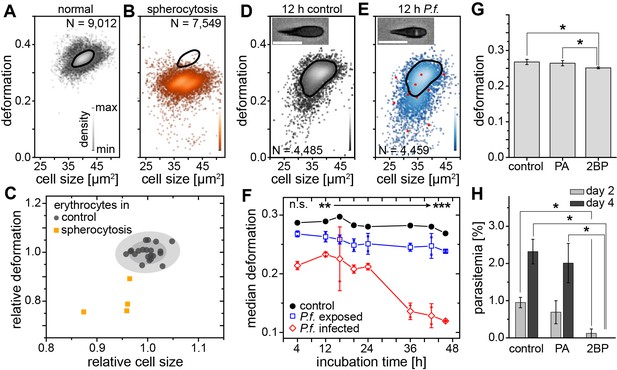
Detection of RBC pathologies — spherocytosis and malaria.
Exemplary density plots of RBC size vs. deformation in samples from (A) healthy donor and (B) patient with spherocytosis. (C) Relative median RBC deformation and size in patients with spherocytosis (orange, n = 4 patients) compared to controls (black, n = 21 donors as in Figure 1E with 68% and 95% confidence ellipses). Density plots of size vs. deformation of (D) control RBCs and (E) RBCs exposed to P.f. (blue), both after 12 hr incubation. Insets show cells with and without image feature (white inclusion), positively indicating actual parasite infection. Infected RBCs are indicated by red dots. Scale bars, 10 µm. (F) Evolution of RBC deformation over 46 hr time course of control (black), P.f. exposed (blue) and P.f. infected RBCs (red); open squares and diamonds, mean ±SD, n = 2; filled squares, individual medians, **p<0.01, ***p<0.001. (G) Reduced deformation of 2BP-treated RBCs compared to PA- and non-treated controls (mean ±SD of population medians, n = 4 donors, *p<0.05). (H) Reduced parasitemia in 2BP- compared to PA- and non-treated controls at 2 and 4 days post infection. Error bars: SD binomial, *p<0.0125.
-
Figure 2—source data 1
Source data for Figure 2.
- https://doi.org/10.7554/eLife.29213.016
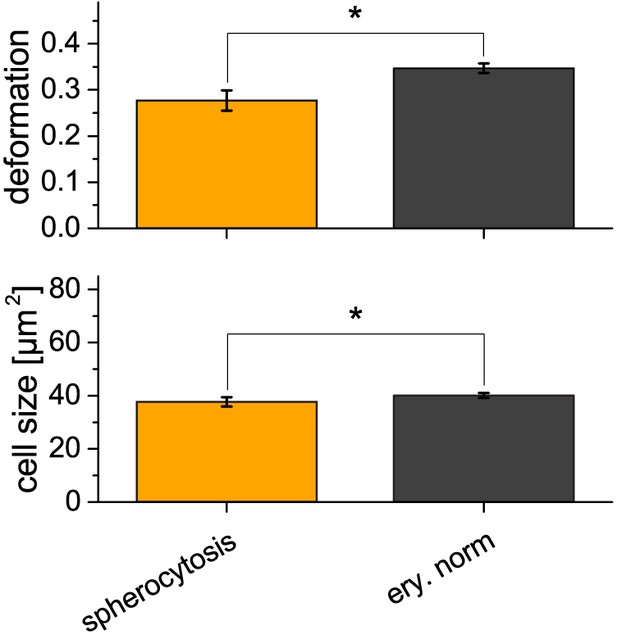
Comparison of erythrocytes in spherocytosis with the healthy control.
Erythrocytes in spherocytosis (n = 4 patients, see also main text Figure 2C) show a statistically significant lower deformation of 0.28 ± 0.02 compared to the healthy norm (n = 21 donors, known from main text Figure 1E and Figure 1—figure supplement 5) with a deformation of 0.35 ± 0.01 (mean ±SD, p=0.002). The cell size in spherocytosis was found to be significantly smaller at 37.8 ± 1.8 µm2 compared to 40.1 ± 0.9 µm2 (mean ±SD, p=0.004) for erythrocytes in the healthy control group. Asterisks indicate statistically significant differences, p<0.05, by Kruskal-Wallis test. Error bars represent the standard deviation.
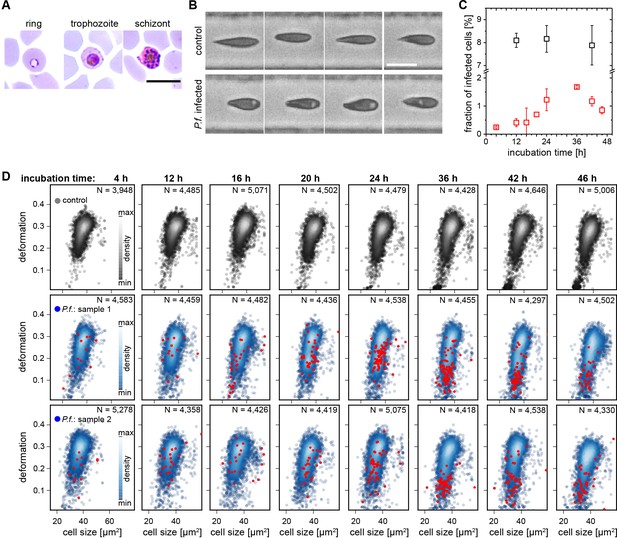
In vitro infection of erythrocytes with Plasmodium falciparum.
(A) Erythrocytes at different stage of infection in a standard blood smear. Scale bar 10 µm. (B) Pictures of erythrocytes in MORE analysis. Cells displaying distinct bright spots are exclusively found in P.f. exposed and infected samples and are therefore considered infected cells. Scale bar 10 µm. (C) Parasitemia of in vitro infection experiments as determined from the blood smear (black) and the fraction of cells clearly detected as infected cells by MORE analysis (red) over the time course of 46 hr. The efficiency of the detection of infected cells in MORE analysis increases with time, possibly due to the growth of the parasites. The deviation from the parasitemia as determined by the blood smear is in part caused by conservative gating settings for the automated identification of infected cells in MORE analysis with the goal of preventing false positive results. The difference could in part also stem from the bright spot being the vacuole forming inside the cell, and not the actual parasite. (D) 46 hr time course of an in vitro malaria infection in erythrocytes displaying the development of deformation and cell size of the erythrocyte populations in the control sample (top row) and two separate exposed and infected samples (middle and bottom row). In addition, red dots indicate those cells identified as infected by MORE analysis. The time course of the median deformation values of the populations is summarized in main text Figure 3C.
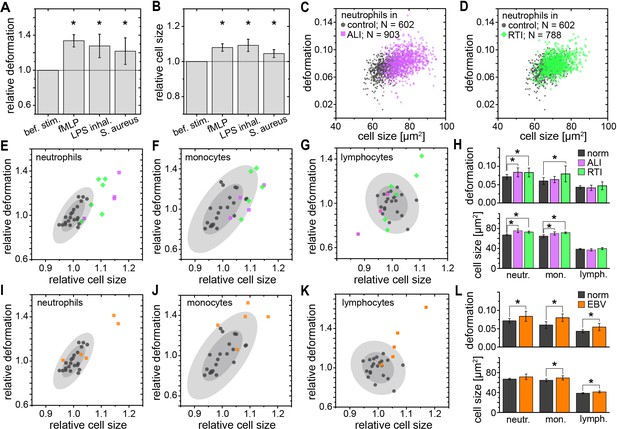
Identification of leukocyte activation and infection in vitro and in vivo.
Relative change (mean ±SD) in (A) deformation and (B) size of neutrophils in diluted whole blood after fMLP (n = 5 donors; see Figure 3—figure supplement 1) and S. aureus (n = 4 donors; see Figure 3—figure supplement 2) stimulation in vitro measured 15–30 min and 30–60 min after stimulation, respectively, and LPS inhalation (n = 2 donors; see Figure 3—figure supplement 1) in vivo measured 135 min after inhalation. Exemplary scatter plots of size vs. deformation of neutrophils in blood of a patient with (C) ALI (magenta) and (D) RTI (green) compared to controls (black). Medians of size and deformation of (E, I) neutrophils, (F, J) monocytes, and (G, K) lymphocytes in blood samples of patients with E, F, G, ALI (n = 4 patients; magenta) and RTI (n = 6 patients; green), and I, J, K, EBV infection (n = 5 patients; orange) relative to the norm (black, n = 21 donors as in Figure 1E with 68% and 95% confidence ellipses). (H, I) Mean and SD of these results, *p<0.05. For typical scatter plots of size vs. deformation of all three cell types and all three disease conditions see Figure 3—figure supplement 3.
-
Figure 3—source data 1
Source data for Figure 3.
- https://doi.org/10.7554/eLife.29213.021
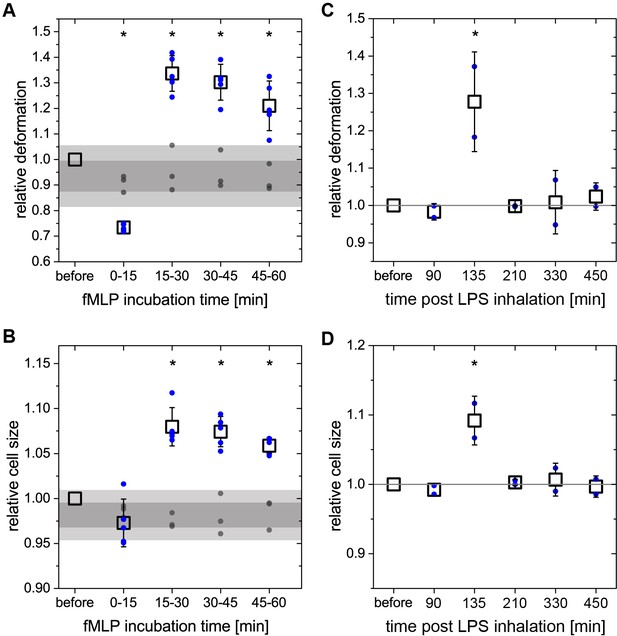
Neutrophil response in blood during in vitro and in vivo stimulation.
(A and B) change of deformation and cell size of neutrophils over time after in vitro fMLP stimulation of diluted whole blood. Relative median values are obtained by normalization to the respective values before stimulation. Blue dots: individual median values of stimulated samples, n = 5 donors. Open squares: mean values thereof. Gray dots: individual median values of control samples treated equally to stimulated samples without fMLP, n = 3 donors. Gray bars: Intervals, indicating 68% and 95% confidence of all control measurements (n = 12). Asterisks indicate statistically significant differences between all control measurements and stimulated samples, p<0.05. After fMLP stimulation, neutrophils show an initial drop in deformation and cell size, followed by a complete change to significantly larger and more deformed cells at later stages of the stimulation. (C) and (D) change of deformation and cell size of neutrophils in patient blood after LPS inhalation. Blood samples were drawn freshly at each time point and measured within 15 min after blood withdrawal. Relative median values are obtained by normalization to the respective values prior to stimulation. Blue dots: individual median values of stimulated samples, n = 2 donors. Open squares: mean values of thereof. Asterisks indicate statistically significant differences to the reference measurements before stimulation, p<0.05. At two hours post LPS inhalation there was a significant increase in both deformation and cell size of neutrophils in the blood of otherwise healthy human volunteers. These values returned to and remained at the baseline one hour later. All error bars represent the standard deviation.
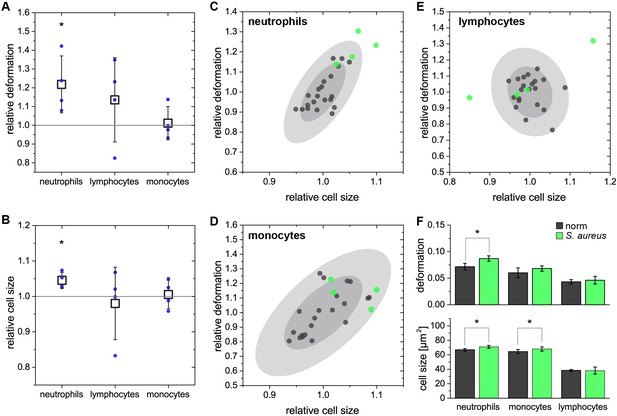
In vitro stimulation of blood with Staphylococcus aureus.
(A and B) change of deformation and cell size shown for neutrophils, lymphocytes and monocytes of S. aureus stimulated blood relative to the respective control before stimulation. Blue dots: medians of stimulated blood cells, n = 4 donors; open squares: mean values and standard deviation of four experiments; asterisks indicate differences to the reference measurements before stimulation, p<0.05 by Kruskal-Wallis test. Cell size and deformation of stimulated neutrophils are significantly increased when compared to the baseline before stimulation. Lymphocytes show strong variations but without any clear trend that is also not found for monocytes. A comparison of S. aureus stimulated blood and the healthy norm known from Figure 1—figure supplement 5 reveals similar results. In panels (C–E) the norm is shown by the individual median values (gray dots, n = 21 donors) for (C) neutrophils; (D) monocytes; and (E) lymphocytes with the 68% and 95% confidence ellipses as gray shadows. Median values of the stimulated samples (n = 4 donors) are displayed as green hexagons and normalized to the average values of the norm. (F) Separate quantification of deformation and cell size of data shown in C-E as mean and standard deviation. Asterisks indicate statistically significant differences to the norm (p<0.05 by Kruskal-Wallis test).
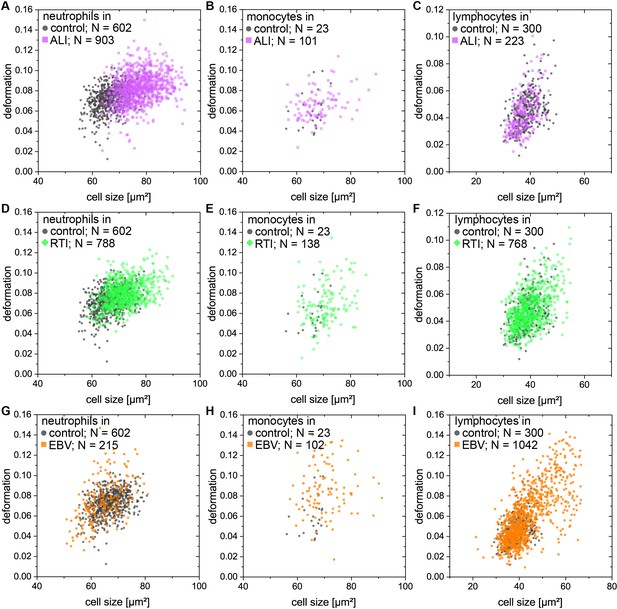
Single cell distributions of neutrophils, lymphocytes, and monocytes from patients with ALI, RTI, and EBV compared to controls.
(A-C) exemplary scatter plots of size vs. deformation of (A) neutrophils; (B) monocytes; and (C) lymphocytes in the blood of a patient with ALI (magenta) compared to controls (black). (D–F) exemplary scatter plots of size vs. deformation of (D) neutrophils; (E) monocytes; and (F) lymphocytes in blood of a patient with RTI (green) compared to controls (black). (G–I) exemplary scatter plots of size vs. deformation of (G) neutrophils; (H) monocytes; and (I) lymphocytes in blood of a patient with EBV (orange) compared to controls (black).
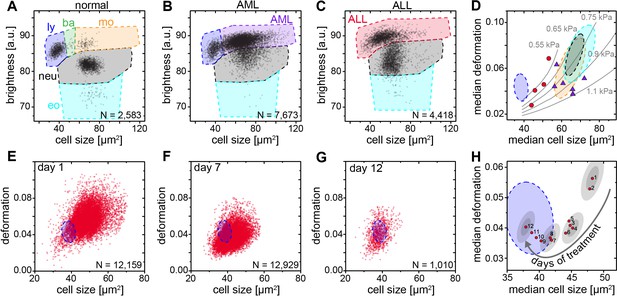
Detection and distinction of leukemia subtypes and monitoring of treatment effects.
(A) Normal brightness vs. size scatter plot of a healthy donor with the gates (shaded areas) used to identify lymphocytes (ly), basophils (ba), monocytes (mo), neutrophils (neu) and eosinophils (eo). (B) Exemplary brightness vs. size scatter plot in AML; blast cells were found in (ba) and (mo) gates. (C) Exemplary brightness vs. size scatter plot in ALL; blast cells were found in (ly), (ba), and (mo) gates. (D) Medians of deformation and size for the respective gates in blood samples of ALL (red circles, n = 4 patients) and AML patients (purple triangles, n = 7 patients). Shaded areas in D (color as in A) represent 95% confidence ellipses of the respective cell type norm (n = 21 donors, as in Figure 1E). Gray lines represent lines of equal elasticity calculated for purely elastic objects. Scatter plots of ALL blast deformation and size at (E) one; (F) seven, and (G) twelve days post therapy start. Blue shaded areas in E-H represent 95% confidence ellipses of the lymphocyte norm (n = 21 donors, as in Figure 1E). (H) Median deformation and size of ALL cells during 12 days of treatment (red dots, days as numbers). Gray shaded areas surrounding data of days 1, 4, 8, and 12 represent the 68% (inner) and 95% (outer) confidence area of a single measurement (according to lymphocyte confidence in Figure 1—figure supplement 6H).
-
Figure 4—source data 1
Source data for Figure 4.
- https://doi.org/10.7554/eLife.29213.024
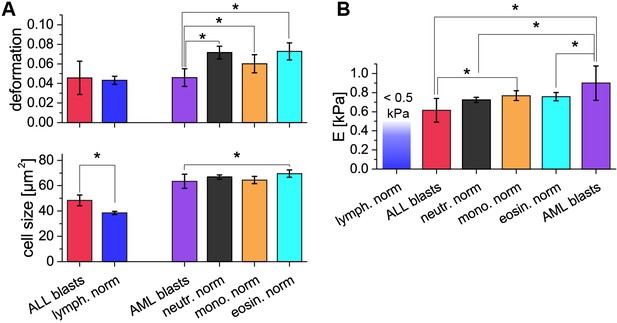
Comparison of ALL and AML blast cells with the norm.
(A) Deformation and cell size of ALL blasts (n = 4 patients, see also main text Figure 4D) are compared to the lymphocyte norm (n = 21 donors, known from main text Figure 1E and Figure 1—figure supplement 5B). ALL blasts were significantly larger than lymphocytes, while no trend – but a large variation – is detected for the deformation. Deformation and cell size of AML blasts (n = 7 patients, see also main text Figure 4D) are compared to the norm of neutrophils, monocytes and eosinophils (n = 21 donors, known from main text Figure 1E and Figure 1—figure supplement 5D). AML blasts were significantly less deformed than the norm of the three cell types. At the same time the cell size did only show significant differences when compared to the larger eosinophils. (B) Comparison of the apparent Young’s modulus of ALL (n = 4 patients, see also main text Figure 4D) and AML (n = 7 patients, see also main text Figure 4D) blasts with each other and the norm of neutrophils, monocytes and eosinophils (n = 21 donors, known from main text Figure 1E and Figure 1—figure supplement 5) when treated as purely elastic. ALL blasts are significantly more compliant compared to AML blasts and the norm of monocytes. No statistically significant difference was found in the comparison of ALL blasts and the norm of neutrophils and eosinophils. AML blasts are also significantly stiffer compared to the norm of neutrophils and eosinophils but no statistically significant difference was found in the comparison to the norm of monocytes. Only an upper estimate for the apparent Young’s modulus of 0.5 kPa can be given for lymphocytes because most deformation and size values for this cell type are located outside the region of stable results from the numerical simulation that was used to convert deformation and cell size data to apparent Young’s moduli. Asterisks indicate statistically significant differences, p<0.05 by Kruskal-Wallis test. Error bars represent the standard deviation.
Videos
RT-DC in action.
Video of the microfluidic channel system during RT-DC measurement of diluted whole blood. The cell suspension flows from left to right through the channel. Cells enter on the left and are focused by sheath flow from the top and bottom of the frame towards the narrow RT-DC measurement channel of 300 µm length and 20 µm width and height in the right half of the image. RT-DC measurements are carried out on the cells that travel through the last third of the length of the measurement channel.
3D visualization of the separation of leukocyte populations.
Rotating angle view in the space of deformation, cell size and cell brightness. Cell identification in order of appearance by coloring: lymphocytes (blue), neutrophil granulocytes (black), eosinophil granulocytes (cyan), monocytes (orange), basophil granulocytes (green).
Additional files
-
Supplementary file 1
Table of relative blood counts by MORE analysis and conventional analysis.
Percentage of all leukocytes identified by MORE analysis compare to conventional full blood cell counts, obtained with Sysmex XE-5000 differential analyzer and verified by a microscopic differential count, of four donors, two male (A, C), two female (B, D). The absolute cell counts per volume obtained by MORE analysis differ from the values of the conventional blood count, since some cells are not detected (up to 40% of all cells). However, this affects all leukocytes similarly so that the relative counts are not changed.
- https://doi.org/10.7554/eLife.29213.025
-
Supplementary file 2
Table comparing conventional biomarkers of leukemia with MORE analysis.
(1) Morphological analysis of air-dried Romanowsky (Wright, Wright-Giemsa, or May-Grünwald- Giemsa)-stained blood or bone marrow smears. The morphological features identified by microscopic examination may suggest either lymphoid or myeloid differentiation of leukemic cells, but with the exception of the identification of Auer rods in myeloblasts none of these features is lineage-specific. Sub-clones can be identified by differences in size and morphological features (e. g. cytoplasmatic vacuoles). (2) Cytochemical staining improves the accuracy and reproducibility of lineage assessment and therefore is required for traditional sub-classification of acute myeloid leukemia (AML) according to the French-American-British (FAB) and WHO criteria. Sudan Black and stains for myeloperoxidase (MPO) to identify myeloblasts and esterase stains like alpha-naphthyl-butyrate to identify monoblasts have remained useful in this regard. Staining must be performed without undue delay as MPO is unstable and becomes undetectable after a week of storage. (3) Immunophenotypic classification is based on identification of cell surface epitopes or cytoplasmatic proteins by fluorescent dye-labeled antibodies. Flow cytometry (fluorescence-activated cell sorting, FACS) is nowadays widely used as a particularly powerful method because multiparameter analysis offers the advantage of segregating leukemic cells from non-neoplastic cells. Thus, rapid analysis allows to establish the lineage of the leukemia (e.g. myeloid versus lymphoid), its stage of differentiation (e. g. T- versus B-ALL) and facilitates minimal residual disease (MRD) monitoring using a leukemia-specific pattern of markers not expressed in that combination on regular blood or bone marrow cells. Notably, some precursor B-cell ALL might be negative for CD45 (leukocyte common antigen) or patients with T-ALL lack TdT or CD34 expression. Although ALL can be classified according to the stage of maturation, the optimal immunologic sub-classification remains a matter of debate. Many ALLs also aberrantly express myeloid-linage associated antigens (mostly CD13, CD33). Therefore the antibody screening panel for acute leukemias must be designed to include at least one very sensitive and one relatively specific marker for each hematopoietic and lymphoid lineage. (4) Molecular (genetic) classification using traditional methods will detect specific cytogenetic and/or molecular abnormalities in 60–80% of ALL and 50–60% of AML cases. The recent advent of whole genome analysis has allowed virtually all acute leukemias to be classified according to specific genetic abnormalities. Markers can be separated into leukemia-specific (e.g. BCR-ABL1; t(15;18)) or leukemic-clone specific (e.g. Ig-heavy chain gene rearrangements, T-cell receptor gene rearrangements). Both are valuable for classification, as prognostic indicators with a defined treatment applied, and are nowadays routinely used for monitoring of MRD by exploiting the high sensitivity of PCR-based amplification of specific gene sequences. The technique is time-consuming and expensive, and usually performed only in reference laboratories. (5) MORE analysis. When compared to these established conventional methods, the advantages of morpho-rheological (MORE) phenotyping are characterized by a very short time for analysis and the minimum amount of blood required. The technique has comparable power with regard to the identification of leukemic cells and the identification of leukemic sub-clones. Its applicability to classify the leukemic lineage (for example by significant differences in size, deformation, and Young’s modulus; see Figure 4—figure supplement 1) and to detect small numbers of leukemic cells can theoretically be expected and has been shown in single cases already, but still has to be tested and proven in a formal comparison, which is beyond the scope of the present study. Potentially, the rheological features of blast cells might represent additional prognostic biomarkers for leukemic cells (stiffness might correlate to drug sensitivity or refractoriness, or identify a leukemic subclone), which will be the subject of future studies. Morpho-rheological phenotyping, thus, compares very well to established biomarkers for following ALL treatment success.
- https://doi.org/10.7554/eLife.29213.026
-
Transparent reporting form
- https://doi.org/10.7554/eLife.29213.027