Signaling pathways as linear transmitters
Figures
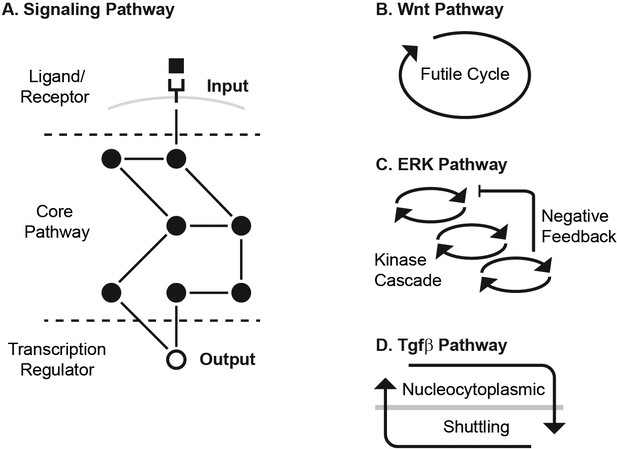
The Wnt, ERK, and Tgfβ pathways transmit input using different core transmission architecture.
(A) Signaling pathways transmit inputs from ligand-receptor interaction to a change in output, the level of transcriptional regulator (white circle). (B-D) The core pathway for each metazoan signaling pathway is defined by distinct architectural features. In the Wnt pathway (B), the output is regulated by a futile cycle of continual synthesis and rapid degradation. In the ERK pathway (C), the output is regulated by a kinase cascade coupled to negative feedback. In the Tgfβ pathway (D), the output is regulated through continual nucleocytoplasmic shuttling.
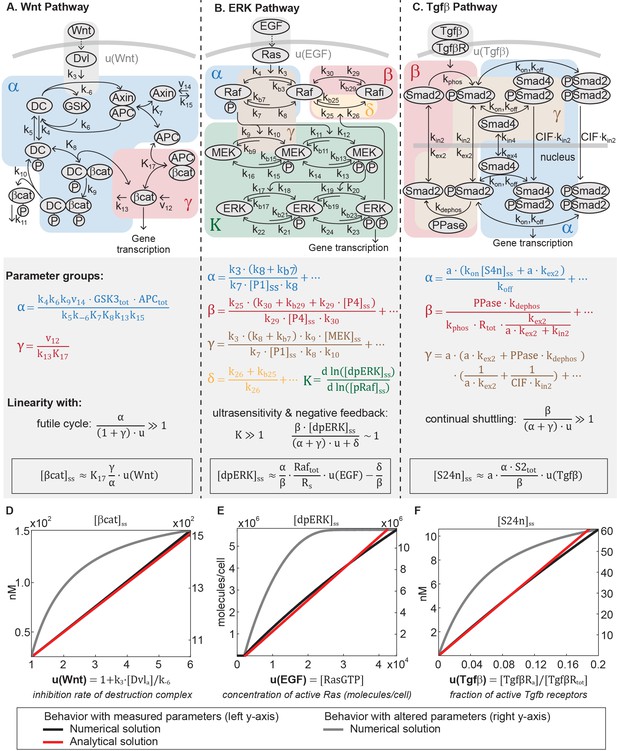
The Wnt, ERK, and Tgfβ pathways are linear signal transmitters.
(A-C) Network diagrams of the signaling pathways. The Tgfβ diagram is modified from Schmierer et al. (2008). In the network diagram in A, DC refers to the β-catenin destruction complex. Below the network diagrams: the parameter groups and linearity equations we analytically derived in this study. Parameter groups and input functions are color-coded to the corresponding reactions in the network diagrams. Parameters that do not appear in the parameter groups either drop out due to irreversible reaction steps (such as k10 and k11 in the Wnt pathway) or negligible (as indicated by ellipses). (D-F) Our analysis reveals that in physiologically relevant parameter values, these pathways generate a linear input-output relationship. The outputs are β-catenin, dpERK, and nuclear Smad complex for the Wnt, ERK, and Tgfβ pathway, respectively. The input functions describe the effect of ligand-receptor interactions on the core pathway. Specifically: is the rate by which Dishevelled/Dvl inhibits the destruction complex upon Wnt ligand activation, where and are defined in the figure and [Dvl]a is the concentration Wnt-activated Dishevelled (see Equations A15); u(EGF) is concentration of EGF-activated Ras (Ras-GTP); and u(Tgfβ) is the fraction of Tgfβ -activated receptors. Red and blue lines, respectively: analytical and numerical solutions with measured parameters (plotted against the left y-axis). Grey line: examples of numerical solutions outside measured parameters (plotted against the right y-axis).
-
Figure 2—source code 1
- https://doi.org/10.7554/eLife.33617.011
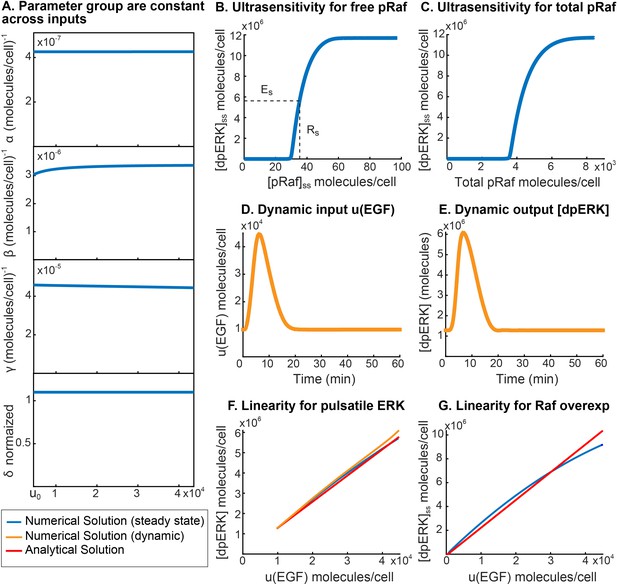
Model simulations for the ERK pathway.
(A) Parameter groups in the ERK model are constant to within 10%, over the physiologically relevant range of u considered here, justifying the inclusion of variables into the parameter groups. (B–C) The dpERK output is an ultrasensitive function of both free and total phosphorylated Raf. The values and are illustrated in (B), and are defined in Appendix 1. (D–F) Numerical simulation of pulsatile response in the ERK pathway. (D) A pulse of input, RasGTP, is generated by EGF addition in an ERK model that includes details of receptor desensitization (Schoeberl et al., 2002). Basal activity of Ras is included to ensure constitutive negative feedback (Fritsche-Guenther et al., 2011). (E) dpERK output also exhibits a pulsatile response, peaking within 10 min. (F) We plot the peak dpERK output against peak input for a range of physiologically relevant u(EGF) doses, and find that it matches our steady-state predictions for linear input-output behavior. (G) Five-fold Raf overexpression does not break the linear input-output behavior.
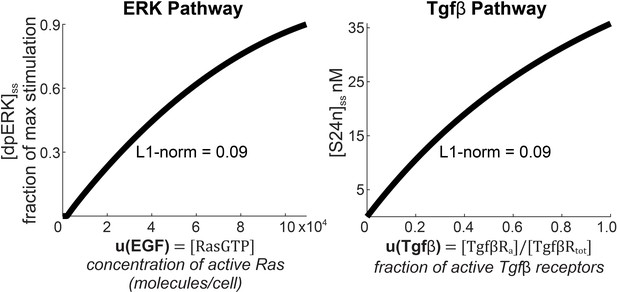
The predicted linearity extends throughout the dynamic range of the ERK and Tgfβ pathways.
(A–B) Numerical simulation of the ERK and Tgfβ models. (A) The ERK model shows linear input-output relationship up to 93% of dpERK activation. (B) The Tgfβ pathway shows linear input-output relationship throughout the entire input range (from 0 to 1). Linearity was analyzed using the L1-norm or least absolute deviation (see Materials and methods). The blue range indicates where L1-norm was computed.
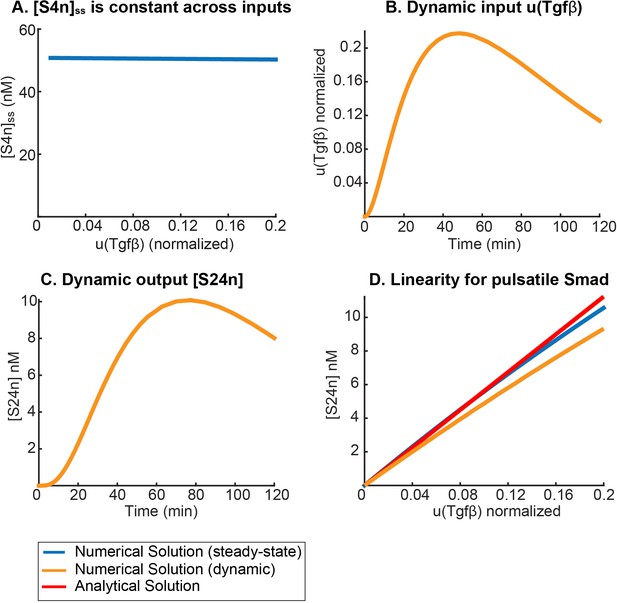
Model simulations for the Tgfβ pathway.
(A) Nuclear Smad4 concentration is constant to within 2%, over a physiologically relevant range of u(Tgfβ) considered here, justifying its inclusion into parameter group . (B–D) Numerical simulation of pulsatile response in the Tgfβ pathway. (B) A pulse of input, active Tgfβ receptor, is generated by Tgfβ addition in a model that includes details of receptor desensitization (Vizán et al., 2013). (C) S24n output also exhibits a pulsatile response. (D) We plot the peak S24n output against peak input, and find that it matches our steady-state predictions for linear input-output behavior.
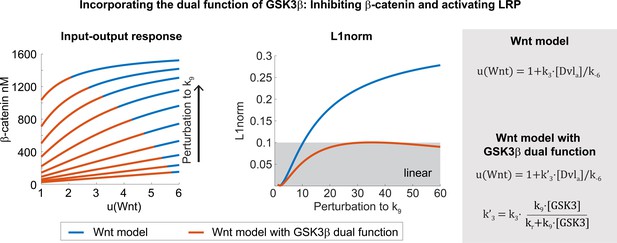
Incorporating into the Wnt model the dual function of GSK3β in phosphorylating β-catenin and LRP5/6.
We include the role of GSK3β in phosphorylating LRP5/6 into the input function u(Wnt), such that u(Wnt) is a function of GSK3β and the phosphorylation rate k9 (for simplicity, the same rate as GSK3β phosphorylation of β-catenin), and the reverse rate kr. In both models, β-catenin increases in response to GSK3β inhibition (e.g., by CHIR99021). However, only the model with the dual function of GSK3β shows a decrease in input range that we observed experimentally.
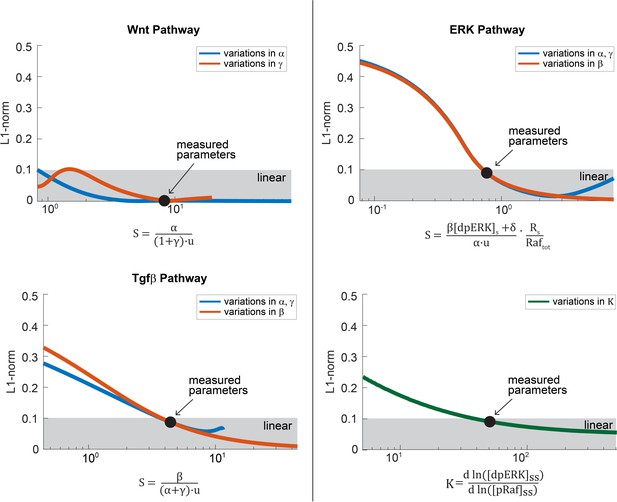
The requirements for linear signal transmission in the Wnt, Tgfβ, and ERK pathway.
In each plot, we varied S, defined in the equation shown on the x-axis, and simulated the input-output curve over the dynamic range of the model. The parameters in the equations are as defined in the main text. For the ERK and Tgfβ pathway, and γ are linked in such a way that they could not easily be varied independently. Linearity was assessed using the L-1 norm, which ranges from 0 to 0.5, with L-1 norm < 0.1 indicating linearity. L1-norm analysis was performed over the full dynamic range of the system, i.e., u(Wnt) = 1- 6, u(ERK) = 0 to 110,000 molecules of Ras-GTP, which gave 90% activation of [dpERK] in unperturbed cells, and u(Tgfβ) = 0 to 1.
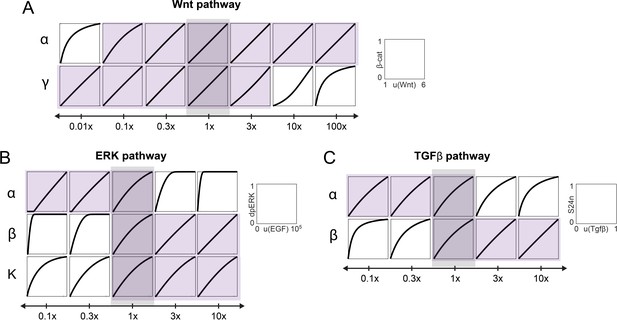
Linear signal transmission occurs over a range of parameters in the model.
In this analysis, the parameter groups in each model were varied as indicated e.g., 3x is three-fold increase, 0.3x is three-fold decrease. 1x corresponds to the measured parameters. Plotted in each box is the input-output relationship, numerically simulated over the full dynamic range of the models, i.e., 1-6 for u(Wnt), 0-105 for u(EGF), and 0-1 for u(Tgfβ). For simplicity, all outputs are normalized from 0 to 1. Grey shade: the unperturbed state. Purple shade: linear input-output response, as defined by L-1 norm < 0.1.
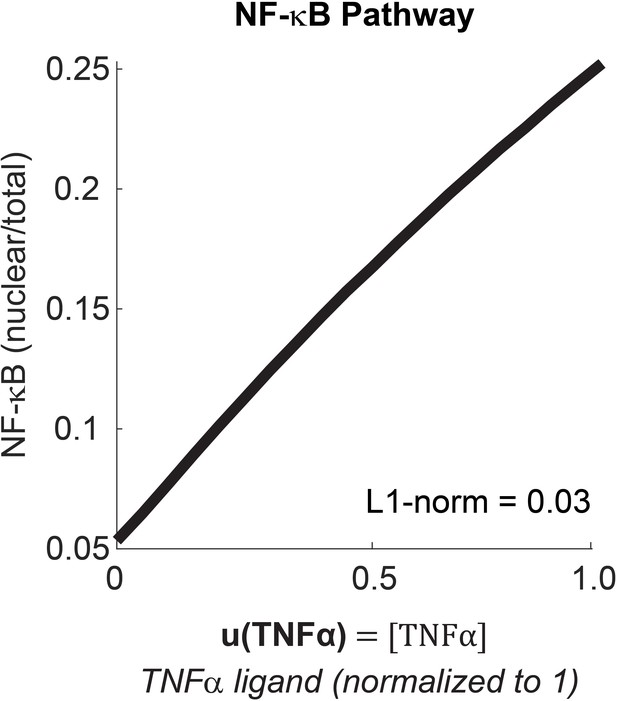
Numerical simulation of the input-output relationship of the NF-κB pathway.
We used the model first built by Hoffman et al. in 2002 (Hoffmann et al., 2002) and later revised by Ashall et al. in 2009 (Ashall et al., 2009). The parameters in the model have been measured or fitted to single-cell dynamics in multiple cell types (Hoffmann et al., 2002; Ashall et al., 2009). We simulated the model here over a physiologically observed dynamic range, i.e., Lee et al. (2014) observed in HeLa cells that at saturating ligand dose (10 ng/mL TNFα, set to one in the model),~25% of NF-κB pool is nuclear. Linearity is assessed using the L1-norm, where L1-norm < 0.1 indicates linear relationship (see Materials and methods).
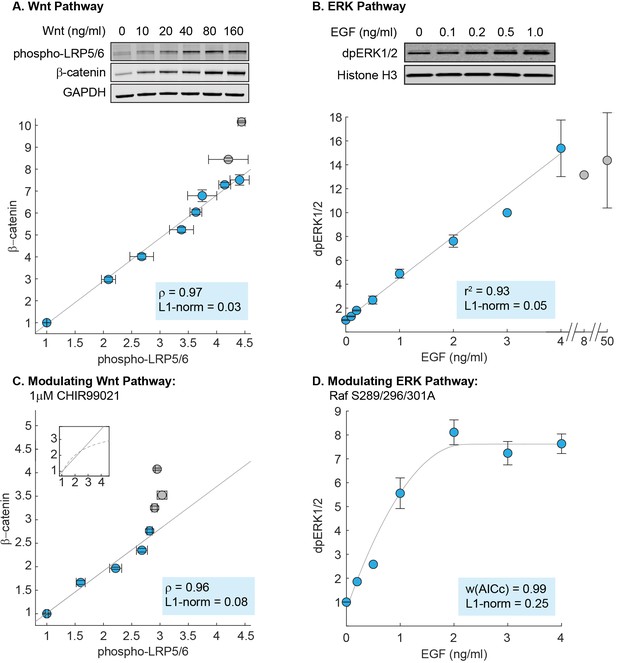
Linearity was observed experimentally in the Wnt and ERK pathways.
(A) Measurements of the input-output relationship in the Wnt pathway. In these experiments, RKO cells were stimulated with 0–1280 ng/mL purified Wnt3A ligand, harvested at 6 hr after ligand stimulation, and lysed for Western blot analyses. Shown on top is a representative Western blot. The data plotted come from seven independent experiments (total N = 66). Each circle indicate the mean intensities of the phospho-LRP5/6 (x-axis) and β-catenin (y-axis) bands for all Western blot biological replicates, and error bars indicate the standard error of the mean. For each gel, we normalize the unstimulated sample (i.e. 0 ng/mL of Wnt3A) to one, and scale the magnitude of the dose response to the average of all gels (described in Materials and methods). The grey line is a least squares regression line, and ρ is the Pearson’s coefficient, where ρ = 1 is a perfect positive linear correlation. (B) Measurements of the input-output relationship in the ERK pathway. In these experiments, H1299 cells were stimulated with 0–50 ng/mL purified EGF ligand, harvested at 5 min after ligand stimulation, and lysed for Western blot analyses. Shown on top is a representative Western blot. The data plotted here come from five independent experiments (total N = 30). Each circle indicates the mean intensities of dpERK1/2 bands across Western blot biological replicates, and the error bars indicate standard error of the mean. Single replicates are plotted without error bars. All data is plotted relative to unstimulated sample. The grey line is a least squares regression line, and r2 is the coefficient of correlation where r2 = 1 is a perfect linear correlation. (C) As in (A), except that cells were treated with 1 μM CHIR99021 (detailed in Materials and methods). The data plotted here come from five independent experiments (total N = 59). The grey line is a least squares regression, and ρ is the Pearson’s coefficient, where ρ = 1 is a perfect positive linear correlation. Shown in the subplot are the same least squares regression line (solid line), overlaid with the model prediction (dashed line). (D) As in (B), but measurements were performed in H1299 cells expressing mutant Raf S289/296/301A. The data plotted here come from three independent experiments (total N = 15). The grey line is a fit using the ERK model. We first fitted the gain of the model to the data (i.e. the y-range), and afterward, varied the strength of dpERK feedback (k25) to find the best fit. We used the weighted Akaike Information Criterion, w(AICc), to verify that the nonlinear fit from the ERK model outperforms a linear least squares fit (see Materials and methods). 0 < w(AICc) < 1, with higher w(AICc) indicates better performance by the non-linear fit. In all figures, linearity was additionally assessed using the least absolute deviations, L1-norm (see Methods). L1-norm can range from 0 to 0.5, with L1-norm < 0.1 indicate a linear relationship. Blue vs grey circles in each figure are explained in the main text. Source files of all Western blot gel images and numerical quantitation data are available in Figure 3—source data 1.
-
Figure 3—source data 1
- https://doi.org/10.7554/eLife.33617.022
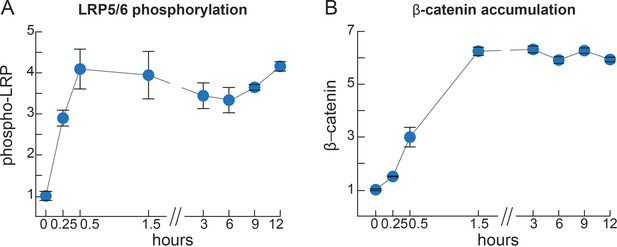
LRP5/6 phosphorylation and β-catenin accumulation are already at steady state at 6 hr after Wnt stimulation.
RKO cells were treated with 160 ng/mL Wnt3A for the specified times, and then assayed for phospho-LRP5/6 and β-catenin level by Western blot. Error bars are standard error of the mean from 2 to 4 biological replicates. Data are plotted relative to the sample at time zero, and normalized to the average maximal activation across experiments. The grey lines connect the mean of each time point.
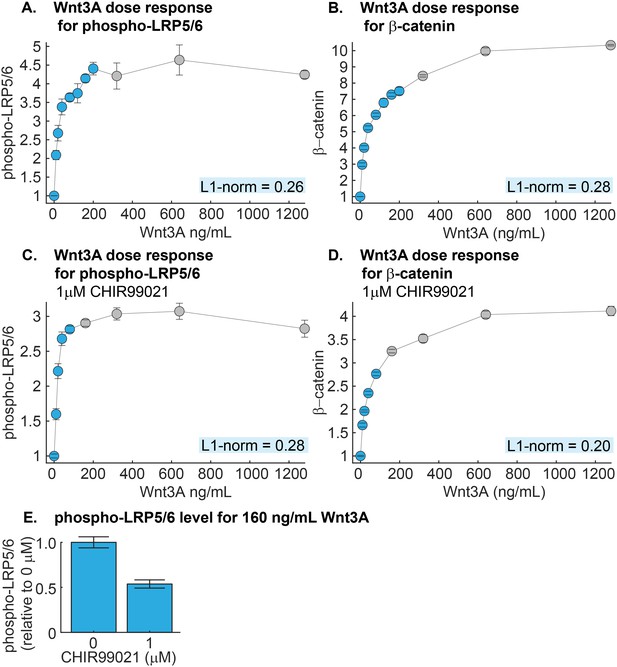
The dynamic range of Wnt signaling in RKO cells.
RKO cells were treated with the specified dose of Wnt3A for six hours, and then assayed for phospho-LRP5/6 and β-catenin by quantitative Western blot. Data are plotted relative to unstimulated samples. (A–B) In wt cells, phospho-LRP5/6 (A) shows > 90% of maximal response at 200 ng/mL Wnt3A, while β-catenin (B) shows 70% of maximal response at 200 ng/mL, and subsequently incremental response until 640 ng/mL Wnt3A. (C–D) In cells pre-treated with 1 µM CHIR99021, phospho-LRP5/6 (C) shows > 90% of maximal response at 80 ng/mL Wnt3A, while β-catenin (D) shows 70% of maximal response at 80 ng/mL and continues incremental activation at higher doses. (E). Cells were treated with 160 ng/mL Wnt3A and assayed for phospho-LRP5/6. Cells pre-treated with 1 µM CHIR99021 (N = 3) exhibited 50% the level of phospho-LRP5/6 as untreated cells (N = 3). The grey lines simply connect the means of data.
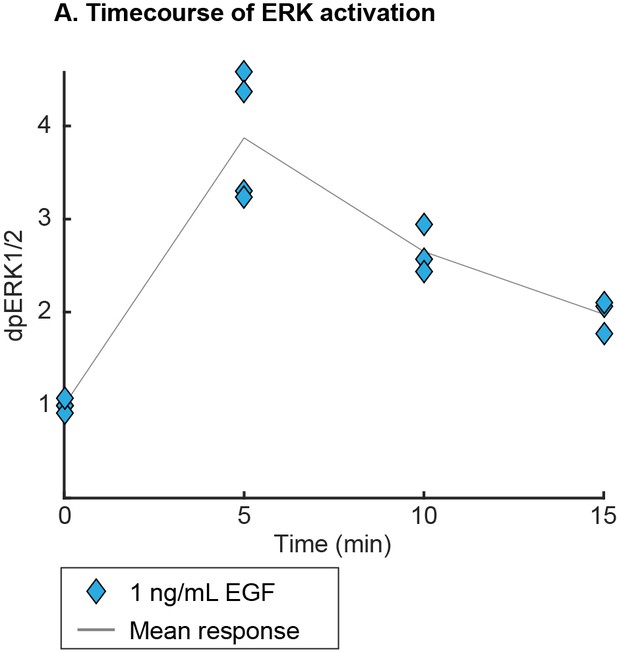
ERK activation peaks at 5 min after EGF stimulation.
H1299 cells were treated with 1 ng/mL EGF for the specified times, and then assayed for dpERK1/2 by Western blot. Data is plotted relative to the samples at time zero, with at least three biological replicates per time point.
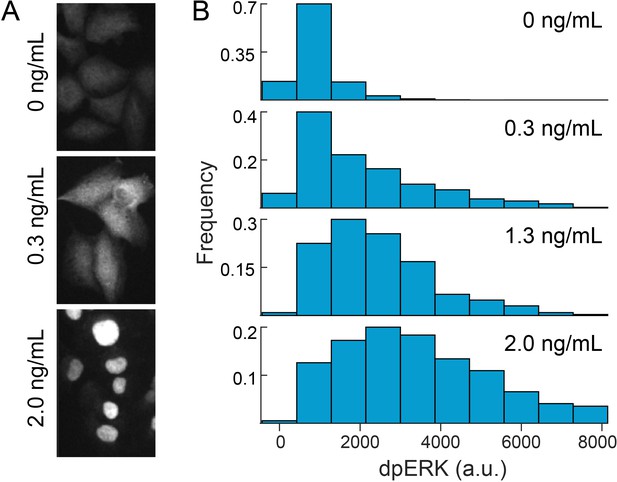
Single-cell immunofluorescence measurements show graded ERK response to EGF.
In these experiments, H1299 cells were treated with varying doses of EGF for 5 min, and then fixed and analyzed for immunofluorescence against doubly phosphorylated ERK (dpERK).
(A) Representative images of cells treated with the indicated doses of EGF. (B) The intensity of nuclear level of dpERK staining across individual cells. Cell nuclei were delineated using DAPI staining (for EGF doses 0, 0.3, 1.3, and 2.0 ng/mL, N = 453, 381, 373, and 413 cells, respectively).
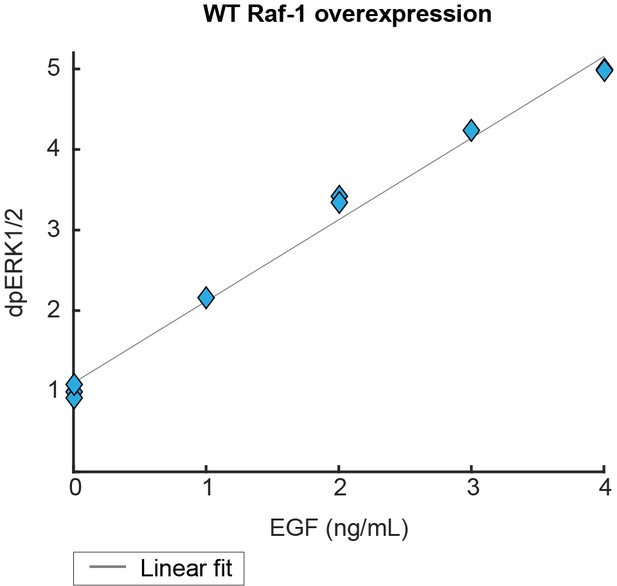
WT Raf-1 overexpression does not affect linear dose-response.
H1299 cells over-expressing Raf-1 were treated with the indicated dose of EGF for 5 min, and then assayed for dpERK1/2 by Western blot. The grey line is a fit from a linear model with r2 = 0.99. Data is plotted relative to unstimulated samples, with total N = 9.
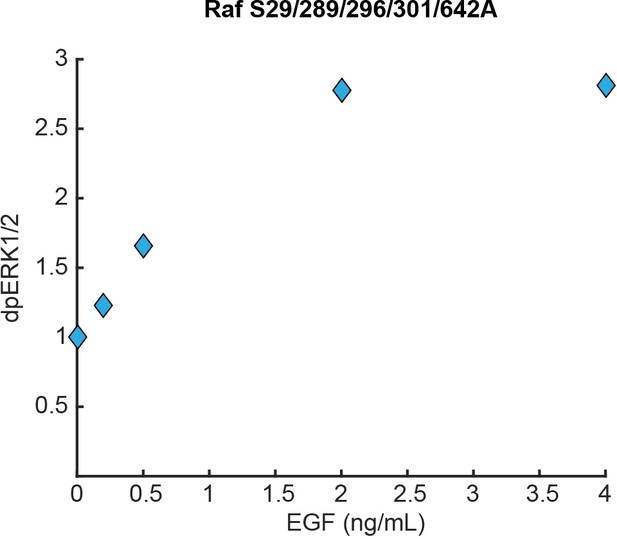
Expression of Raf S29/289/296/301/642A induces non-linear dose-response.
H1299 cells expressing the Raf mutant Raf S29/289/296/301/642 were treated with the indicated dose of EGF for 5 min, and then assayed for dpERK1/2 by Western blot. Data is plotted relative to unstimulated samples, with total N = 5.
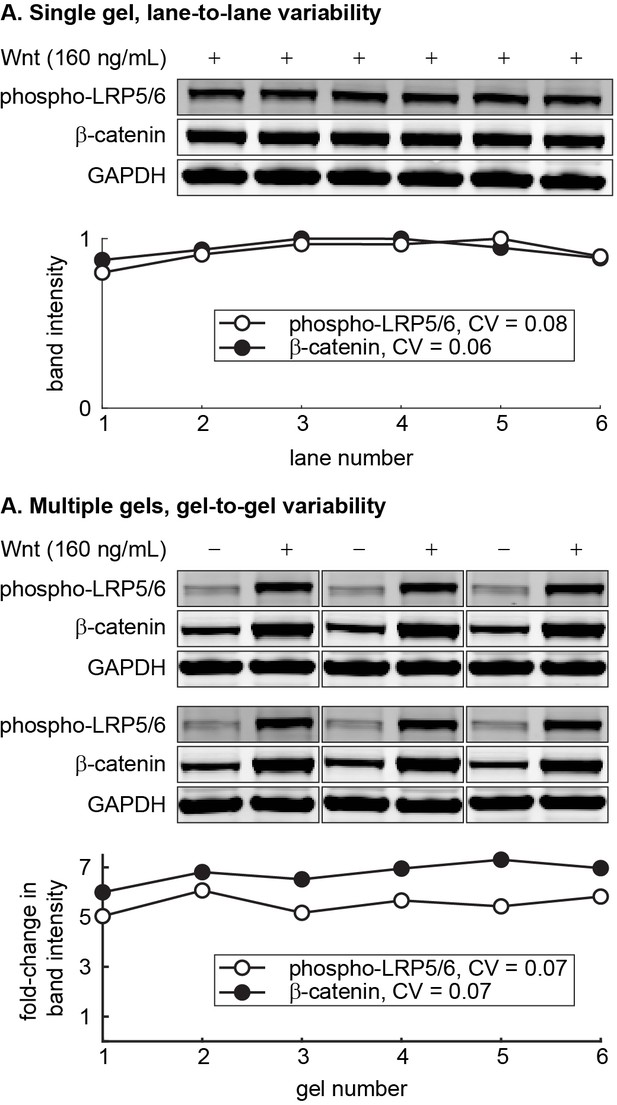
Technical variability from Western blot.
(A) The level of β-catenin and phosphorylated LRP, measured across different lanes. (B) Ligand-stimulated change in β-catenin and phophorylated LRP level, measured in six independent Western blots. CV is coefficient of variation, defined as standard deviation/mean.
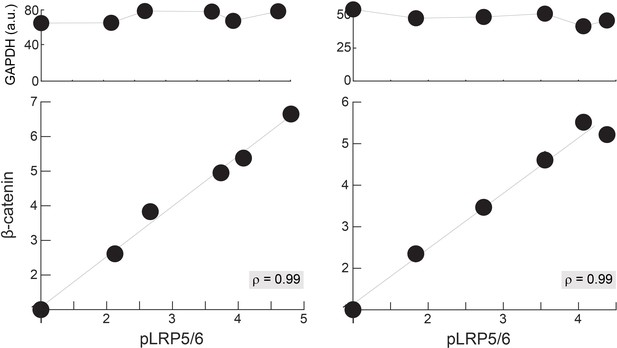
Linearity is not an artifact of loading control normalization.
In these two independent experiments, RKO cells were stimulated with a range of Wnt3A dose (0-160 ng/mL), the cells lysed after 6 hr, and analyzed for Western blot against β-catenin and phosphorylated LRP5/6 (pLRP5/6).
Top row: In each experiment, GAPDH intensity varies with <10% CV across samples. Bottom row: Raw β-catenin and LRP intensity data without normalization with GAPDH loading control. The measurements are plotted relative to unstimulated cells. Grey lines are least squares regression lines, and is the Pearson correlation coefficient.
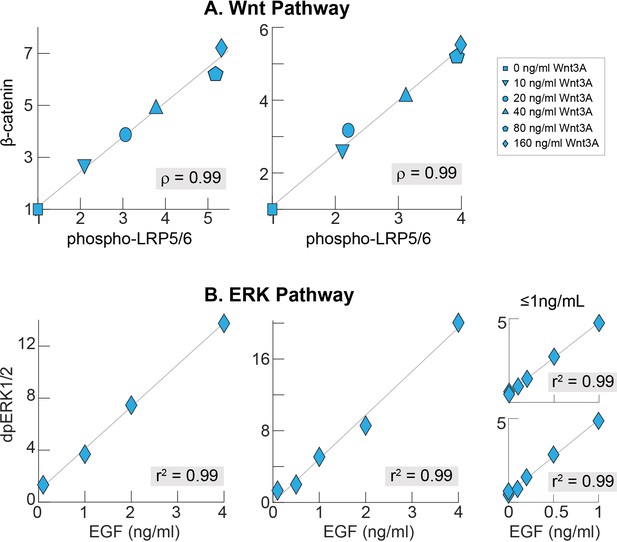
Linearity was observed across independent experiments.
(A) In these two independent experiments, RKO cells were stimulated with a range of Wnt3A doses, lysed after 6 hr, and analyzed for Western blot against β-catenin and phospho-LRP5/6. (B) In these four independent experiments, H1299 cells were stimulated with a range of EGF doses, lysed after 5 min, and analyzed for Western blot against doubly-phosphorylated ERK. All measurements are plotted relative to unstimulated cells. Grey lines are least squares regression, ρ is Pearson correlation coefficient, and r2 is correlation coefficient.
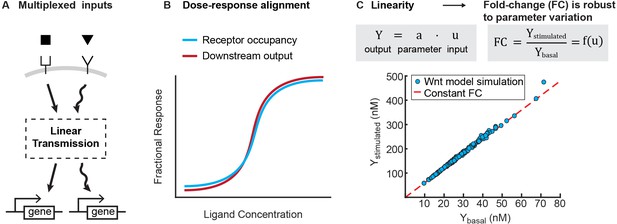
Benefits of linearity.
(A) Linearity enables multiplexing of inputs to a signaling pathway. Multiplexed signals can be independently decoded downstream, and therefore regulate distinct transcriptional events. (B) Illustration for how linearity between the receptor occupancy and downstream outputs gives rise to dose-response alignment (Andrews et al., 2016). (C) Linearity can produce fold-changes in output that are robust to variation in cellular parameters. To illustrate this, we added lognormal noise (0.1 CV) to all parameters of the Wnt model, and simulated the level of β-catenin before and after Wnt stimulation (blue circles). As long as the model operates in the regime of linear signal transmission (i.e. , where is output, is input, and is a scalar that is a function of parameters), variation in parameters affects stimulated and basal level of β-catenin equally, and we get a constant fold change in β-catenin (i.e. red line, where is independent of parameter variations).
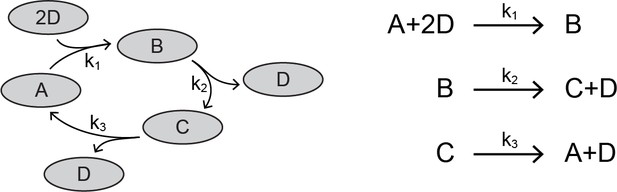
Network of four proteins.
https://doi.org/10.7554/eLife.33617.026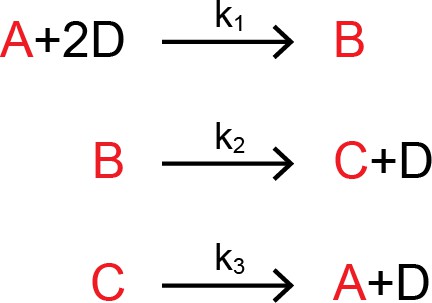
Reaction set corresponding to protein network.
https://doi.org/10.7554/eLife.33617.027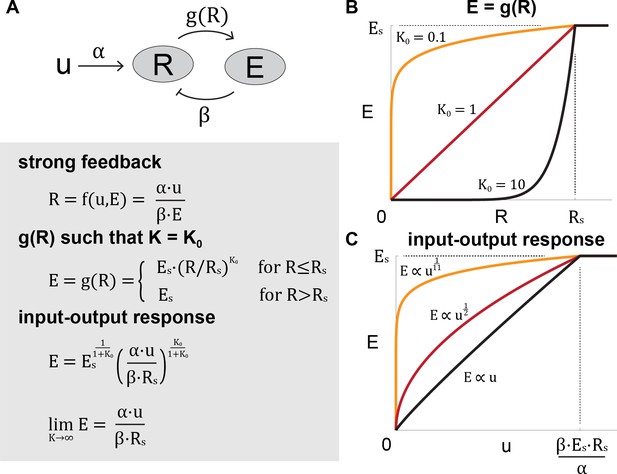
Toy model of the ERK pathway.
https://doi.org/10.7554/eLife.33617.028Tables
Parameters, variables, and equations of the Wnt model.
https://doi.org/10.7554/eLife.33617.029Parameter | Label | Value | |
---|---|---|---|
Activation rate of Disheveled/Dvl by Wnt | |||
Inactivation rate of Dvl | |||
Dissociation of destruction complex (DC) by active Dvl | |||
Phosphorylation of DC | |||
Dephosphorylation of DC | |||
Forward rate for DC binding | |||
Reverse rate for DC binding | |||
Dissociation constant for APC:axin binding | |||
Dissociation constant for β-catenin:DC binding | |||
Phosphorylation rate of β-catenin | |||
Rate of phosphorylated β-catenin release from DC | |||
Degradation rate of phosphorylated β-catenin | |||
Synthesis rate of β-catenin | |||
Degradation rate of β-catenin | |||
Synthesis rate of axin | |||
Degradation rate of axin | |||
Dissociation constant for β-catenin:TCF binding | |||
Dissociation constant for β-catenin:APC binding | |||
Total concentration of Disheveled | |||
Total concentration of adenomatous polyposis coli | |||
Total concentration of T-cell factor | |||
Total concentration of glycogen synthase kinase 3β | |||
Independent Variable | Label | ||
Active Disheveled | |||
APC*/axin*/GSK3 (* denotes phosphorylated) | |||
APC/axin/GSK3 | |||
β-catenin*/APC*/axin*/GSK3 | |||
β-catenin* | |||
β-catenin | |||
axin | |||
Dependent Variable | Label | ||
Inactive Disheveled | |||
GSK3 | |||
APC/axin | |||
APC | |||
β-catenin/APC*/axin*/GSK3 | |||
TCF | |||
β-catenin/TCF | |||
β-catenin/APC | |||
Differential Equations | |||
Equations for fast equilibrium reactions | |||
Parameters, variables, and equations of the ERK model.
Values highlighted in yellow have been changed from the original model (explained in section ‘ERK Model’).
Parameter | Label | Value | |
---|---|---|---|
Forward rate for Raf:RasGTP binding | |||
Reverse rate for Raf:RasGTP binding | |||
Phosphorylation rate for Raf by RasGTP | |||
Forward rate of pRaf:P1 binding | |||
Reverse rate of pRaf:P1 binding | |||
Dephosphorylation rate of pRaf by P1 | |||
Forward rate of MEK:pRaf binding | |||
Reverse rate of MEK:pRaf binding | |||
Phosphorylation rate of MEK by pRaf | |||
Forward rate of pMEK:pRaf binding | |||
Reverse rate of pMEK:pRaf binding | |||
Phosphorylation rate of pMEK by pRaf | |||
Forward rate of dpMEK:P2 binding | |||
Reverse rate of dpMEK:P2 binding | |||
Dephosphorylation rate of dpMEK by P2 | |||
Forward rate of pMEK:P2 binding | |||
Reverse rate of pMEK:P2 binding | |||
Dephosphorylation rate of pMEK by P2 | |||
Forward rate of ERK:dpMEK binding | |||
Reverse rate of ERK:dpMEK binding | |||
Phosphorylation rate of ERK by dpMEK | |||
Forward rate of pERK:dpMEK binding | |||
Reverse rate of pERK:dpMEK binding | |||
Phosphorylation rate of pERK by dpMEK | |||
Forward rate of pERK:P3 binding | |||
Reverse rate of pERK:P3 binding | |||
Dephosphorylation rate of pERK by P3 | |||
Forward rate of dpERK:P3 binding | |||
Reverse rate of dpERK:P3 binding | |||
Dephosphorylation rate of dpERK by P3 | |||
Forward rate of Raf:dpERK binding | |||
Reverse rate of Raf:dpERK binding | |||
Hyper-phosphorylation rate of Raf by ppERK | |||
Forward rate of pRaf:dpERK binding | |||
Reverse rate of pRaf:dpERK binding | |||
Hyper-phosphorylation rate of phosphorylated Raf by dpERK | |||
Forward rate of Rafi:P4 binding | |||
Reverse rate of Rafi:P4 binding | |||
Dephosphorylation rate of Rafi by P4 | |||
Total Raf | |||
Total MEK | |||
Total ERK | |||
Total phosphatase P1 | |||
Total phosphatase P2 | |||
Total phosphatase P3 | |||
Total phosphatase P4 | |||
Variable | Label | ||
Unphosphorylated Raf | |||
Raf bound to RasGTP | |||
Phosphorylated Raf | |||
Phosphatase for phosphorylated Raf | |||
Phosphorylated Raf bound to its phosphatase | |||
Unphosphorylated MEK | |||
MEK bound to its kinase | |||
Phosphorylated MEK | |||
Phosphorylated MEK bound to its kinase | |||
Doubly-phosphorylated MEK | |||
MEK phosphatase | |||
Doubly-phosphorylated MEK bound to its phosphatase | |||
Phosphorylated MEK bound to its phosphatase | |||
Unphosphorylated ERK | |||
ERK bound to its kinase | |||
Phosphorylated ERK | |||
Phosphorylated ERK bound to its kinase | |||
Doubly-phosphorylated ERK | |||
ERK phosphatase | |||
Phosphorylated ERK bound to its phosphatase | |||
Doubly-phosphorylated ERK bound to its phosphatase | |||
Raf bound to doubly-phosphorylated ERK | |||
Hyper-phosphorylated, ‘inactive’ Raf | |||
Phosphorylated Raf bound to doubly-phosphorylated ERK | |||
Phosphatase for hyper-phosphorylated Raf | |||
Hyper-phosphorylated Raf bound to its phosphatase | |||
Differential Equations | |||
Algebraic Equations for conserved species | |||
Parameters, variables and equations of the Tgfβ model.
https://doi.org/10.7554/eLife.33617.031Parameter | Label | Value | ||
---|---|---|---|---|
Phosphorylation rate of Smad2 | ||||
Dephosphorylation rate of Smad2 | ||||
Nuclear import rate of Smad2 | ||||
Nuclear export rate of Smad2 | ||||
Nuclear import rate of Smad4 | ||||
Nuclear export rate of Smad4 | ||||
Smad complex import factor | ||||
Forward rate for Smad complex binding | ||||
Reverse rate for Smad complex binding | ||||
Cytoplasmic to nuclear volume ratio | ||||
Total Smad2 (initialized to cytoplasm) | ||||
Total Smad4 (initialized to cytoplasm) | ||||
Total phosphatase in nucleus | ||||
Total Receptors | ||||
Variable | Label | |||
Cytoplasmic Smad2 | ||||
Cytoplasmic phosphorylated Smad2 | ||||
Cytoplasmic Smad4 | ||||
Cytoplasmic Smad2:Smad4 complex | ||||
Cytoplasmic Smad2:Smad2 complex | ||||
Nuclear Smad2 | ||||
Nuclear phosphorylated Smad2 | ||||
Nuclear Smad4 | ||||
Nuclear Smad2:Smad4 complex | ||||
Nuclear Smad2:Smad2 complex | ||||
Differential Equations | ||||
Algebraic Equations for conserved species | ||||
Examples of biological systems where the Wnt, ERK, and Tgfβ pathways have been shown to produce graded response in single-cell level.
https://doi.org/10.7554/eLife.33617.032Pathway | Systems where graded response has been observed | References |
---|---|---|
Live imaging of single cells | ||
Tgfβ pathway | Mouse myoblasts | Frick et al., 2017; Warmflash et al. (2012) |
Human epidermal keratinocytes | Nicolás et al. (2004); Warmflash et al. (2012) ; Schmierer et al. (2008); Vizán et al. (2013) | |
Human cervical epithelial cells | Nicolás et al. (2004) | |
Human breast epithelial cells | Strasen et al. (2018) | |
Canonical Wnt pathway | Human embryonic kidney cells | Kafri et al. (2016) (this is the only published live single-cell imaging study in the Wnt pathway so far) |
ERK pathway | Mouse fibroblasts | Toettcher et al. (2013) |
Mouse embryonic fibroblasts | Mackeigan et al. (2005) | |
Human non-small cell lung carcinoma | Cheong et al., 2011 | |
Human mammary gland cells | Selimkhanov et al. (2014); Perrett et al., 2013Perrett et al., 2013 | |
Human cervical epithelial cells | Voliotis et al. (2014); Whitehurst et al. (2004); Perrett et al., 2013Perrett et al., 2013 | |
Human foreskin fibroblasts | Whitehurst et al. (2004) | |
Immunofluorescence and FACS studies | ||
Tgfβ pathway | Xenopus embryo | Schohl and Fagotto (2002) |
Mouse testes | Itman et al. (2009) | |
Zebrafish embryo | Dubrulle et al. (2015) | |
Canonical Wnt pathway | Xenopus embryo | Schneider et al. (1996); Fagotto and Gumbiner (1994); Schohl and Fagotto (2002) |
Mouse embyo | Aulehla et al., 2008 | |
Planaria | Sureda-Gómez et al., 2016 | |
Sea anemone embryo | Wikramanayake et al., 2003 | |
ERK pathway | Chick embryo | Delfini et al. (2005) |
Xenopus embryo | Schohl and Fagotto (2002) | |
Human T lymphocyte cells | Lin et al. (2009) | |
Rat adrenal gland cells | Santos et al., 2007 |
Additional files
-
Transparent reporting form
- https://doi.org/10.7554/eLife.33617.024