Biophysics of object segmentation in a collision-detecting neuron
Figures
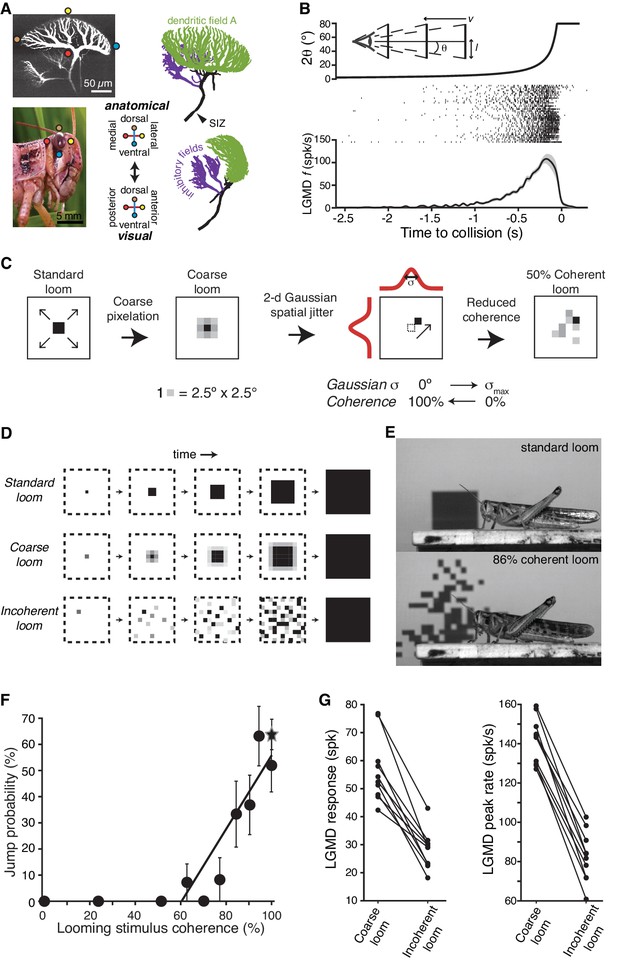
LGMD responses and escape behavior are sharply tuned to the spatial coherence of looming stimuli.
(A) LGMD 2-photon scan (top, left), eye close-up of Schistocerca americana (bottom, left), rostral, and lateral view of a LGMD reconstruction used for modeling (top and bottom right). Excitatory dendritic field in green, SIZ: spike initiation zone. Colored dots illustrate the retinotopic mapping of excitatory inputs to the LGMD. (B) Top, schematic of visual stimulus, half-size l, approach speed v, half-angular subtense at the eye, θ. Note the non-linear increase in angular subtense (2θ), characteristic of looming stimuli. Middle, spike rasters of the LGMD responses to looming stimuli. Bottom, mean instantaneous firing rate (f) of LGMD looming response. Shaded area is ±1 sem. (C) The coherence of looming stimuli was altered by first applying a coarse pixelation to create photoreceptor sized pixels. Then, a zero-mean random shift was added to the position of these coarse pixels to generate the reduced coherence stimuli. The standard deviation of the random shifting (in degrees) determined the reduction in coherence, with σmax = 40° for electrophysiological experiments and σmax = 80° for behavioral ones (Materials and methods). (D) Illustration of coherent and incoherent stimuli. For coarse looms (middle row) grayscale levels are set so that luminance in each coarse pixel is equal to that of standard looms in every frame. For reduced coherence looms (bottom), the spatial locations of the coarse pixels were altered. (E) Video frames from presentation of standard looming (top) and 86% coherent (bottom) stimuli. (F) Jump probability increased sharply with stimulus coherence above 50% (r = 0.91, p=5.9·10−4), 202 trials from 66 animals. Circles are data from coarse and reduced spatial coherence stimuli; star shows response to standard looms. (G) The LGMD's spike count (p=2.5·10−4, Wilcoxon rank sum, WRS) and peak firing rate (p=1.9·10−4, WRS) were lower for 0% coherent than 100% coherent looming stimuli (N = 10).
-
Figure 1—source code 1
A Matlab script that will import the data in Figure 1—source data 1 and generate the plots in Figure 1.
To run the script requires auxiliary matlab files 'linear_plotter.m', 'lin_Fit.m', 'ErrorPlot.m, 'ErrorBars.m', and 'isvect.m'.
- https://doi.org/10.7554/eLife.34238.004
-
Figure 1—source data 1
An.xlsx spreadsheet with source data plotted in Figure 1.
Associated code for generating plots is in the Matlab file Figure1_SourceCode1.m.
- https://doi.org/10.7554/eLife.34238.005
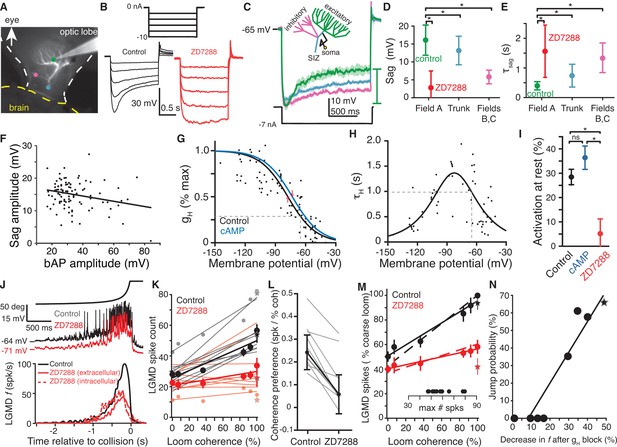
HCN channels in dendritic field A are responsible for spatial coherence sensitivity.
(A) Image showing the LGMD stained in vivo with Alexa 594 and the recording electrode with tip at the green dot. Colored dots indicate the recording locations of traces shown in (C). (B) Hyperpolarizing current steps (top) injected in field A generate a characteristic rectifying sag in control recordings (left), but application of ZD7288 completely removed the sag (right). (C) Schematic of the LGMD’s dendritic subfields and example traces showing larger rectifying sag in field A than in field C or near the SIZ. Solid lines are the average response with shaded region of ±1 sd. Sag amplitude was measured as the amount of rectification from peak hyperpolarization to steady state, as indicated by the green bar. (D) Sag amplitudes following steps from rest yielding peak hyperpolarizations between −95 and −115 mV are consistently larger in field A (N = 82,58) than after ZD7288 application (N = 13,9) or in recordings from the trunk (N = 11,13) or inhibitory subfields (N = 6,6; *: p<0.05, KW-MC). (E) The sag time constant for these responses was smaller in field A (N = 82,58) than after ZD7288 application (N = 13,9) or in recordings from the trunk (N = 11,13) or inhibitory subfields (N = 6,6; *: p<0.01, KW-MC). For (D, E), points are median and error bars are one mad. (F) Sag amplitude along the trunk and in field A decreased with increased backpropagating action potential (bAP) amplitude, a measure of electrotonic distance from the spike initiation zone (r = −0.25, p=0.01, N = 104,69). (G) Activation curve of gH measured in voltage clamp. Black line is control (N = 8,7; v1/2 = −77.6 mV, 28% of max at RMP, dashed line; R2 = 0.69) and blue line after local application of cAMP (N = 6,6; v1/2 = −73.4 mV, 35% of max at RMP). Red arrows indicate shift in v1/2. (H) Time constant of gH from voltage clamp recordings (N = 8,7; τHmax = 1.34 s, at −83 mV; τH = 985 ms at RMP, dashed line; steepness = 20 mV; R2 = 0.61). (I) Resting activation of HCN channels, relative to max, displayed as mean and sem (control N = 82,58; cAMP N = 6,6; ZD7288 N = 13,10; *: p<0.001, ns: p=0.076, unpaired t-test). (J) Intracellular recordings of LGMD’s membrane potential in response to looming stimuli show decreased RMP and activation after blockade of gH (top). Bottom, mean instantaneous firing rates (f) in response to looming stimuli declined after intra- or extra-cellular application of ZD7288 (N = 10,10 p=4.1·10−5, WRS). (K) Each line shows the linear fit to the LGMD response of an animal before (black) and after (red) puffing ZD7288 (N = 10). Half-tone dots show representative data from one animal for the corresponding fit line. Thick lines and dots are population averages. Stars are standard loom. The slope of control data was higher than after gH block (p=0.001), but the intercepts were not different (p=0.18; ANCOVA, N = 10,10). (L) For all experiments coherence preference decreased after gH blockade (N = 10, gray lines). Coherence preference was calculated as the increase in spike count per percent increase in stimulus coherence. Black dots and lines show the average coherence preference decreased by 0.18 spikes per percent stimulus coherence (p=7.9·10−5, paired t-test). (M) After gH block the slope of coherence-dependent increase was reduced from 0.45 to 0.16 (p=3.7·10−4, ANCOVA test of slopes, N = 10,10). Solid lines and dots are coarse loom data, stars are standard loom, error bars are ±1 sem, and dashed lines are compartmental simulation results. Insets show plot normalization values. (N) Jump probability for a stimulus correlates strongly with its gH-dependent increase in firing (r = 0.94, p=4.1·10−4). Circles are data from coarse and reduced spatial coherence stimuli; star shows response to standard looms. N: number of recordings, number of animals.
-
Figure 2—source code 1
A Matlab script that will import the data in Figure 2—source data 1 and generate the plots in Figure 2.
- https://doi.org/10.7554/eLife.34238.012
-
Figure 2—source code 2
A Matlab script that will import the data in Figure 2—source data 2 and generate the plots in Figure 2—figure supplement 1.
To run the script requires auxiliary matlab files 'ErrorBars.m' and 'isvect.m'.
- https://doi.org/10.7554/eLife.34238.013
-
Figure 2—source code 3
A Matlab script that will import the data in Figure 2—source data 3 and generate the plots in Figure 2—figure supplement 2.
To run the script requires auxiliary matlab files 'linear_plotter.m', 'lin_Fit.m', 'ErrorBars.m', and 'isvect.m'.
- https://doi.org/10.7554/eLife.34238.014
-
Figure 2—source data 1
An.xlsx spreadsheet with data plotted in Figure 2.
Associated code for generating plots is in the Matlab file Figure2_SourceCode1.m.
- https://doi.org/10.7554/eLife.34238.015
-
Figure 2—source data 2
An.xlsx spreadsheet with source data plotted in Figure 2—figure supplement 1.
Associated code for generating plots is in the Matlab file Figure2_SourceCode2.m.
- https://doi.org/10.7554/eLife.34238.016
-
Figure 2—source data 3
An.xlsx spreadsheet with source data plotted in Figure 2—figure supplement 2.
Code for generating plots is in the Matlab file Figure2_SourceCode3.m.
- https://doi.org/10.7554/eLife.34238.017
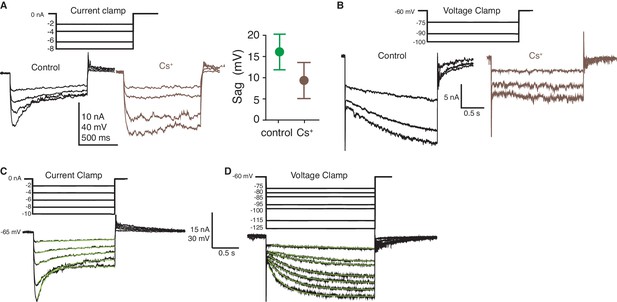
ZD7288 and Cs+ block gH within the LGMD.
(A) Cs+ blocked the rectifying sag generated by HCN channels. At left, example traces showing increased input resistance and decreased sag after intracellular Cs+ application. At right, the sag amplitude decreased after Cs+ (p=1.2·10−5; WRS). Plotted as in Figure 2D. (B) Cs+ blocked the inward current generated by gH as illustrated with hyperpolarizing voltage steps. (C) Example current clamp steps used to characterize the rectifying sag generated by HCN channels. (D) Example voltage clamp steps used to characterize the activation function of HCN channels. In (C), (D) the green lines overlaying the traces are the single exponential fits to the data used to calculate sag amplitude and time constant as well as channel activation and time constant (see Materials and methods). The traces within each panel (A, B) are from a single animal, as are those in (C), (D).
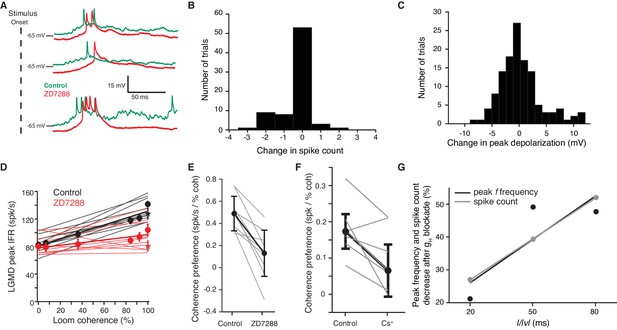
Effects of gH on local or looming stimuli assessed with block by ZD7288 or Cs+.
(A) Responses to 1° ON flashes at three different visual locations before and after addition of ZD7288. (B, C) Histograms of change in response to 1° flashes from control to gH blockade. On average, (B), spike count decreased slightly by 0.3 spikes per stimulus (p<0.01) and, (C), peak membrane depolarization slightly increased by 0.2 mV (p=0.52 N = 4,4). (D) LGMD's peak firing rate increased with looming stimulus coherence in control. After blocking gH with ZD7288 this increase was reduced (p=5.8·10−5 test of slopes, p=0.82 test of y-intercept; ANCOVA). (E) Coherence preference calculated from peak firing rates decreased for all 10 animals tested (N = 10, p=2.5·10−4, paired t-test). (F) Blockade with Cs+ decreased the coherence preference of the LGMD (N = 7, p=0.026, paired t-test). (G) Responses to faster looming stimuli (smaller l/|v|), were less sensitive to gH blockade than slower stimuli (N = 5 animals for l/|v = 50 ms, N = 3 for 20 and 80 ms).
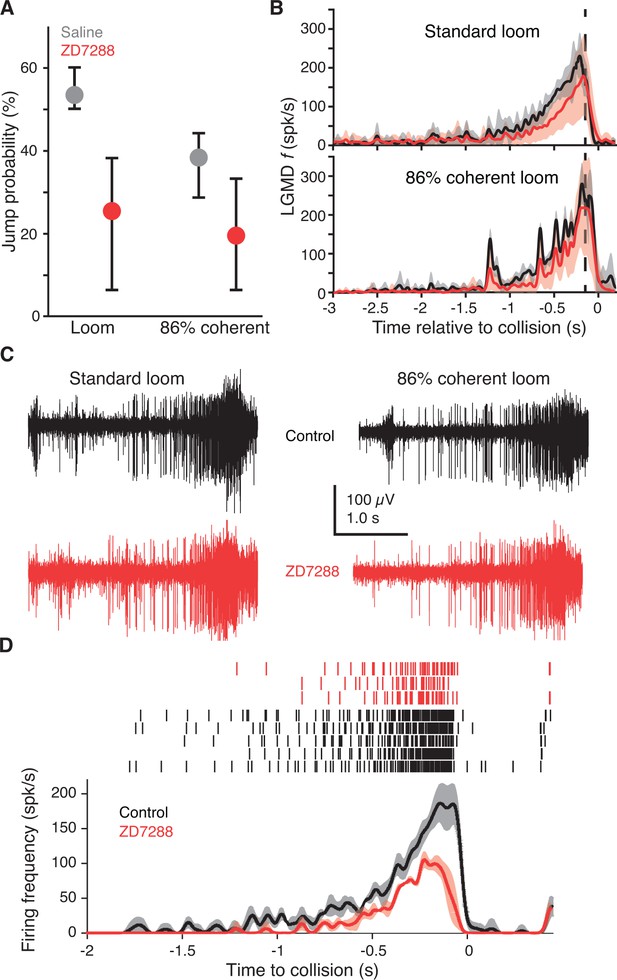
Blocking HCN channels removed coherence preference of escape behavior.
(A) Jump probability for coherent looming stimuli decreased after injecting ZD7288 into the lobula, compared to saline injection (p=0.008, ASL). Error bars are bootstrapped 95% confidence intervals. Responses to 86% coherent stimuli after ZD7288 injection were not significantly different from responses after saline injection (p=0.08, ASL) or standard looming responses after ZD7288 injection (p=0.34, ASL). Saline injection: 48 trials from five animals, ZD7288 injection: 41 trials from five animals. (B) LGMD instantaneous firing rates (f) during jump experiments decreased after ZD7288 injection. ZD7288 decreased responses to both stimuli (p=0.019 for loom, p=0.015 for 86% coherent, WRS; N = 3). Vertical dashed lines show the average time of jump. (C) Example extracellular recordings during jump experiments before and after ZD7288 application. The control responses displayed have 182 and 165 spikes, and responses after ZD7288 have 128 and 122 spikes for the standard and 86% coherent loom, respectively. (D) Rasters and instantaneous firing rates after stereotactic injection of ZD7288 through the eye in restrained animals; LGMD responses were reduced similarly to intracellular and visually guided application (Figure 2J), demonstrating that the stereotactic injection method successfully targeted the LGMD.
-
Figure 3—source code 1
A Matlab script that will import the data in Figure 3—source data 1 and generate the plots in Figure 3.
To run the script requires auxiliary matlab files 'ErrorPlot.m', 'ErrorBars.m' and 'isvect.m'.
- https://doi.org/10.7554/eLife.34238.020
-
Figure 3—source code 2
A Matlab script that will import the data in Figure 3—source data 2 and generate the plots in Figure 3—figure supplement 1.
To run the script requires auxiliary matlab files 'ErrorBars.m' and 'isvect.m'.
- https://doi.org/10.7554/eLife.34238.021
-
Figure 3—source data 1
An.xlsx spreadsheet with data plotted in Figure 3.
- https://doi.org/10.7554/eLife.34238.022
-
Figure 3—source data 2
An.xlsx spreadsheet with source data plotted in Figure 3—figure supplement 1.
- https://doi.org/10.7554/eLife.34238.023
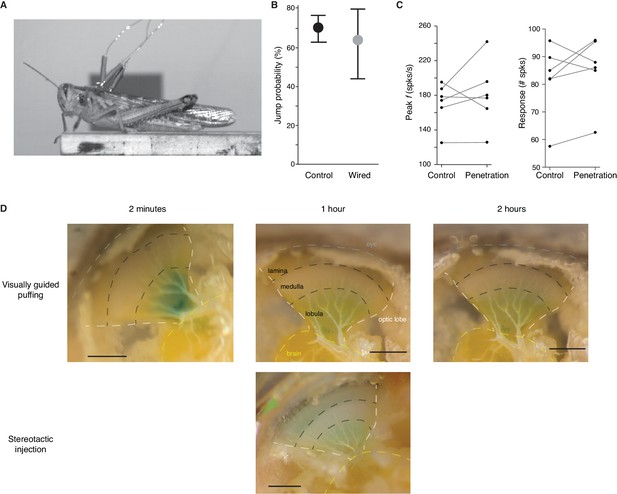
Change in escape behavior due to gH blockade within the LGMD.
(A) Video frame from presentation of standard looming stimulus showing the wires used to record LGMD activity in jumping animals. (B) Jump probability, displayed as mean and 95% confidence intervals, was not significantly reduced by the surgery to implant the electrodes or attaching the wires (p=0.74 ASL, N = 11 for control, N = 5 for wired). (C) LGMD responses were not reduced by the injection procedure. Lines show the response of six individual animals before and after pipette penetration through the eye. On average, a 5% increase in peak firing rates (p=0.56) and a 7% increase in spike count (p=0.44) occurred after penetration (Wilcoxon Sign Rank test). (D) Comparison between visually guided drug application and the stereotactic injection method used for ZD7288 application during behavioral testing. Shortly after puffing, the dye spreads along the surface of the lobula (left). As the dye spreads into the tissue the color fades but remains within the lobula (middle, top). In the second hour, little additional spreading is seen (right). Below, for stereotactic injection, the pipette was inserted through the eye (green arrow) and lowered to the lobula. Postmortem dissection an hour after injection revealed staining throughout the lobula. Dashed lines indicate boundaries between different anatomical regions. The white lines indicate the edge of the optic lobe and the yellow line outlines the brain. The two black lines show the approximate boundaries between the lamina and medulla and medulla and lobula. Scale bars in images are 0.5 mm.
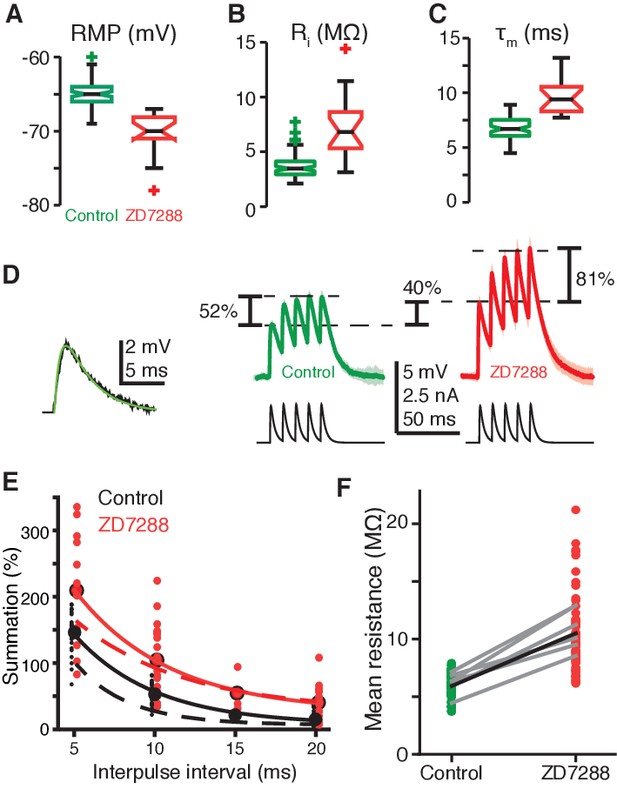
gH conductance decreases EPSP amplitudes and summation.
(A) RMP in field A decreased after blockade of gH by ZD7288 (control N = 82,58; ZD7288 N = 15,9; p=1.9·10−9, WRS). (B) Input resistance (Ri) in field A increased after gH blockade (control N = 78,58; ZD7288 N = 17,10; p=6.1·10−7, WRS). (C) Membrane time constant (τm) also increased in field A after gH block (control N = 82,58; ZD7288 N = 16,10; p=1.3·10−8, WRS). (D) Left, example visual EPSP (black) and sEPSP (green). Right, example responses to a series of 5 sEPSPs with 10 ms interpulse interval. On average ZD7288 block of gH led to 51% increase in 1st sEPSP amplitude (p=6.0·10−4, WRS) and subsequent summation of sEPSPs from 53% in control to 104% (p=0.001, WRS; control N = 14,7; ZD7288 N = 9,5). (E) Summation increased after gH removal for all interpulse intervals. Points and solid lines show experimental data, dashed lines are simulation results. (F) The integrated membrane potential (Vm) normalized by the integrated current (total charge) increased an average of 77% after ZD7288 (thick black line; p=1.7·10−19, WRS, N = 14,7 in control and 9,5 in ZD7288). Gray lines are from six recordings held through puffing (p≤0.01, paired t-test).
-
Figure 4—source code 1
A Matlab script that will import the data in Figure 4—source data 1 and generate the plots in Figure 4.
- https://doi.org/10.7554/eLife.34238.025
-
Figure 4—source data 1
An.xlsx spreadsheet with data plotted in Figure 4.
- https://doi.org/10.7554/eLife.34238.026
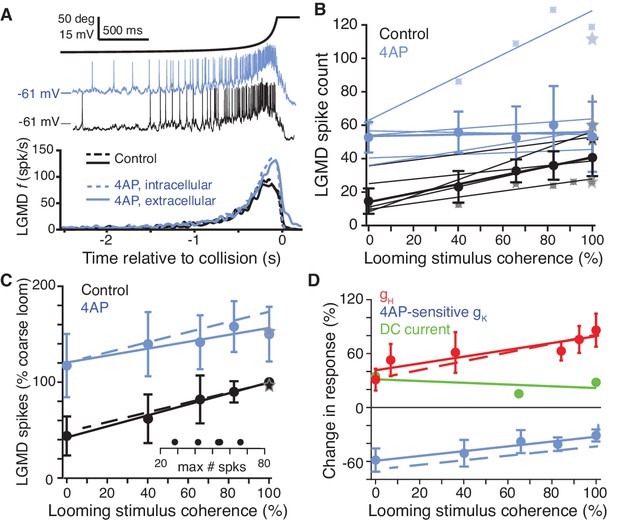
A 4AP-sensitive K+conductance decreases responses to incoherent stimuli.
(A) The time course of the looming stimulus is indicated on top by its subtended visual angle (2θ, see Figure 1B) and (middle) example recordings of LGMD membrane potential in response to looming stimuli before and after local puff of 4AP. Below, average firing rate of the LGMD before and after application of 4AP. Both intracellular and extracellular application had the same effect on looming responses (N = 5,5 for extracellular, N = 3,3 for intracellular). (B) Individual linear fits (control: r = 0.93 ± 0.04; 4AP r = 0.64 ± 0.48, mean ±sd) to the LGMD responses before and after puffing 4AP show an increase in firing for all animals, and a decrease in coherence preference for all but one animal. Data points for the top and bottom fit are shown for example. Thicker lines show linear fits to the median response (error bars are ± mad). (C) 4AP increased average responses to all stimuli (p=0.002; WRS), and reduced the coherence-dependent increase in firing from 0.57 to 0.36 (p=0.014, ANCOVA test of slopes; N = 5,5). Plotted as in Figure 2M. Dashed lines in C) and D) are simulation data. (D) To estimate the influence of HCN and 4AP-sensitive K+ channels on firing we calculated the percent change after channel blockade relative to control (channels present). gH increased responses, with larger increase for coherent stimuli. The K+ channels decreased responses with larger decreases for incoherent stimuli. As comparison, tonic −2.5 nA current injection resulted in smaller effects, with percent change independent of stimulus coherence (p=0.36, KW).
-
Figure 5—source code 1
A Matlab script that will import the data in Figure 5—source data 1 and generate the plots in Figure 5.
To run the script requires auxiliary matlab files 'linear_plotter.m', 'lin_Fit.m', 'ErrorBars.m' and 'isvect.m'.
- https://doi.org/10.7554/eLife.34238.029
-
Figure 5—source code 2
A Matlab script that will import the data in Figure 5—source data 2 and generate the plots in Figure 5—figure supplement 1.
To run the script requires auxiliary matlab files 'linear_plotter.m', 'lin_Fit.m', 'ErrorBars.m' and 'isvect.m'.
- https://doi.org/10.7554/eLife.34238.030
-
Figure 5—source data 1
An.xlsx spreadsheet with data plotted in Figure 5.
- https://doi.org/10.7554/eLife.34238.031
-
Figure 5—source data 2
An.xlsx spreadsheet with source data plotted in Figure 5—figure supplement 1.
- https://doi.org/10.7554/eLife.34238.032
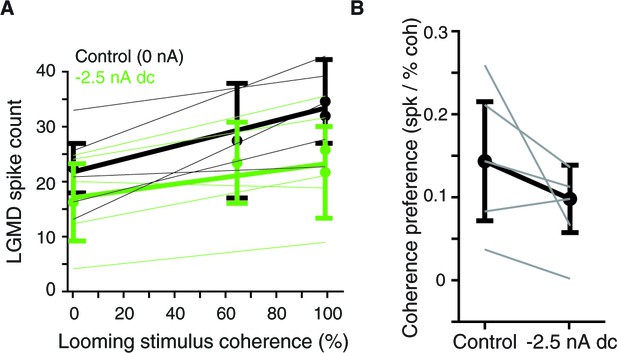
Effect of hyperpolarizing current injection on coherence preference.
(A) LGMD spike counts with and without a –2.5 nA holding current as a function of stimulus coherence. Thin lines are fits to responses of individual animals (N = 5), thick lines and dots are averages (mean ±sd). (B) The hyperpolarizing current reduced responses for all animals and reduced coherence preference in 3 of 5 animals. Mean reduction was 0.08 spikes per percent coherence (p=0.10, paired t-test).
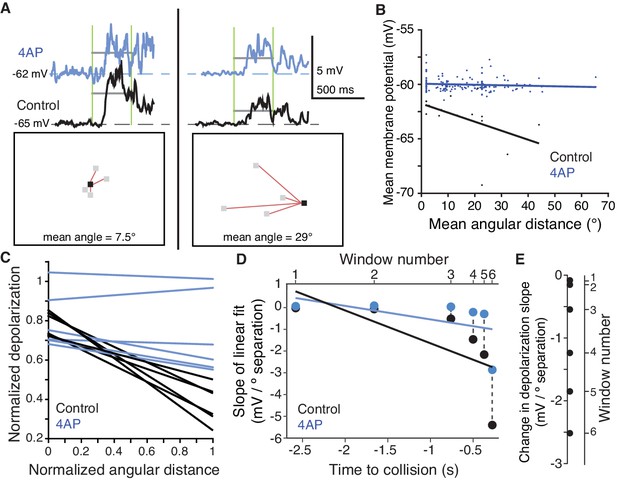
A 4AP-sensitive K+conductance reduces dendritic depolarization for spatially incoherent inputs.
(A) Example of the first of six time periods in which the mean membrane potential and mean angular distance were measured. Top, traces of the dendritic membrane potential recorded within field A. At left are responses to higher spatial coherence stimuli, at right are responses to lower spatial coherence stimuli. Black traces are control and blue traces are after 4AP application. Vertical green bars mark the time period measured; horizontal grey bars show the mean Vm during the time period. Bottom, example stimulus frames taken within the first time period. Red lines are drawn between newly changing coarse pixels (grey) and the only previously darkened one (black). (B) Under control conditions, an inverse relationship is seen, with more spatially dispersed stimuli generating less membrane depolarization. Application of 4AP removed this effect (p=0.0002, ANCOVA test of slopes). (C) Membrane potential changes showed a strong negative correlation with distance between stimulated regions in control (r = −0.54, p=4.1·10−8), but 4AP application significantly reduced this effect (p=0.006, ANCOVA test of slopes, N = 7,7). Each line pair is from a different time period of the stimulus. (D) The reduction in depolarization of distant inputs is shown with a point from each time window. Although the range of distances decreases as the stimulus nears collision (p=1.2·10−6, t-test), the reduction in depolarization per degree of separation increases (p=1.7·10−10, KW). (E) Within each time period, the 4AP-sensitive current caused a decrease in response to spatially distant inputs (p=0.03, signed rank, N = 7,7).
-
Figure 6—source code 1
A Matlab script that will import the data in Figure 6—source data 1 and generate the plots in Figure 6.
To run the script requires auxiliary matlab files 'linear_plotter.m' and 'lin_Fit.m'.
- https://doi.org/10.7554/eLife.34238.035
-
Figure 6—source code 2
A Matlab script that will import the data in Figure 6—source data 2 and generate the plots in Figure 6—figure supplement 1.
To run the script requires auxiliary matlab files 'linear_plotter.m' and 'lin_Fit.m'.
- https://doi.org/10.7554/eLife.34238.036
-
Figure 6—source data 1
An.xlsx spreadsheet with data plotted in Figure 6.
- https://doi.org/10.7554/eLife.34238.037
-
Figure 6—source data 2
Acsv file with source data plotted in Figure 6—figure supplement 1.
- https://doi.org/10.7554/eLife.34238.038
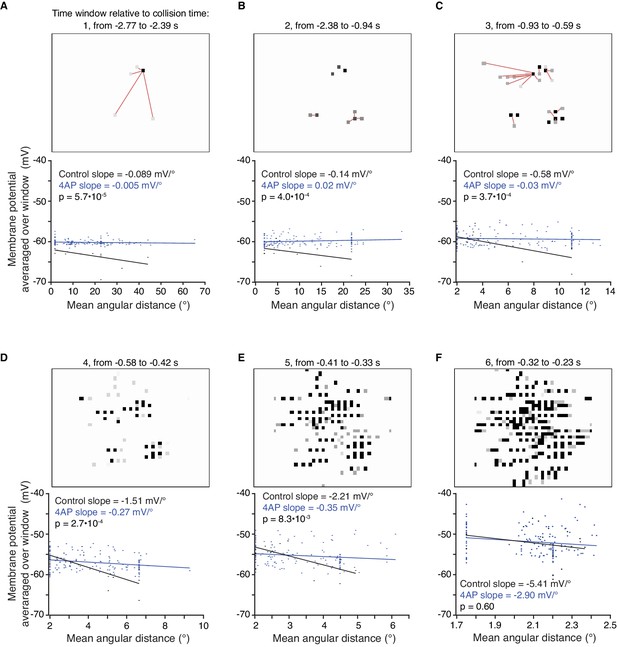
Relationship between mean stimulus angular distance and membrane potential before and after application of 4AP.
(A–F) The mean angular distance between newly appearing coarse pixels and earlier changing ones was measured within six time periods (see Materials and methods). For each time period, an example stimulus frame from within that period is shown above the relationship between angular distance and membrane potential from within the same period. For the first three periods, red lines are drawn between newly changing coarse pixels (grey) and the closest earlier changing one (black). Under control conditions, an inverse relationship is seen, with more spatially dispersed stimuli generating less membrane depolarization. Application of 4AP removed this effect for the first five periods (p<0.01 ANCOVA test of slopes). Near the end of the stimulus there is little separation between newly appearing and earlier changing pixels, as illustrated by the small range of angular distances on the abscissa of the bottom right panel. Accordingly, the slopes in control and 4AP were not significantly different (p=0.60; l/|v| = 50 ms; N = 7,7).
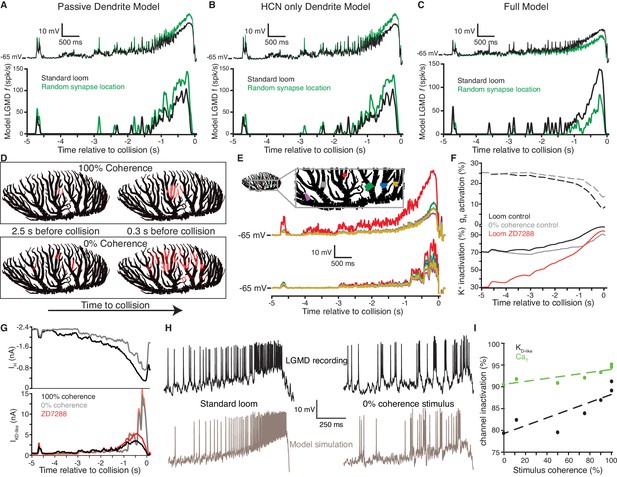
An active biophysical model reproduces the preference for coherent synaptic inputs.
(A) A model LGMD with realistic morphology and passive dendrites generated a stronger response to spatially randomized inputs (green) than retinotopically arranged ones (black). Spatially randomizing inputs increased mean membrane potential 1.23 ± 0.01 mV at the base of field A (top) and increased firing by 59% (bottom). (B) Adding HCN channels to the dendrites did not change this trend. Spatially random inputs (green) increased mean membrane potential 1.23 ± 0.01 mV at the base of field A (top) compared to spatially coherent ones (black) and increased firing by 64% (bottom). (C) The full model, with both HCN and inactivating K+ channels, however, had 1.25 ± 0.01 mV lower membrane potential at the base of field A and 61% less spiking in response to spatially random inputs. (D). Images of field A illustrating the branches receiving synaptic inputs. Brighter red indicates stronger inputs. During looming stimuli, excitation spreads slowly from a single location due to retinotopy (top). Spatially incoherent stimuli produce inputs spread over a much larger dendritic region. (E) The simulated membrane potential was measured at several dendritic locations indicated in the image at the top (traces below are color coded by location). For a coherent loom, a much larger depolarization occurs on dendritic branches receiving prolonged excitation. Incoherent stimuli generate a similar level of depolarization across the dendritic arbor. (F) At bottom, the time course of K+ channel inactivation shows higher inactivation during a coherent looming stimulus (black) than an incoherent coarse stimulus (gray). After blocking HCN channels (red), the resting inactivation is much less and never reaches the inactivation level of control. At top, HCN channel activation is lower during coherent stimuli since K+ channel inactivation leads to increased depolarization. (G) The time course of the HCN and KD-like total membrane currents during 100% and 0% coherence stimuli. IH decreases throughout both stimuli, but IKD-like increases more during incoherent stimuli. Red lines show the increased KD-like currents after HCN block. (H) Comparison of the membrane potential near the base of field A for experimental data (top) and model simulation (bottom) reveal a steady ramp up in firing rate in response to coherent looming stimuli and a burstier firing pattern in response to a 0% coherence coarse looming stimulus. (I) The mean channel inactivation during the last 2 s before collision increased with stimulus coherence for both K+ and CaT channels (with slopes of 0.094 and 0.030, respectively).
-
Figure 7—source code 1
A Matlab script that will import the data in Figure 7—source data 1 and generate the plots in Figure 7.
To run the script requires auxiliary matlab files 'linear_plotter.m' and 'lin_Fit.m'.
- https://doi.org/10.7554/eLife.34238.041
-
Figure 7—source data 1
An.xlsx spreadsheet with data plotted in Figure 7.
Rich media files.
- https://doi.org/10.7554/eLife.34238.042
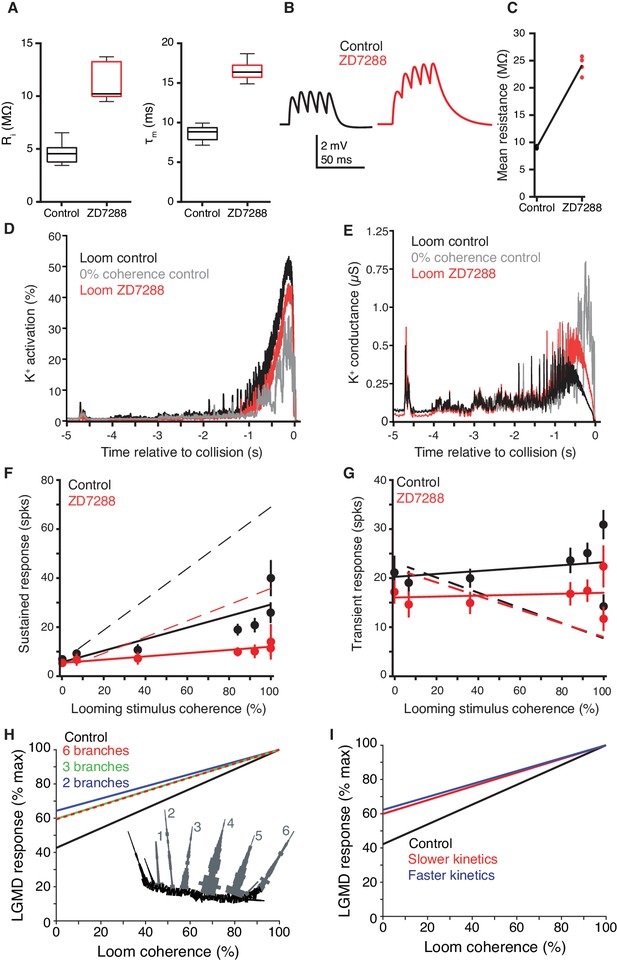
Additional comparisons of the LGMD model with experimental data.
(A) In model simulations, input resistance (Ri) and membrane time constant (τm) were increased by gH removal (compare to Figures 4B,C). (B) Simulated LGMD model sEPSP amplitude and summation increased after gH removal. Example traces from NEURON simulation shown have an interpulse interval of 10 ms (compare to Figure 4D) (C) Model LGMD simulation showed normalized, integrated responses to sEPSP currents comparable to experimental data (Figure 4F). (D) The time course of K+ channel activation shows higher activation during a coherent looming stimulus (black) than an incoherent coarse stimulus (gray). After blocking HCN channels (red), the resting activation is less and never reaches the activation level of control. (E) The time course of K+ channel conductance (the product of activation and inactivation) shows lowest conductance during a coherent looming stimulus (black) and highest for an incoherent coarse stimulus (gray). After blocking HCN channels (red), the resting conductance is less but surpasses that of the control for looming stimuli. (F) Sustained firing increased with stimulus coherence in control conditions more than after gH block (p=5.8·10−7, ANCOVA test of slopes). (G) Transient or burst firing, however, showed no gH-dependent coherence increase (p=0.53, ANCOVA test of slopes). In F, G) N = 10,10 for solid lines and dots; dashed lines from model simulations. (H) Reducing the number of dendritic branches, by compressing them into electrotonically equivalent cylinders decreased coherence selectivity. The branch number refers to the number of equivalent cylinders receiving synaptic inputs during the looming stimuli. The reduced morphology of field A, shown in the lower inset, had synaptic inputs impinge across six equivalent branches (grey, 1–6). Compartments in black did not receive synaptic inputs. The 2 and 3 branch morphologies were further reduced by combining 2 or 3 of these equivalent cylinders. (I) A ten-fold increase or decrease in the kinetics of HCN activation and KD-like inactivation both reduced the coherence selectivity.
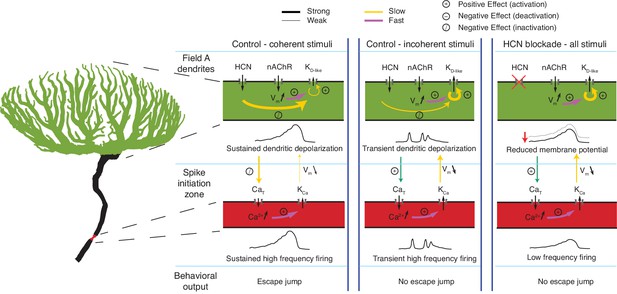
Schematic illustration of the effects of HCN channels on responses to looming stimuli.
Arrows indicate current flow, changes in Ca2+ or membrane potential, and the strongest interaction between channels, dendrite and SIZ, during looming stimulus detection. For all visual stimuli, excitatory nicotinic acetylcholine receptors (nAChR) produce a fast activation of KD-like. In control conditions during coherent stimuli (left), the addition of gH on top of nAChR activation produces sustained depolarization which slowly inactivates KD-like channels within field A (green) and CaT channels at the SIZ (red). This results in reduced KD-like and KCa conductances and sustained high frequency firing. In control conditions during incoherent stimuli (middle), the increased dendritic area of nAChR activation increases the number of KD-like channels activated. The resulting increase in the hyperpolarizing KD-like conductance prevents the gH-dependent inactivation of KD-like channels, producing only transient depolarization. The transient depolarization initiates CaT driven bursts and subsequent KCa conductance activation. This prevents the sustained high-frequency firing necessary for initiating escape. After gH blockade (right), the resting membrane potential is reduced, increasing the activatable KD-like and CaT channels. Without gH, depolarization cannot be sustained high enough to inactivate these channels leading to an increased KD-like and KCa conductances and lower frequency firing which fails to produce escape behaviors.
Videos
Looming stimulus with synchronized LGMD membrane potential.
At top is a standard looming stimulus with an l/|v| value of 50 ms. Beneath is the recorded membrane potential of a LGMD neuron during presentation of this stimulus. The vertical blue bar marks the current time of the stimulus. The last burst of activity is caused by the removal of the final black square (not shown in movie). In an experiment, the stimulus appears smoother due to the 200 frame/s refresh rate of the video monitor instead of the 30 frames/s shown here.
Spatially incoherent coarse looming stimulus with synchronized LGMD membrane potential.
At top is a 0% coherence coarse looming stimulus with an l/|v| value of 50 ms and 2° coarse pixels. Beneath is the recorded membrane potential of a LGMD neuron during presentation of this stimulus. The vertical blue bar marks the current time of the stimulus. The last burst of activity is caused by the removal of the final black square (not shown in movie).
Escape jump from a looming stimulus.
Video of an escape jump from a standard looming stimulus with an l/|v| value of 80 ms. Video was recorded at 200 frames/s and is slowed to 60 frames/s. The animal had received a control saline injection in the optic lobe of the right eye.
Additional files
-
Source code 1
ErrorBars.m matlab file.
- https://doi.org/10.7554/eLife.34238.044
-
Source code 2
ErrorPlot.m matlab file.
- https://doi.org/10.7554/eLife.34238.045
-
Source code 3
Isvect.m matlab file.
- https://doi.org/10.7554/eLife.34238.046
-
Source code 4
lin_Fit.m matlab file.
- https://doi.org/10.7554/eLife.34238.047
-
Source code 5
linear_plotter.m matlab file.
- https://doi.org/10.7554/eLife.34238.048
-
Supplementary file 1
Statistics table.
A table including additional details on the statistical analyses presented in the Results and Figures.
- https://doi.org/10.7554/eLife.34238.049
-
Transparent reporting form
- https://doi.org/10.7554/eLife.34238.050