Functionally asymmetric motor neurons contribute to coordinating locomotion of Caenorhabditis elegans
Figures
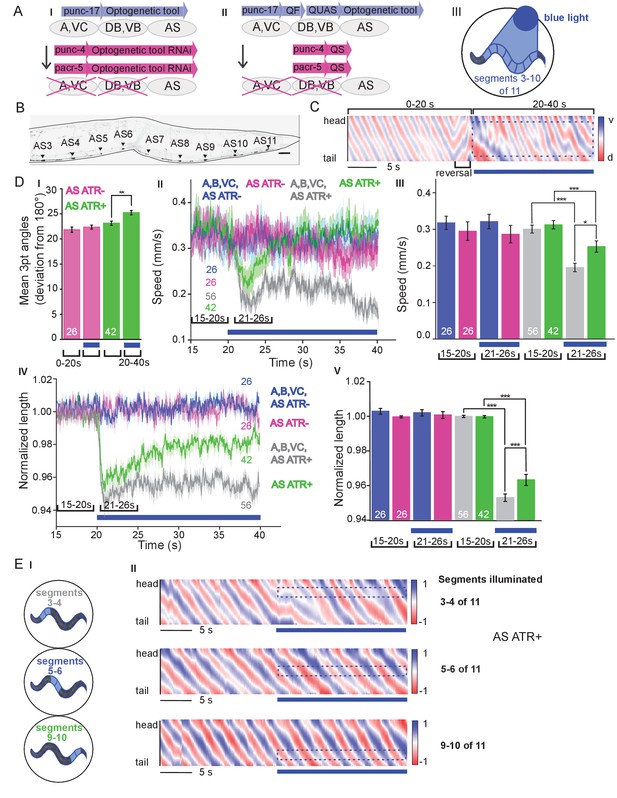
Specific photodepolarization of AS MNs via ChR2 leads to body contraction, increased bending angles and reduced speed in freely moving C. elegans.
(A) ‘Subtractive’ expression and illumination strategy to achieve specific stimulation of AS MNs by optogenetic tools: (I) Silencing of optogenetic tool protein expression in the non-target subsets of MNs by dsRNA; (II) Using the Q system for conditional expression. The transcriptional activator QF binds to the QUAS sequence to induce optogenetic tool expression. The transcriptional inhibitor QS suppresses expression in unwanted cells by binding to QF; (III) Selective illumination of the VNC MNs by 470 nm blue light. The body of the worm was divided into 11 segments, of which 3 – 10 were illuminated in animals moving freely on agar plates. (B) Expression pattern of ChR2(H134R)::YFP in AS MNs by the dsRNA subtractive approach; scale bar, 20 µm. See also Figure 1—figure supplement 1. (C) Representative body postures kymograph (20 s) of normalized 2-point angles of a 100-point spine, calculated from head to tail of the animal. Positive and negative curvature is represented by blue and red color. Animal expressed ChR2 in AS MNs as in AI and was illuminated after 10 s as in AIII. Blue bar, period of 470 nm illumination. (D) Photodepolarization of AS MNs by ChR2 (in animals raised with ATR): I) Analysis of mean bending angles, before and during the blue light illumination period (as in C). (II, III) Locomotion speed: Mean ±SEM crawling speed of animals before and during blue illumination (blue bar), comparing animals expressing ChR2 in AS MNs or in all types of cholinergic MNs in the VNC, raised in the presence or absence of ATR (III: Group data of mean speed of the animals before (15–20 s) and during (21–26 s) ChR2 photoactivation); IV, (V) Mean ±SEM body length of the animals shown in I, II (V: Group data of the mean length before (15–20 s) and during (21–26 s) photoactivation). (E) Depolarization of subsets of AS MNs in body segments. (I) Scheme of anterior, midbody and posterior segmental illumination; II) Representative body posture kymographs of 2-point angles from head to tail before (20 s) and during ChR2 photoactivation by blue light in the segments of the worm body, corresponding to experiments as in E I). See also Figure 1—video 1 and Figure 1—figure supplement 2. P values *≤0.05; **≤0.01; ***≤0.001. Number of animals is indicated in D. Statistical test in D III and V: ANOVA with Tukey’s post hoc test.
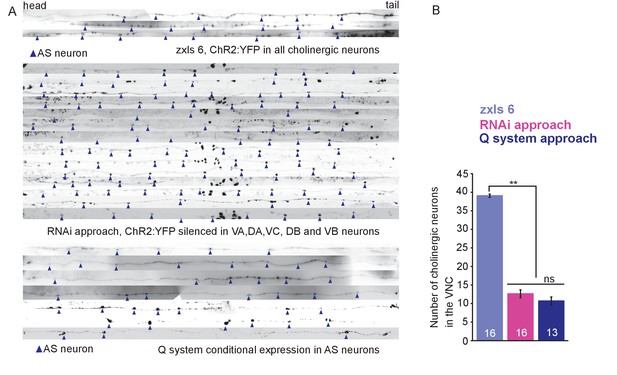
Expression of ChR2 can be restricted to AS MNs.
(A) Expression of ChR2::YFP in the VNC MNs, either in all cholinergic neurons (upper panel), or restricted in AS MNs using the RNAi approach (middle panel), or the Q system for conditional expression (lower panel). Arrow heads point to AS MNs. Note that in the top panel, many more MN cell bodies are visible in addition to the AS MNs. Animals were photographed, then the VNCs were cut from the resulting composite images, virtually straightened and aligned at the vulva. (B) Quantification of the visible MNs in the VNC for each of the three expression approaches. P value **≤0.01. Number of animals is indicated. Statistical test: ANOVA with Tukey’s post hoc test.
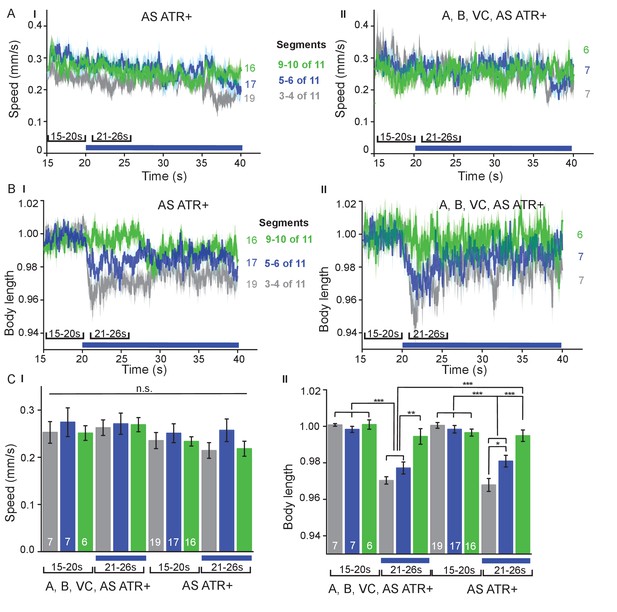
Local AS neuron activation affects body length.
(A I, II) C I) Speed and (B I, II) (C II) body length (time traces, A, B, and group data, C) before and during photodepolarization of AS MNs or of all cholinergic MNs in the anterior, middle and posterior segments of the worm body by ChR2 (in animals raised with ATR). P value *≤0.05, **≤0.01, ***≤0.001; number of animals is indicated. Statistical test: ANOVA with Tukey’s post-hoc test.
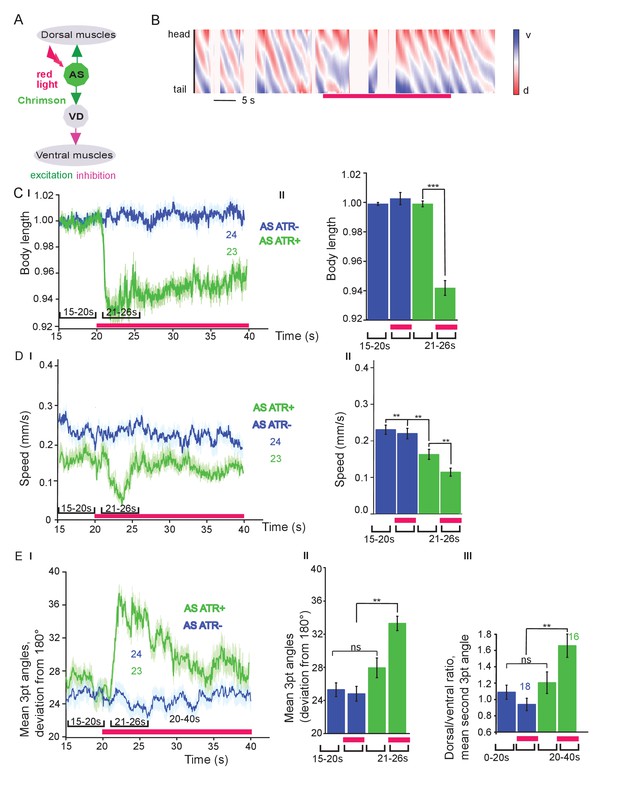
Specific photodepolarization of AS MNs via Chrimson leads to body contraction, increased bending angles and reduced speed in freely moving C. elegans.
(A) AS MNs expressing Chrimson were illuminated by 650 nm red light. (B) Representative body postures kymograph (40 s) of normalized 2-point angles of a 100-point spine, calculated from head to tail of the animal. Dorsal and ventral curvature is represented by blue and red color. The animal expressing Chrimson in AS MNs was illuminated after 20 s. Red bar, period of 650 nm illumination. Interruptions in the analysis are due to inability of the analysis script to assign periods of strong body bends when anterior and posterior body touched. (C) Photodepolarization of AS MNs by Chrimson (in animals raised with ATR): I, II) Body length and group data (II) of mean length of the animals before (15–20 s) and during (21–26 s) Chrimson photoactivation. (D) I, II) Locomotion speed: Mean ±SEM crawling speed of animals before and during blue illumination (red bar), comparing animals expressing Chrimson in AS MNs, raised in the presence or absence of ATR, and group data (II) of mean speed of the animals before (15–20 s) and during (21–26 s) Chrimson photoactivation. (E) I) Mean (±SEM) time traces of all 3-point bending angles before and during red illumination (red bar; animals raised with and without ATR). II) Group data as in I, comparing 5 s red light illumination (red bar), to the 5 s before illumination. III) Mean (±SEM) ratio of dorsal to ventral bending at the 2nd 3-point bending angle in animals expressing AS::Chrimson during photostimulation (animals raised with and without ATR). See also Figure 1—video 2. P values **≤0.01; ***≤0.001. Number of animals is indicated. Statistical test: ANOVA with Tukey’s post hoc test.
Freely moving animal before and during photodepolarization of AS MNs by ChR2 (in animal raised with ATR), blue light = 470 nm, 1.8 mW/mm2.
Video plays at 3x speed (75 f/s).
Freely moving animal before and during photodepolarization of AS MNs by Chrimson (in animal raised with ATR), red light = 650 nm, 1.8 mW/mm2.
Video plays at 3x speed (75 f/s).
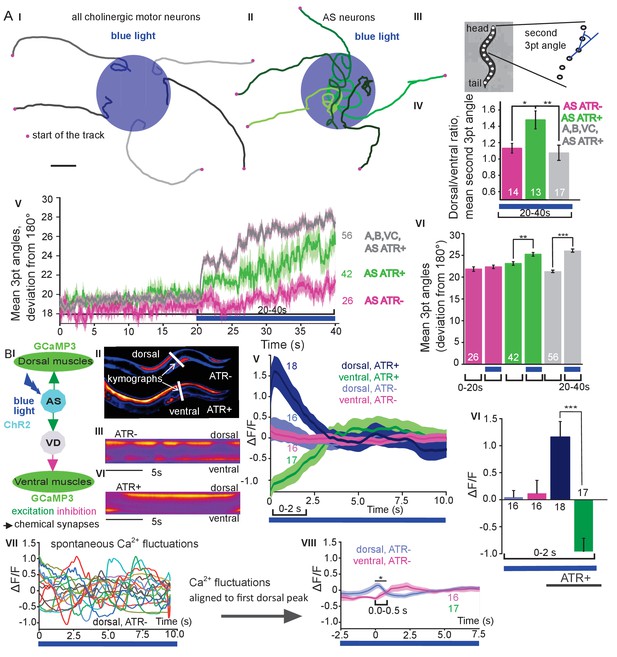
Photodepolarization of AS MNs causes transient activation of dorsal and simultaneous inhibition of ventral BWMs, and a dorsal bias in freely crawling animals.
A) (I, II) Representative locomotion tracks of freely moving animals (raised with ATR) with ChR2 expressed in all cholinergic MNs (I) or only in AS MNs (II) before (20 s) and during photostimulation (20 s) by 470 nm blue light (indicated by blue shaded area; tracks are aligned such that they cross the blue area at the time of light onset). (III) Schematic showing the thirteen points defining eleven 3-point angles along the spine of the animal. (IV) Mean (±SEM) ratio of dorsal to ventral bending at the 2nd 3-point bending angle in animals expressing AS::ChR2 or ChR2 in all VNC cholinergic neurons during photostimulation (animals raised with and without ATR). (V) Mean (±SEM) time traces of all 3-point bending angles (absolute values) before and during blue illumination (blue bar; ChR2 in AS MNs or in all cholinergic MNs; raised with and without ATR). (VI) Group data of experiments in (V), comparing 20 s blue light illumination (blue bar), to the 20 s before illumination. (B) I) AS MNs expressing ChR2 are illuminated by 470 nm blue light, Ca2+ signal is recorded in the BWM expressing GCaMP3 (arrows, chemical synapses). (II) Representative snapshots of Ca2+ signals in BWM cells during blue light illumination in animals cultivated with and without all-trans-retinal (ATR). Lines indicate regions used to generate kymographs. (III) Representative kymograph of spontaneous Ca2+ fluctuations in BWM cells of animal raised without ATR. (IV) Representative kymograph of Ca2+ signals in BWM cells in animals cultivated with ATR, in which AS MNs were photostimulated for the entire period. (V, VI) Mean Ca2+ signals (ΔF/F ± SEM) in dorsal and ventral BWM during the first 10 s of illumination (V) in animals raised with and without ATR and group data (VI), quantified during the first 2 s of illumination. (VII) Transients of spontaneous Ca2+ signals in dorsal muscles in animal without ATR. VIII) Mean Ca2+ signals (ΔF/F ± SEM) in dorsal and ventral BWM in animals raised without ATR, but aligned to the first dorsal Ca2+ spike, showing reciprocity of spontaneous dorso-ventral muscle activity. See also Figure 2—video 1. P values *≤0.05; **≤0.01; ***≤0.001; number of animals is indicated. Statistical test: ANOVA with Tukey’s post-hoc test.
Ca2+ signal in the BWM expressing GCaMP3 during photodepolarization of AS MNs by ChR2 (in animal raised with ATR), blue light = 470 nm, 1.2 mW/mm2.
Video plays at 3x speed (75 f/s).
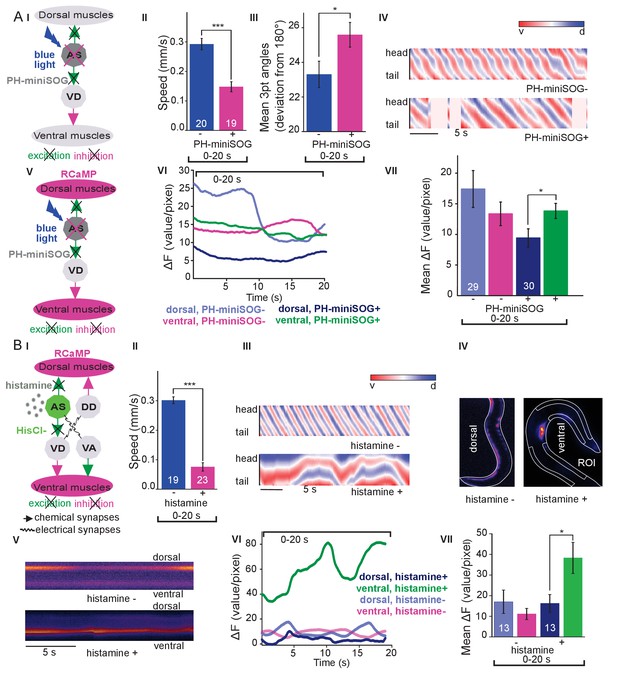
Optogenetic ablation and chronic hyperpolarization of AS MNs disrupts the locomotion pattern.
(A) I) Schematic of optogenetic ablation of AS MNs by PH-miniSOG and connectivity to relevant cell types (arrows, chemical synapses; curved lines, electrical synapses). Quantification of mean ±SEM speed (II) and bending angles (III) of animals without or with expression of PH-miniSOG (via the Q system) in AS MNs, following 150 s of blue light exposure and 2 hr resting period. IV) Representative body posture kymographs (as in Figure 1C) of wild type animal (upper panel) and animal expressing PH-miniSOG after photoactivation (lower panel). V) Schematic of Ca2+ imaging in BWM (RCaMP fluorescence) during PH-miniSOG ablation. VI) Representative transients of Ca2+ signaling in dorsal and ventral muscles after AS MN ablation by PH-miniSOG. VII) Mean Ca2+ signals (ΔF/F ± SEM) in dorsal and ventral BWM of animals without or with expression of PH-miniSOG. See also Figure 3—figure supplement 1A and Figure 3—video 1. (B) I) Schematic of Ca2+ imaging in BWM (RCaMP fluorescence) during hyperpolarization of AS MNs by HisCl1 (expressed in AS MNs via the Q system), and connectivity to relevant cell types (see also AI; note that this simplified diagram reflects cell type connectivity but does not accurately reflect connections between individual cells). (II) Mean ±SEM speed of freely moving animals on agar dishes without and with 10 mM histamine. (III) Representative body posture kymographs of animals freely moving on agar without (upper) or with 10 mM histamine (after 240 s incubation; lower panel). (IV) Representative fluorescent micrographs of Ca2+ activity in the BWM of animals mounted on agar slides without (left) or with 10 mM histamine (after 240 s incubation; right panel). (V) Representative kymographs (20 s) of Ca2+ activity in dorsal and ventral BWM of animals as in IV. (VI) Representative Ca2+ activity in dorsal and ventral BWM from animals as (shown in IV, V. VII) Mean ±SEM fluorescence of dorsal and ventral BWM as in VI. See also Figure 3—figure supplement 1B and Figure 3—video 2. P values *≤0.05, ***≤0.001; number of animals indicated in AII, VII; BII, VII. Statistics: T-test for AII, III and B II; ANOVA with Tukey’s post-hoc test for A VII and BVII.
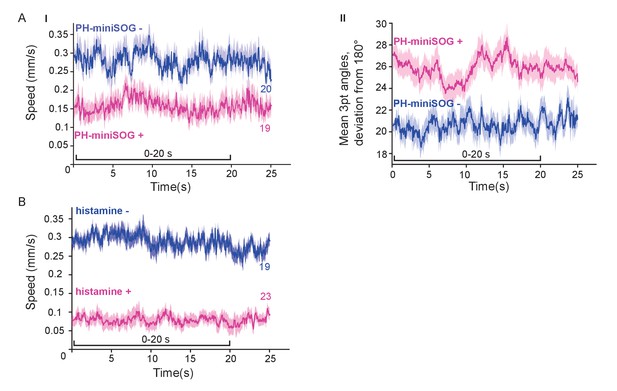
Optogenetic inactivation and HisCl1-induced hyperpolarization of AS MNs affects locomotion speed and bending angles.
(A) Time traces of mean (±SEM) speed (I) and bending angles (II) of freely moving wild type animals, or animals expressing PH-miniSOG in AS MNs, 2 hr after 150 s of blue light exposure. (B) Time traces of mean (±SEM) speed of freely moving animals expressing HisCl1in AS MNs, comparing animals on plates without and with 10 mM histamine. Number of animals tested is indicated.
Freely moving animal, 2 hr after optogenetic ablation (150 s) of AS MNs by PH-miniSOG.
Video plays at 3x speed (75 f/s).
Freely moving animal expressing HisCl1 after 4 min exposure on 10 mM histamine.
Video plays at 3x speed (75 f/s).
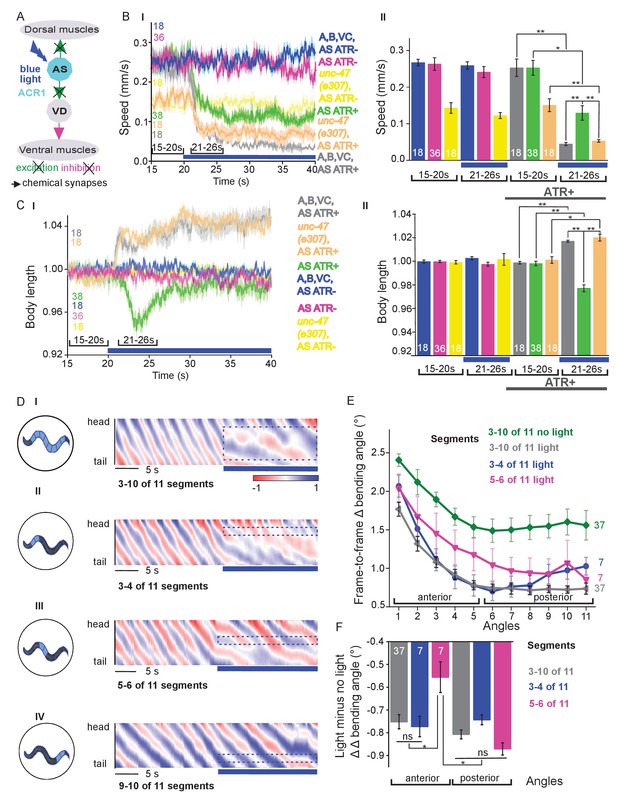
Acute optogenetic hyperpolarization of AS MNs ceases locomotion, causes disinhibition of ventral BWM via GABAergic VD MNs, and blocks propagation of the locomotion body wave.
(A) Schematic of experiment; hyperpolarization of AS MNs by the ACR1 anion channel rhodopsin activated by 470 nm blue light (arrows, chemical synapses). (B) Time traces (I) and group data quantification (II) of mean ±SEM speed before (15 – 20 s) and during (21 – 26 s) blue illumination (indicated by blue bar). Compared are strains expressing ACR1 in all VNC cholinergic neurons, or in AS MNs only (via the Q system), in wild type or unc-47(e307) mutant background, raised in the presence or absence of ATR, as indicated. (C) Time traces (I) and group data quantification (II) of mean ±SEM body length of the animals shown in B. (D) Hyperpolarization of AS MNs in all (I), in the anterior (II), middle (III) and posterior (IV) segments of the worm body. Representative body postures kymographs of normalized 2-point angles from head to tail in animal expressing ACR1 in AS MNs before and during illumination by blue light in the indicated body segments. (E) Mean, absolute difference of bending angles, from one video frame to the next (25 Hz), at each of eleven 3-point angles, for experiments as in (D). (F) Mean difference of the differential bending angles between dark and illuminated conditions, for the analyses shown in (E). Data were averaged for the anterior five or the posterior 6 3-pt bending angles. See also Figure 4—figure supplement 1 and Figure 4—videos 2-4. P values *≤0.05; **≤0.01; number of animals is indicated. Statistics: ANOVA with Tukey’s post hoc test.
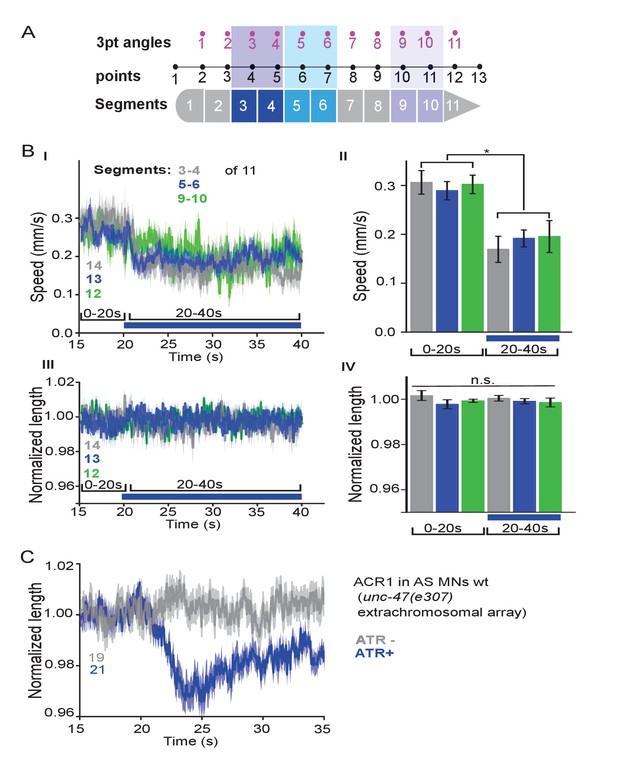
Local stimulation of AS MNs in different body segments.
(A) Schematic showing how the 13 points defining 11 3-point angles correspond to the 11 body segments that were individually illuminated. (B) Mean speed (I, II) and body length (III, IV) of animals expressing ACR1 in AS MNs, in which the indicated body segments were illuminated. Group data shown in II, IV. (C) The extrachromosomal array expressing ACR1 in the unc-47(e307) mutant as shown in main Figure 4B,C functions as expected in wt background. P value *≤0.05; number of animals is indicated. Statistical test in B: ANOVA with Tukey’s post-hoc test.
Freely moving animal expressing ACR1 in AS MNs before (20 s) and during ACR1 photoactivation by 470 nm 1.8 mW/mm2 blue light.
Video plays at 3x speed (75 f/s).
Selective illumination of anterior segment.
Freely moving animal expressing ACR1 in AS MNs before (20 s) and during ACR1 photoactivation by 470 nm 1.8 mW/mm2 blue light. Video plays at 3x speed (75 f/s).
Selective illumination of midbody segment.
Freely moving animal expressing ACR1 in AS MNs before (20 s) and during ACR1 photoactivation by 470 nm 1.8 mW/mm2 blue light. Video plays at 3x speed (75 f/s).
Selective illumination of posterior segment.
Freely moving animal expressing ACR1 in AS MNs before (20 s) and during ACR1 photoactivation by 470 nm 1.8 mW/mm2 blue light. Video plays at 3x speed (75 f/s).
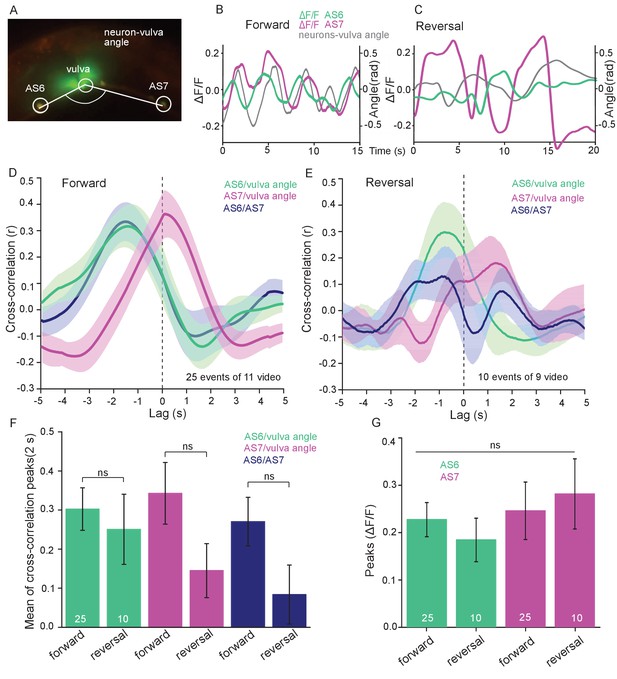
AS MNs show oscillatory Ca2+activity in moving animals.
(A) Fluorescent micrograph (merged channels) of the vulva region, showing red (mCherry) and green (GCaMP6), expressed in AS MNs (with the use of the Q system), and GFP, expressed in vulva muscles. Angle between vulva and the two flanking AS6 and AS7 neurons is indicated. (B, C) Representative analysis of time traces of Ca2+ signals (ΔF/F) in AS6 and AS7, as well as the angle defined by the vulva and the two neurons during crawling (B, 15 s, forward; C, 20 s, reverse). (D, E) Cross-correlation analysis (mean ± SEM) of single periods of the body wave (5 s each) for each of the AS6 and AS7 GCaMP6 signals with the vulva angle, as well as for the two Ca2+ signals, during forward (D) or backward (E) locomotion. (F) Comparison of the mean cross-correlation peaks (during the 2 s centered on the peak) of the fluorescence of AS6 or AS7 and the neuron/vulva angle, or between AS6 and AS7 neurons, for forward or reverse locomotion. (G) Comparison of the peak Ca2+ signals (mean ± SEM) in AS6 and AS7, during forward or reverse locomotion, respectively. See also Figure 5—video 1. Number of animals is indicated in D-G. Statistical test: ANOVA with Tukey’s post-hoc test.
Moving animal expressing GCaMP6 and mCherry in the AS MNs while the animal is being automatically tracked via the GFP marker in vulva muscles.
Video plays at 0.35x speed (7 f/s).
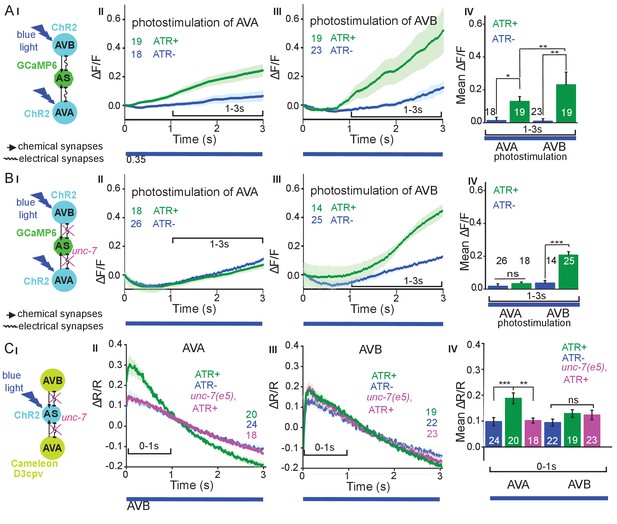
Reciprocal and asymmetric mutual activation of AS MNs and forward and reverse PINs, AVB and AVA.
(A) (I) Schematic of the experiment for measurement of AS MN Ca2+ signals (GCaMP6) during AVB or AVA photodepolarization via ChR2 with 470 nm blue illumination (arrows, chemical synapses; curved lines, electrical synapses). (II, III) Time traces of mean (±SEM) Ca2+ transients (ΔF/F) in AS MNs during depolarization of AVA (II) and AVB (III) by ChR2, in animals raised in absence or presence of ATR. Brackets indicate time periods used for statistical analysis in IV. (IV) Group data quantification of experiments shown in II and III (for the 1–3 s time period). See also Figure 6—figure supplement 1 and Figure 6—video 1, 2. (B) (I-IV), as in A (I-IV), but in the unc-7(e5) gap junction mutant background. (C) (I) Schematic of the experiment for measurement of Ca2+ signals (cameleon) in AVB or AVA PINs during AS MN photodepolarization via ChR2. (II, III) Mean (±SEM) of Ca2+ transients (ΔR/R YFP/CFP ratios) in AVA (II) and AVB (III) during AS MN depolarization, in wild type or unc-7(e5) mutant animals, raised in absence or presence of ATR. IV) Group data quantification of experiments in II and III (for the 0–1 s time period). P values *≤0.05; **≤0.01; ***≤0.001; number of animals is indicated. Statistical test: Mann-Whitney U test.
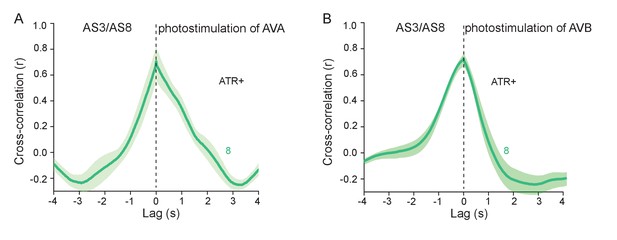
AS MNs are simultaneously activated by photostimulation of the AVA and AVB PINs.
(A,B) Cross-correlation analysis of GCaMP6 fluorescence signals (ΔF/F) in AS3 and AS8 neurons, during photodepolarization of AVA (A) or AVB (B), expressing ChR2, respectively, in animals raised in the presence of ATR.
Ca2+ signal in the AS MNs expressing GCaMP6 during photodepolarization of the AVA PIN by ChR2 (in animal raised with ATR), 470 nm blue light, 1.2 mW/mm2.
Video plays at 3x speed (75 f/s).
Ca2+signal in the AS MNs expressing GCaMP6 during photodepolarization of AVB by ChR2 (in animal raised with ATR), 470 nm blue light, 1.2 mW/mm2.
Video plays at 3x speed (75 f/s).
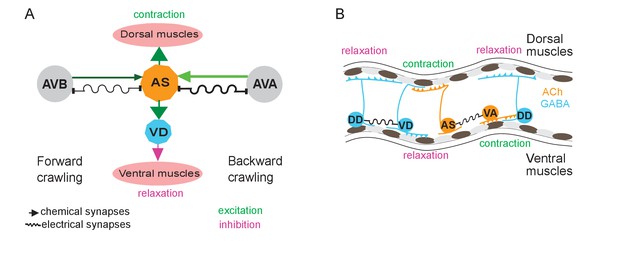
Models summarizing findings of this work.
(A) AS MNs asymmetrically regulate dorso-ventral bending during forward and backward locomotion, by excitatory chemical transmission to dorsal muscles and ventral GABAergic VD MNs, thus causing ventral inhibition. Interconnections (arrows, chemical synapses; curved lines, electrical synapses; thickness of lines indicates relative synapse number as an approximation of synaptic strength; color shade represents strength of functional connections measured in this work) of AS MNs and their other synaptic partners,that is the PINs AVB and AVA, via both chemical synapses from the PINs and (reciprocal) electrical synapses from AS MNs are also shown. Data in this work suggest (chemical) excitatory regulation of AS MNs by AVA and AVB during forward and backward locomotion, respectively, and reciprocal electrical regulation of AVA by AS MNs. (B) Interconnections and functional roles of AS MNs and other VNC MNs during the propagation of the undulatory wave along the body. Depolarization (which could be initiated by AVB, not shown here, or by proprioceptive feedback from the adjacent body segment) of AS MNs causes contraction of the dorsal BWMs and simultaneous relaxation of ventral BWMs through the excitation of VD MNs. This phase is followed by contraction of ventral BWMs, e.g. through the electric coupling of AS and VA MNs, and relaxation of the dorsal BWMs through VD-DD electrical coupling or VA-DD chemical synapses. Cholinergic (orange) and GABAergic (blue) cell types are indicated. Antero-posterior localization of cell bodies and connectivity to other cell types are arbitrary.
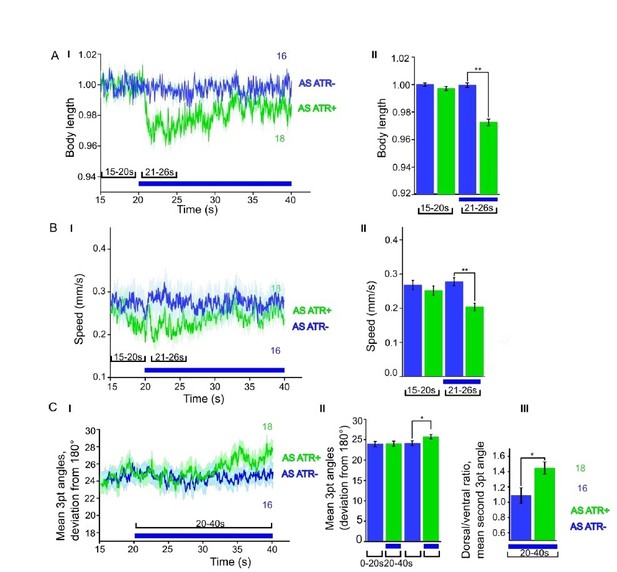
ChR2 expressed and specifically photoactivated in AS neurons pf L4 larvae.
Light-evoked effects on body length, locomotion speed and body bending angles.
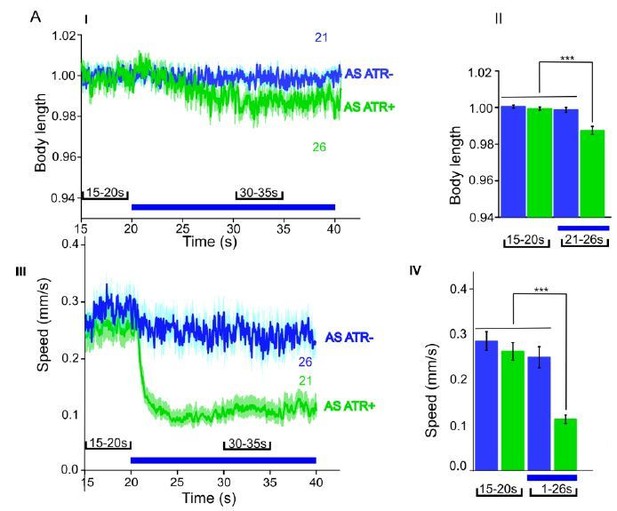
ACR1 expressed and specifically photoactivated in AS neurons of L4 larvae.
Light-evoked effects on body length and locomotion speed.
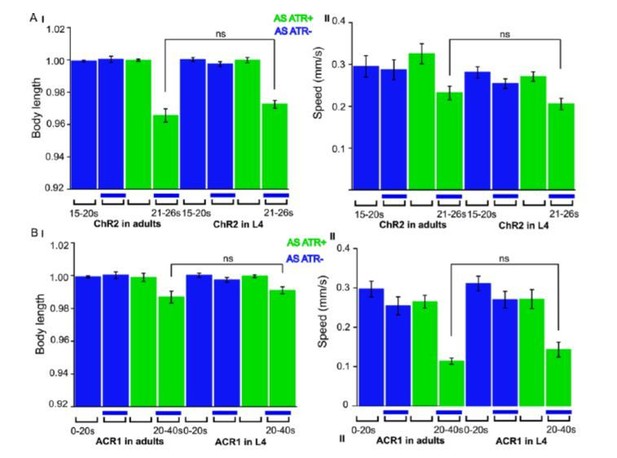
ChR2 and ACR1 expressed and specifically photoactivated in AS neurons in L4 larvae.
Light evoked effects on body length and locomotion speed. Comparisons to the same experiments performed in adult animals, as reported in the manuscript.
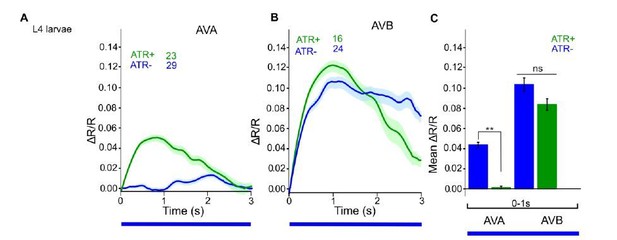
ChR2 expressed and specifically photoactivated in AS neurons of L4 larvae, imaging of Ca2+ transients in AVA or AVB neurons, using Cameleon.
Compare to Figure 6C in the manuscript (formerly 6B, data for adult animals).
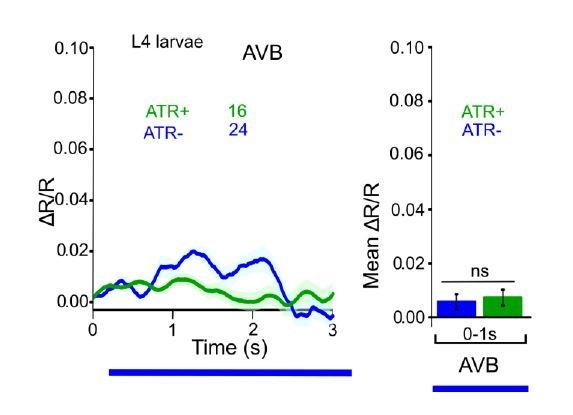
Figure R5.
ChR2 expressed and specifically photoactivated in AS neurons, imaging of Ca2+ transients in AVB neurons of L4 larvae, using Cameleon. Apparent photobleaching of CFP during the first 1-2 seconds was corrected for.
Additional files
-
Transparent reporting form
- https://doi.org/10.7554/eLife.34997.027