A terpene synthase-cytochrome P450 cluster in Dictyostelium discoideum produces a novel trisnorsesquiterpene
Abstract
Terpenoids are enormously diverse, but our knowledge of their biosynthesis and functions is limited. Here we report on a terpene synthase (DdTPS8)-cytochrome P450 (CYP521A1) gene cluster that produces a novel C12 trisnorsesquiterpene and affects the development of Dictyostelium discoideum. DdTPS8 catalyzes the formation of a sesquiterpene discoidol, which is undetectable from the volatile bouquet of wild type D. discoideum. Interestingly, a DdTPS8 knockout mutant lacks not only discoidol, but also a putative trisnorsesquiterpene. This compound was hypothesized to be derived from discoidol via cytochrome P450 (CYP)-catalyzed oxidative cleavage. CYP521A1, which is clustered with DdTPS8, was identified as a top candidate. Biochemical assays demonstrated that CYP521A1 catalyzes the conversion of discoidol to a novel trisnorsesquiterpene named discodiene. The DdTPS8 knockout mutant exhibited slow progression in development. This study points to the untapped diversity of natural products made by D. discoideum, which may have diverse roles in its development and chemical ecology.
https://doi.org/10.7554/eLife.44352.001Introduction
With over 80,000 structures identified, terpenoids constitute the largest class of natural products made by living organisms (Christianson, 2017). Most terpenoid natural products are known from plants (Chen et al., 2011), but bacteria (Yamada et al., 2015; Dickschat, 2016) and fungi (Keller et al., 2005; Schmidt-Dannert, 2015) are also rich sources. Recently we have shown that dictyostelid social amoebae, a class of eukaryotic soil microorganisms, also have the genetic capacity to produce monoterpenes (C10), sesquiterpenes (C15) and diterpenes (C20) (Chen et al., 2016). Dictyostelid social amoebae have a unique life cycle, consisting of both unicellular and multicellular phases. When their bacterial food supply becomes scarce, amoebae start to aggregate, going through clearly-defined morphological changes to eventually form fruiting bodies (Kessin, 2001). Dictyostelium discoideum and D. purpureum are among the most extensively investigated species of dictyostelid social amoebae. Our recent studies illustrated that the multicellular stages of the life cycle of both D. discoideum (Chen et al., 2016) and D. purpureum (Chen et al., 2018) are characterized by the emission of a complex mixture of volatile organic compounds that is dominated by sesquiterpenes. While some terpenoids are produced by both species, most appear to be species-specific (Chen et al., 2018). The biological function of these terpenoids is completely unknown.
The D. discoideum genome contains nine full-length genes (DdTPS1-9) encoding terpene synthases, the pivotal enzymes that create the terpenoid carbon skeleton (Christianson, 2017). All nine genes are expressed during the multicellular stage, suggesting a role for volatile terpenoid biosynthesis in fruiting body development. Indeed, biochemical characterization of the encoded proteins DdTPS1-9 revealed terpene synthase activity for all tested enzymes. The sesquiterpene products of DdTPS1-4, DdTPS6, DdTPS7 and DdTPS9, as well as the diterpene product of DdTPS5, could be detected in the volatile bouquet of D. discoideum during multicellular development (Chen et al., 2016; Rabe et al., 2016a; Rinkel et al., 2017). D. discoideum also released the monoterpene linalool that was produced by several recombinant terpene synthases including DdTPS2, DdTPS3 and DdTPS9, suggesting that at least one of these enzymes functions as a monoterpene synthase in vivo. In brief, the in vitro products of all DdTPSs except DdTPS8 could be detected in the volatile bouquet of D. discoideum.
DdTPS8 has sesquiterpene synthase activity, but does not produce mono- or diterpenes in vitro (Chen et al., 2016). DdTPS8 exhibits the second highest level of expression among all nine DdTPS genes during development, so it was surprising to see that the major sesquiterpene product of DdTPS8 was not detected in the D. discoideum volatiles (Chen et al., 2016). One possibility is that the product of DdTPS8 serves as a substrate for other enzymes, particularly cytochrome P450s (CYPs). CYPs are heme-containing proteins that catalyze a wide variety of oxidative reactions (Coon, 2005), often on terpenoid substrates (Hamberger and Bak, 2013). In this context it is interesting to note that the profile of volatiles emitted by D. discoideum contains a number of unknown compounds that are candidates for derivatives of the DdTPS8 product. Here we present conclusive evidence that the DdTPS8 sesquiterpene product is modified by a CYP that is physically clustered with DdTPS8 on chromosome six and produces a novel trisnorsesquiterpene named as discodiene. A DdTPS8-knockout mutant of D. discoideum, which failed to produce discodiene, showed slower progression in development than the wild type strain. This raises interesting questions about the specific biological role of discodiene as one constituent of a bouquet of volatiles produced by D. discoideum during its development.
Results
The product of DdTPS8 is the new sesquiterpene alcohol discoidol
DdTPS8 had been shown to have sesquiterpene synthase activity in previous work (Chen et al., 2016), but the structure of its product was not identified. In the present study, the coding sequence of DdTPS8 was cloned into the expression vector pET32a and expressed heterologously in Escherichia coli. After protein purification by Ni2+-NTA affinity chromatography, the recombinant DdTPS8 enzyme was incubated with its substrate farnesyl diphosphate (FDP), resulting in the formation of a sesquiterpene alcohol as a single product. The compound was purified and structure elucidation by NMR spectroscopy (Supplementary file 1) revealed a new bicyclic sesquiterpenoid, which was named discoidol (Figure 1A). Discoidol is a stereoisomer of the known sesquiterpene alcohol jinkoh-eremol (Nakanishi et al., 1983).
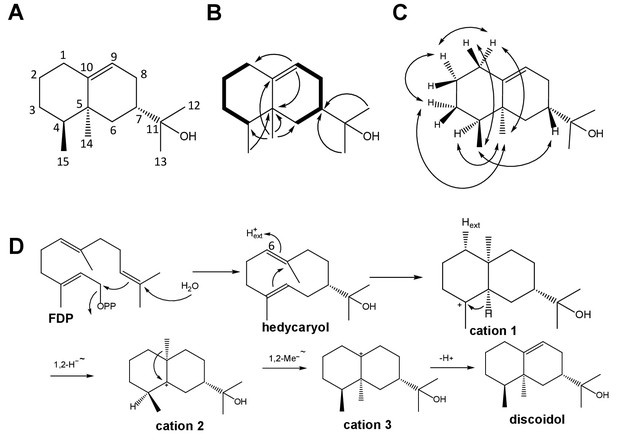
Structure elucidation of DdTPS8 product and biosynthetic mechanism.
(A) Structure of discoidol. (B) Contiguous spin systems indicated by bold lines observed in discoidol by 1H,1H-COSY NMR, single headed arrows indicate diagnostic HMBC correlations. (C) important NOESY correlations that are indicated by double headed arrows observed in discoidol. (D) biosynthetic mechanism from farnesyl diphosphate (FDP) to discoidol catalyzed by DdTPS8. See also Figure 1—figure supplements 1–4.
The absolute configuration of discoidol was determined by enzymatic conversion of enantioselectively deuterated (R)- and (S)-(1-13C,1-2H)geranyl diphosphate (Rabe et al., 2017) that were elongated with isopentenyl diphosphate to the corresponding FDP isotopomers using the FDP synthase from Streptomyces coelicolor (Rabe et al., 2016b). Their conversion by the discoidol synthase resulted in enantioselectively deuterated discoidol with known absolute configuration at the deuterated carbon (Figure 1B). The absolute configuration of discoidol was then deduced by assignment of the relative orientation of the two hydrogen atoms at C2 by NOESY (Figure 1C). The additional 13C-NMR label at this carbon was used for a highly efficient analysis by HSQC spectroscopy, giving an intensive cross-peak for the attached hydrogen (Figure 1—figure supplement 1). These experiments resulted in the assigned absolute configuration of (4S,5S,7S)-discoidol.
The proposed cyclization mechanism of discoidol synthase (Figure 1D) starts with a 1,10-cyclization of FDP with attack of water to yield the neutral intermediate hedycaryol. A protonation-induced second cyclization results in cation 1, that upon a 1,2-hydride shift to cation 2, 1,2-methyl group migration to cation three and deprotonation reacts to form discoidol. This mechanism was experimentally supported by a series of incubation experiments using isotopically labeled substrates. The reprotonation of hedycaryol was demonstrated by incubation of (6-13C)FDP (Rabe et al., 2015) with discoidol synthase in a deuterium oxide enriched buffer, resulting in a triplet in the 13C-NMR spectrum of the product cation 1 (Figure 1—figure supplement 2) that indicated a direct 13C-2H connection. The stereochemical course for the reprotonation was evident from HSQC analysis of the obtained labeled product (Rabe et al., 2016b), demonstrating reprotonation from the Si face of C6. The 1,2-hydride shift from cation 1 to cation two was followed with (3-13C,2-2H)FDP (Klapschinski et al., 2016), resulting in a triplet for C4 of discoidol that demonstrated a direct 13C-2H bond (Figure 1—figure supplement 3). Finally, the stereochemical fate of the terminal geminal methyl groups of FDP was followed with (12-13C)FDP and (13-13C)FDP (Rabe et al., 2015), showing a stereospecific incorporation of labeling into discoidol (Figure 1—figure supplement 4).
A DdTPS8 knockout mutant of D. discoideum lacks discoidol and a putative discoidol metabolite
The absence of the DdTPS8 product discoidol in D. discoideum culture coupled with the high level of expression of the corresponding gene, suggested that discoidol is produced but further modified in vivo. If this hypothesis holds true, the disruption of discoidol biosynthesis would abolish the biosynthesis of the modified product as well. Therefore, we analyzed a D. discoideum mutant in which DdTPS8 was disrupted by an insertion of a blasticidin resistance cassette between nucleotides 544 and 545 of the DdTPS8 open reading frame (Figure 2A), which was verified by sequencing (Figure 2—figure supplement 1). The DdTPS8 mutant was next allowed to develop until the culmination stage of multicellular development (the ultimate stage in fruiting body formation when the expression of DdTPS8 is the highest) and subjected to headspace chemical profiling. In comparison to the wild type, the DdTPS8 mutant lacked a major peak (peak one in Figure 2B) whose structure was unknown. This unknown compound appeared to have a molecular mass of 162, consistent with the mass of a degraded sesquiterpene that had lost a fragment containing three carbon atoms and one oxygen atom. Based on these findings, we hypothesized that the unknown compound is a putative C12 trisnorsesquiterpene derived from discoidol through C-C bond cleavage.
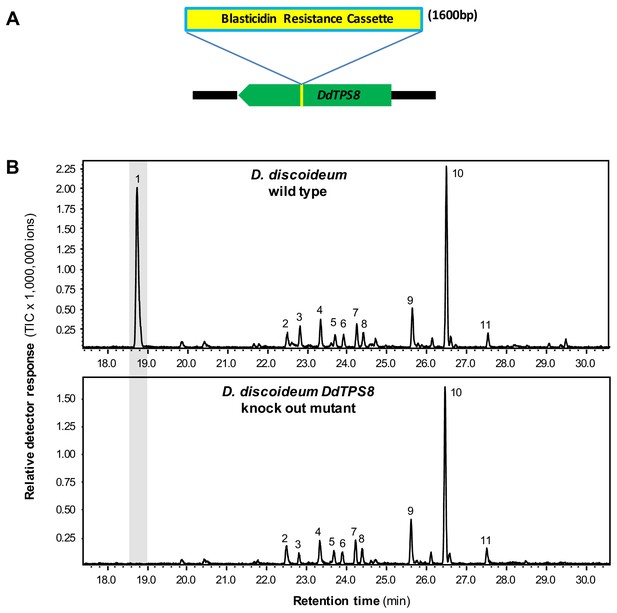
DdTPS8 insertional mutant and its volatile profile.
(A) Schematic presentation of DdTPS8 gene with an insert of 1.6 kb. (B) Volatiles were collected from the headspace of the cultures and analyzed using GC-MS. Total ion chromatograms are shown. 1, unknown compound; 2, unidentified compound; 3, unidentified sesquiterpene hydrocarbon; 4, unidentified compound; 5, β-maaliene; 6, aristolene; 7, calarene; 8–10, unidentified sesquiterpene hydrocarbons; 11, nerolidol. See also Figure 2—figure supplement 1.
The CYP family of D. discoideum: identification and coexpression analysis with DdTPS8
Among the diverse reactions catalyzed by CYPs with terpenes as substrates are oxidative degradations (Stanjek et al., 1999; Irmler et al., 2000; Larbat et al., 2009; Lee et al., 2010). This led us to hypothesize that the cleavage of discoidol to form the unknown compound is catalyzed by a CYP. Analysis of the D. discoideum genome led to the identification of a total of 54 putative CYP genes. Among them, 41 were annotated as full-length intact genes whereas the rest were pseudogenes or partial genes (Supplementary file 2). The 41 full-length genes were assigned to 17 families and 34 subfamilies (Supplementary file 2).
Coexpression analysis of TPS genes and CYP genes has been a useful tool to identify candidate CYPs that catalyze the modification of TPS products (e.g. Ginglinger et al., 2013). Thus we performed coexpression analysis of CYP genes with DdTPS8 in D. discoideum based on their expression patterns during the 24 hr of multicellular development that consists of several stages: vegetative growth, streaming, loose aggregate, mound, Mexican hat, and fruiting body (Figure 3A). Gene expression data of CYP genes and DdTPS8 were obtained from dictyExpress (http://dictyexpress.biolab.si) (Parikh et al., 2010) and used to calculate Pearson correlation coefficients (r) between individual CYP genes and DdTPS8. Among the 41 CYP genes, three showed significant correlation coefficients with DdTPS8 exhibiting a P value lower than 0.001 (Supplementary file 3): CYP521A1 (r = 0.994), CYP508C1 (r = 0.992) and CYP519C1 (r = 0.951). These three CYP genes, like DdTPS8, showed a maximal level of expression at 16 hr during multicellular development (Figure 3A). Examination of gene expression during development revealed that the transcripts of both DdTPS8 and CYP521A1 were nearly undetectable in vegetatively growing cells. Small amounts of transcripts accumulated between 4–8 hr of development, continued to accumulate until they peaked at 16 hr, and declined thereafter (Figure 3A). We also noticed that the top candidate CYP521A1 is located 685 bp away from DdTPS8 in a head-to-head configuration on chromosome 6 (Figure 3B), suggesting a possibility that DdTPS8 and CYP521A1 form a biosynthetic cluster.
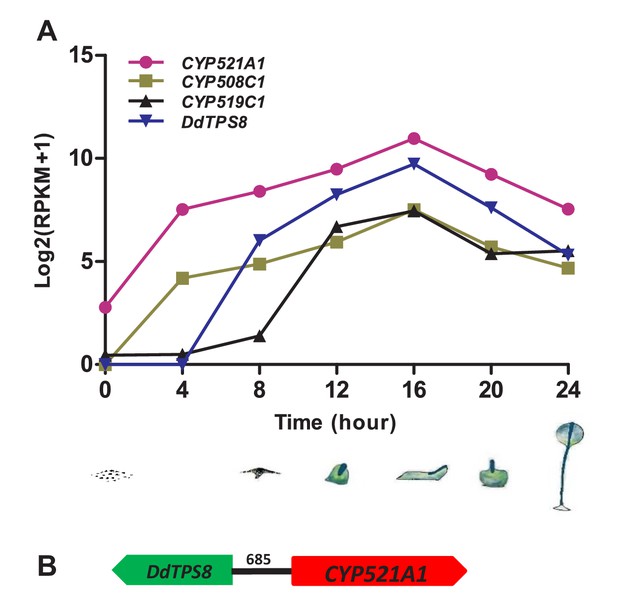
Cytochrome P450 (CYP) genes associated with DdTPS8.
(A) Expression pattern of three CYP genes that showed highest level of coexpression coefficient with DdTPS8. The cartoons show the six stages of multicellular development of D. discoideum: individual cells (0 hr), streaming (8 hr), loose aggregate (10 hr), slug (16 hr), Mexican hat (20 hr) and fruiting bodies (24 hr). (B) DdTPS8 and CYP521A1 are neighbor genes. The number above the black line indicates the length of the intergenic region in base pairs.
CYP521A1 catalyzes the oxidative degradation of discoidol to form the novel trisnorsesquiterpene discodiene
In our first attempts to characterize the enzymatic activity of CYP521A1, we expressed it together with yeast or Arabidopsis P450 reductases in Saccharomyces cerevisiae and incubated the resulting microsome preparations with discoidol. However, no enzymatic activity was detected. Assays containing yeast microsomes, recombinant DdTPS8 produced in Escherichia coli, and (E,E)-FDP showed no activity either. The lack of activity could be due to the incompatibility of the P450 reductase from a plant (Arabidopsis) or a fungus (yeast) with a CYP from D. discoideum. As such, we turned to a P450 reductase from D. discoideum. Among the three P450 reductase genes, redA, redB and redC, of D. discoideum, redB showed an expression pattern (Gonzalez-Kristeller et al., 2008) similar to that of DdTPS8 and CYP521A1. Thus, we selected redB for our assays. The open reading frames of CYP521A1 and redB were inserted into the vector pRSFDuet−1, which allows their coexpression in E. coli. The resulting constructs, together with another expression vector carrying the complete open reading frame of DdTPS8, were both introduced into E. coli Bl21 (DE3)-Star. Assuming that the intrinsic FDP pool of E. coli is sufficient to provide substrate for DdTPS8, we analyzed potential terpene accumulation in the headspace of the resulting E. coli culture (Figure 4A). Indeed, GC-MS analysis confirmed the formation of both discoidol with a molecular mass of m/z = 222 (Figure 4B) and the unknown terpenoid with a molecular mass of m/z = 162 (Figure 4C). E. coli cells harboring DdTPS8, redB, and CYP508C1, another P450 gene highly coexpressed with DdTPS8, produced only discoidol and no further terpenoids (Figure 4A). When CYP521A1 and redB were expressed in the absence of DdTPS8, no terpene formation was observed. To test the enzyme activities in a cell-free system, a crude protein extract made from E. coli expressing CYP521A1, redB, and DdTPS8 was incubated with (E,E)-FDP and NADPH. Although product formation was rather low, we were able to detect discoidol and the P450 metabolite with m/z = 162 in the headspace of the assay (Figure 4—figure supplement 1).
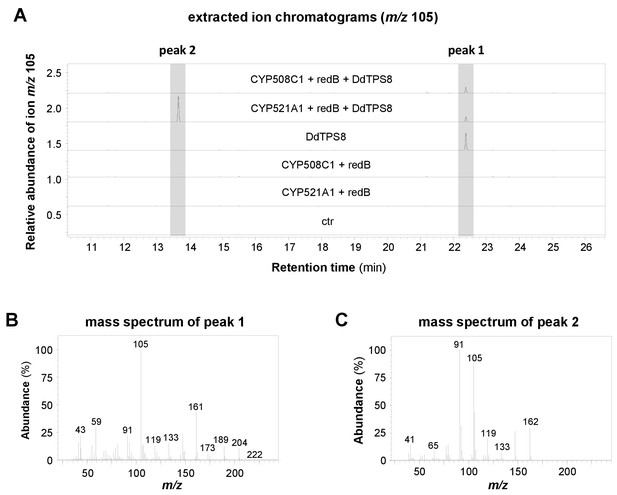
Volatile profiles of E. coli Bl21-DE3-Star expressing different combinations of CYP521A1, CYP508C1, the P450 reductase gene RedB, and the terpene synthase gene DdTPS8.
Volatiles were collected from the headspace of the induced bacterial cultures using PDMS tubes and analyzed using GC-TDU-MS. The extracted ion chromatograms for m/z 105 (A) and the mass spectra of the DdTPS8 product (B) and CYP521A1 product (C) are shown. See also Figure 4—figure supplement 1.
Production of the unknown P450 oxidation product in all of the assays tested was too low for NMR analysis. However, the molecular ion at m/z = 162 in the EI mass spectrum (Figure 5A), pointed to a degradation of discoidol with loss of a fragment representing a molecular weight of 60 Da. The same reaction with completely labeled (13C15)discoidol, obtained from (13C15)FDP (Rabe et al., 2015) with discoidol synthase, produced a degradation product with incorporation of twelve 13C atoms (Figure 5B), demonstrating that the degradation product is a trisnorsesquiterpene. Furthermore, the fragment ion at m/z = 59 observed in the mass spectrum of discoidol (indicative of its 1-hydroxy-1-methylethyl group) was missing, suggesting that the degradation may have affected this portion of the molecule. There are two plausible hypotheses for the reaction catalyzed by CYP521A1 that would be consistent with the observed mass spectrum of the trisnorsesquiterpene (Figure 5—figure supplement 1). The reactive iron-oxo species of the cytochrome P450 could initiate the degradation reaction by hydrogen abstraction from the carbon originating from C-9 of FPP with formation of a stabilized allyl radical or from C-1 of FDP leading to a less stable radical (Figure 5—figure supplement 1). The radical intermediate then can react in the oxygen rebound by the cleavage of acetone and formation of water, resulting in different C = C double bond positions in the C12H18 product. To confirm the loss of the 1-hydroxy-1-methylethyl group and to distinguish between the alternative products, an incubation experiment with (11-13C,1,1-2H2)FDP (Rinkel et al., 2016) was performed (Figure 5—figure supplement 1). This substrate was converted into (11-13C,6,6-2H2)−1 by the discoidol synthase and degraded by CYP521A1 into a product with a mass spectrum showing the loss of the 13C label from the 1-hydroxy-1-methylethyl group (Figure 5C), but retaining both deuterium atoms (Figure 5D). This result is consistent with the mechanism that leads to a product with a conjugated double bond system, but not with the mechanism that would result in a product with the non-conjugated double bonds (Figure 5—figure supplement 1). The tentatively identified compound is a new natural product for which we propose the name discodiene. A full structure elucidation of discodiene by NMR spectroscopy was not possible, because of the poor conversion of discoidol by CYP521A1. Future structure elucidation may be possible by synthesis of a reference compound.
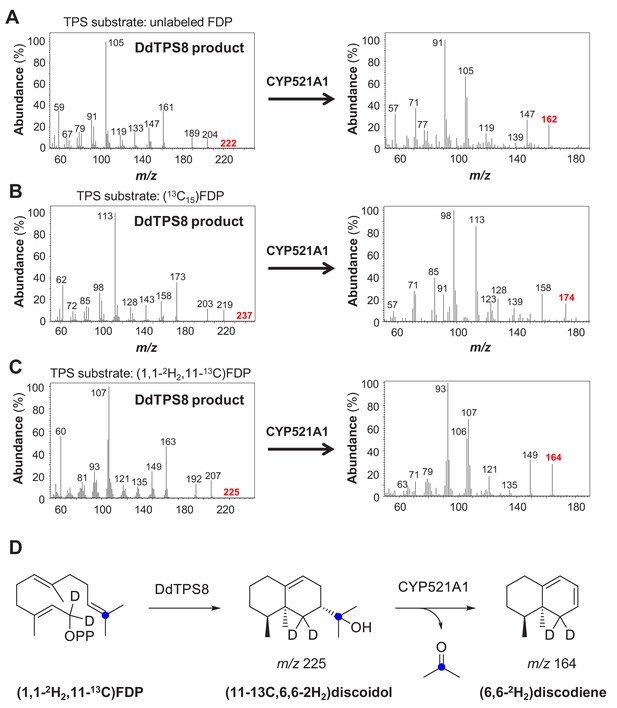
Mass spectra (A–C) and structures (D) of DdTPS8 products and CYP521A1 products derived from unlabeled farnesyl diphosphate (FDP) (A), 13C15-FDP (B), and 1,1–2 H2,11–13C-FDP (C + D).
The genes were coexpressed in E. coli Bl21-DE3-Star together with the P450 reductase gene RedB. Crude protein extracts were incubated with unlabeled or labeled (E,E)-FDP and volatile enzyme products were collected from the headspace of the assays using PDMS tubes. Product analysis was performed with GC-TDU-MS. See also Figure 5—figure supplement 1.
The DdTPS8 knockout mutant of D. discoideum displayed delayed multicellular development
To examine the biological function of discodiene, cells of the DdTPS8 mutant strain and the wild type parental AX4 strain were grown separately in HL5 medium. They were then starved, deposited on black nitrocellulose filters, and allowed to develop and their morphologies were compared. Comparing their morphologies, the mutant and the wild type developed well on black filters for the first few hours and there were no differences between them. However, a difference was observed after 16 hr of starvation: the multicellular development of the mutant was delayed compared to the wild type (Figure 6). At this time point, the wild type cells formed fingers, whereas the mutant was mainly at the tipped aggregate stage. At 20 hr (Figure 6), the wild type began to transition from fingers into so-called ‘Mexican hats’, so a mix of the two stages was observed, while the mutant was delayed at the finger stage. It is also worthwhile noting that the mutant fingers were slightly elongated and narrower compared to the wild type. At 24 hr, the wild type began entering the culmination stage, which involves complex cell movements to form a ball of spores carried on top of a cellular stalk. However, the mutant was still mostly at the finger stage with some Mexican hats visible (Figure 6). Eventually, both the wild type and the mutant developed into well-proportioned fruiting bodies, with stalks and spores, and they were largely indistinguishable (Figure 6, 54h). Spore formation and germination were also compared but no differences were observed between the wild type and the DdTPS8 mutant. These data suggest that DdTPS8 has a function during later stages of multicellular development in D. discoideum, during the transition from fingers to Mexican hats, which is consistent with its temporal expression pattern (Figure 3A).
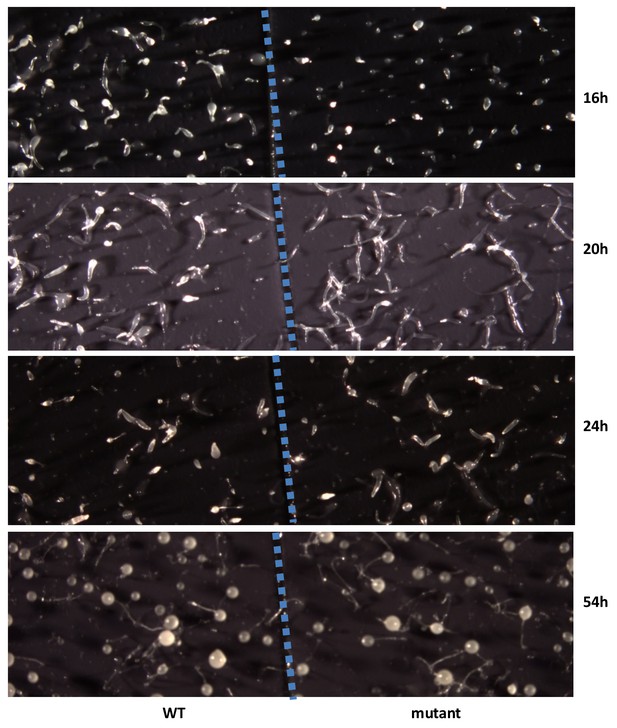
Developmental phenotype of the DdTPS8 knockout mutant.
Wild type AX4 cells (WT) and mutant DdTPS8 cells (mutant) were grown separately in HL5 to the log phase, washed in buffer, and plated clonally on dark nitrocellulose filters. The filters were cut in half and placed next to each other in one dish. The cells were incubated in the dark at 22°C and photographed from above with a dissecting microscope at the indicated times. This experiment was independently performed three times with same results and the data shown represent one of the replicates.
Other putative TPS-CYP gene clusters in D. discoideum and D. purpureum
Following the functional validation of the DdTPS8-CYP521A1 gene cluster, we examined other members of the TPS and CYP families in D. discoideum and identified two additional putative TPS-CYP gene clusters (Figure 7A). DdTPS2 is organized in a head-to-head fashion with CYP519C1 and the two are separated by an intergenic region of 580 bp. Interestingly, the opposite side of CYP519C1 is adjacent to DpTPS11, which encodes a predicted partial terpene synthase (Chen et al., 2016). DdTPS3 also appear to be part of a gene cluster that contains two CYP genes. The immediate neighbor of DdTPS3 is a partial CYP gene CYP515A2_ps. Nevertheless, in a distance of 3148 bp from the start codon of DdTPS3 there is an intact CYP512A1 gene, which is arranged in a tail-to-head fashion with DdTPS3.
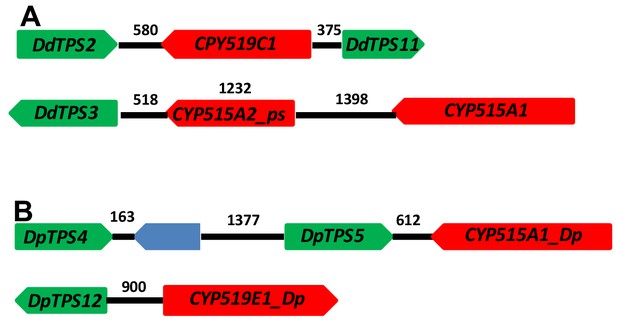
TPS-CYP gene clusters in D. discoideum (A) and D. purpureum (B).
Green blocks depict TPS genes, red blocks indicate CYP genes. The blue block indicates a non-TPS/CYP gene. The numbers above the black lines indicate length in base pairs.
As mentioned earlier, D. purpureum is related to D. discoideum and its TPS family has been comprehensively characterized in our recent study (Chen et al., 2018). For comparison, we also analyzed the CYP family in D. purpureum and searched for putative TPS-CYP clusters in this species. We found a total of 54 putative CYP genes (Supplementary file 4). Among them, 47 were annotated as full-length intact genes whereas the remaining are partial genes. The 47 full-length genes were assigned to 12 families and 29 subfamilies. By examining the physical locations of TPS and CYP genes, two putative TPS-CYP clusters were identified in D. purpureum: DpTPS5 is linked to CYP5121A1_Dp with an intergenic region of 612 bp and DdTPS12 is linked to CYP919E1_Dp with an intergenic region of 900 bp (Figure 7B). In the former cluster, DdTPS5 is in a tandem repeat with DdTPS4.
Discussion
In this study, we identified and characterized a terpene synthase (DdTPS8)-cytochrome P450 (CYP521A1) gene cluster in the social amoeba D. discoideum (Figure 3) that encodes two enzymes catalyzing consecutive reactions to form the novel trisnorsesquiterpene discodiene (Figure 4, Figure 5). We also present evidence that the biosynthesis of discodiene has an effect on multicellular development of D. discoideum (Figure 6). The successful determination of CYP521A1 as discodiene synthase relied on a number of lines of evidence. First, it was clear that the sesquiterpene product of DdTPS8, discoidol (Figure 1), does not accumulate (Figure 2) and thus must be further modified after formation. Second, chemical profiling of the DdTPS8 knockout mutant of D. discoideum in comparison to the wild type suggested that discoidol is converted into a degradation product (Figure 2). Third, coexpression analysis and colocalization in the genome implicated CYP521A1 as the top candidate that acts downstream of DdTPS8 (Figure 3). Fourth, enzyme assays confirmed that cytochrome CYP521A1 converts discoidol into a trisnorsesquiterpene that was tentatively identified by an isotopic labeling strategy as discodiene (Figure 5), the same compound that is produced by the wild type D. discoideum but not the DdTPS8 mutant (Figure 2).
DdTPS8 is a microbial terpene synthase that shares many mechanistic features of other typical plant terpene synthases, such as a protonation-induced cyclization of a neutral intermediate, a 1,2-hydride shift and a methyl group migration (Degenhardt et al., 2009). DdTPS8 catalyzes the formation of a new sesquiterpene alcohol, discoidol (Figure 1). It is interesting to note that the most closely related terpene synthase in D. discoideum, DdTPS4, also forms a sesquiterpene alcohol (Chen et al., 2016). However, the product of DdTPS4, (E)-nerolidol, is an acyclic compound in contrast to the cyclic product of DdTPS8. Based on evolutionary relatedness, the encoding genes can be inferred to have arisen from a gene duplication event. It will be interesting to determine the mechanism underlying the functional divergence of DdTPS4 and DdTPS8 in forming acyclic and cyclic sesquiterpene alcohols, respectively. It is also interesting to note that a stereoisomer of discoidol known as jinkoh-eremol has been previously isolated from an agarwood (Aquilaria sp.), a eudicotyledon flowering plant (Nakanishi et al., 1983). Since the biosynthesis of jinkoh-eremol in agarwood is most likely catalyzed by typical plant terpene synthases, which are only distantly related to the microbial type terpene synthases that include D. discoideum TPSs (Jia et al., 2016), convergent evolution has occurred in D. discoideum and plants for the biosynthesis of similar terpenes. Such convergence has been previously reported, as in the recent discovery that the fungal diterpene phomopsene is the product of a bacterial terpene synthase (Lauterbach et al., 2018). However, bacterial terpene synthases are frequently observed to make the opposite enantiomers of terpenes found in plants (Rabe et al., 2016a), which clearly points to different evolutionary origins in these cases.
Discodiene, the degradation product of discoidol, is a novel trisnorsesquiterpene natural product. Another important member of this group is geosmin, a particularly widespread compound in soil microorganisms that exhibits the smell of earth. While it was initially speculated that geosmin may be formed by oxidation of a terpene synthase product (Spiteller et al., 2002; Cane and Watt, 2003), it became later evident from feeding experiments (Dickschat et al., 2005) and characterization of the enzyme (Jiang et al., 2007) that a bifunctional terpene synthase catalyzes the formation of this compound involving initial cyclization to an enantiomer of hedycaryol followed by a second cyclization and oxidative cleavage of a 1-hydroxy-1-methylethyl unit. Trisnorsesquiterpenes with loss of the same 1-hydroxy-1-methylethyl unit as in discodiene have been reported from the myxobacterium Chondromyces crocatus (Schulz et al., 2004) and from streptomycetes (Citron et al., 2012), but the enzymology and mechanism of their formation are unclear. Oxidative dealkylations such as the reaction catalyzed by CYP521A1 converting discoidol into discodiene are known from a few other cytochrome P450s, including angelicin synthase (CYP71AJ4) from Ammi majus (Larbat et al., 2009), secologanin synthase (CYP72A1) from Catharanthus roseus (Irmler et al., 2000), DMNT/TMTT synthase (CYP82G1) from Arabidopsis (Lee et al., 2010), and the degradation of (+)-marmesin to psoralene by the psoralene synthase (Stanjek et al., 1999). All these enzymes are derived from plants, belonging to different CYP families. The fact that D. discoideum and plants belong to different kingdoms suggests convergent evolution of CYPs for catalyzing oxidative cleavage reactions.
D. discoideum morphogenesis is a tightly regulated developmental process that begins with the aggregation of individual cells into a mound of approximately 50,000 cells and ends with the formation of a fruiting body that consists of spores and a stalk (Kessin, 2001). The culmination stage, which is marked by the onset of stalk formation, occurs at the end of development, between 16 and 24 hr after the process begins. The DdTPS8 knockout mutant exhibited delayed development at the culmination stage (Figure 6), around the same time that DdTPS8 and CYP521A1 exhibited their maximal level of expression, suggesting a potential role of discodiene in regulating D. discoideum development. A number of metabolites have been demonstrated to regulate Dictyostelium development, such as the polyketides DIF-1 (Thompson and Kay, 2000) and 4-methyl-5-pentylbenzene-1,3-diol (Anjard et al., 2011), gamma-aminobutyric acid, the nucleotide cyclic diguanylate, the cytokinin discadenine and an unidentified steroid (Loomis, 2014; Schaap, 2016). One major difference between discodiene and these metabolites is that discodiene is a volatile. Since disrupting DdTPS8 did not fully block the development of D. discoideum, discodiene might be merely a component of the volatile bouquet needed for development. It is also possible that discodiene may not control developmental programs directly, but rather influence them indirectly by acting as an ‘aggregation/culmination pheromone’ as already speculated for volatile terpenoids in D. discoideum (Chen et al., 2016).
The organization of terpenes synthase genes and CYP genes involved in the same biochemical pathway as metabolic gene clusters has been documented in a growing number of cases including bacteria (Nett et al., 2017), fungi (Quin et al., 2014), and plants (Boutanaev et al., 2015). Our findings add the Dictyostelium social amoebae to this list. With only 9 TPS genes in D. discoideum, it is unlikely that the clustering of with CYP genes (Figure 3B and Figure 7A) is accidental. It is equally intriguing to observe that D. purpureum contains two TPS-CYP clusters (Figure 7B). With the evolutionary relationship of TPS genes from D. discoideum and D. purpureum recently determined (Chen et al., 2018), we are able to examine the relatedness of these TPS-CYP gene clusters. The ortholog of DdTPS8 in D. purpureum is DpTPS6 (Chen et al., 2018), which is not clustered with any CYP gene. Moreover, D. purpureum lacks an ortholog of CYP521A1, implying that the D. purpureum may not produce discodiene, consist with the volatile profiling of this species (Chen et al., 2018). DdTPS2-CYP519C1 and DpTPS2-CYP919E1_Dp appear to be species-specific. In contrast, DdTPS3-CYP515A1 and DpTPS5-CYP515A1_Dp are an orthologous pair based on the orthology of the respective TPS genes (DdTPS3 and DpTPS5) (Chen et al., 2018) and CYP genes (CYP515A1 and CYP515A1-Dp are in the same subfamily). Taken together, these TPS-CYP clusters suggest the biosynthesis of both shared as well as species-specific terpenoids in D. discoideum and D. purpureum.
Besides terpenoids, dictyostelid social amoebae produces many other types of natural products (Barnett and Stallforth, 2018), including polyketides. Polyketide synthases (PKSs) are pivotal enzymes for polyketide biosynthesis (Khosla, 2009) and the production of some polyketides involves CYPs (e.g., Bedewitz et al., 2018). The D. discoideum genome contains 40 PKS genes (Zucko et al., 2007). With the demonstration of the DdTPS8-CYP521A1 cluster catalyzing consecutive reactions to produce a novel natural product, the potential for generating chemical diversity of natural products in D. discoideum using the building blocks derived from 9 TPSs, 41 CYPs and 40 PKSs is enormous, which can be envisioned to be a fruitful future research direction. It would be interesting to know what functions these natural products have, especially in chemical communications between the organism and its environment.
Materials and methods
Strains of D. discoideum and mutant analysis
Request a detailed protocolThe wild type AX4 strain of D. discoideum (DBS0237637) was obtained from the Dictybase Stock Center (http://dictybase.org/). The DdTPS8 mutant was obtained from the gridded collection of the Genome Wide Dictyostelium Insertion (GWDI) Project (https://remi-seq.org). The mutant stain was cultured clonally and the genotype was verified by diagnostic PCR with insertion (Blasticidin resistance gene) specific and gene specific primers (Supplementary file 5), followed by characterization with gene-insert specific primers to verify the location of the insertion. To characterize the developmental progression of the mutant, we grew both wild type (AX4) and DdTPS8 mutant in HL5 liquid medium to the mid-log growth phase. We then collected the cells by centrifugation, washed them and deposited them on black nitrocellulose filters (Shaulsky and Loomis, 1993). We examined developmental morphology and photographed the structures from above using a dissecting microscope. This experiment was repeated three times.
Headspace collection and GC-MS analysis
Request a detailed protocolCulturing of the wild type AX4 and the DdTPS8 mutant strain of D. discoideum, headspace collection and chemical identification using GC-MS were performed as previously reported (Chen et al., 2016) with three biological replicates.
CYP gene search and gene co-expression analysis
Request a detailed protocolProteome sequences of D. discoideum and D. purpureum were downloaded from Dictybase (http://dictybase.org) and were used as dataset for identifying CYP genes. A HMM model of P450 gene family (PF00067) downloaded from Pfam 31.0 (http://pfam.xfam.org) was used to search putative p450 genes against the downloaded protein dataset using HMMER 3.1b two with an e-value cutoff of 1e−2. CYPs were blast searched against named CYPs from all protists. Sequences were sorted based on best blast hit percent ID and named based on the Standardized Cytochrome P450 nomenclature rules (Nelson et al., 1996).
Expression data of CYP genes in D. discoideum during its 24 hr developmental program were obtained from online web-interface program DictyExpress (https://dictyexpress.research.bcm.edu). Pearson correlation coefficients (PCCs) between the expression of DdTPS8 and that of CYP genes of D. discoideum were calculated using IBM SPSS (v.25, https://www.ibm.com).
Cloning of two CYP genes, redB gene and vector construction
Request a detailed protocolFull-length cDNAs for two CYP genes, CYP521A1 and CYP508C1, and one cytochrome p450 reductase gene (DDB_G0269912, known as redB) were cloning by RT-PCR. Approximately 0.1 g tissue of D. discoideum at the culmination stage was collected and disrupted by TissueLyser II (https://www.qiagen.com). Total RNA was isolated using RNeasy Plant Mini Kit (https://www.qiagen.com) and converted to cDNAs using the First strand cDNA synthesis kit (https://www.gelifesciences.com). Full-length cDNAs for each of the three genes were amplified by PCR using gene-specific primers (Supplementary file 5), cloned into pGEM-T Easy vector (https://www.promega.com), and fully sequenced. Next, the redB gene was added with the NdeI and KpnI restriction sites using PCR and ligated into the NdeI and KpnI site of the second MSC of the vector pRSFDuet1 (http://www.emdmillipore.com) to produce pRSFDuet1::redB. Then CYP521A1 and CYP508C1 were cloned into the BamH1 and PstI sites of the first MSC of pRSFDuet1::redB to produce two plasmids pRSFDuet1:: CYP521A1::redB and pRSFDuet1:: CYP508C1::redB.
DdTPS8 protein purification and product isolation
Request a detailed protocolA full-length cDNA for DdTPS8 was cloned into the pET32a vector. E. coli BL 21 harboring pET32a_DdTPS8 was inoculated in a LB liquid preculture containing ampicillin (50 mg/L). For protein isolation the preculture was used to inoculate large scale 2YT liquid cultures (8 × 1 L) containing ampicillin (50 mg/L). Cells were grown to an OD600 = 0.4 at 37°C and 160 rpm, followed by cooling of the cultures to 18°C. IPTG (0.4 mm) was added and the culture was further incubated at 18°C and 160 rpm over night. Cells were harvested by centrifugation at 4°C and 3600 rpm for 30 min. The supernatant was discarded and the cell pellet was resuspended in 80 mL binding buffer (20 mm Na2HPO4, 0.5 m NaCl, 20 mm imidazole, 1 mm MgCl2, pH 7.0). Cell disruption was done by ultra-sonication on ice for 8 × 60 s. The cell debris was removed by repeated centrifugation (2 × 10 min) at 4°C and 11000 rpm to yield the soluble enzyme fractions. Protein purification was performed by Ni2+-NTA affinity chromatography with Ni2+-NTA superflow (Qiagen) using binding buffer (4 × 20 mL; 20 mm Na2HPO4, 0.5 m NaCl, 20 mm imidazole, 1 mm MgCl2, pH 7.0) and elution buffer (4 × 20 mL; 20 mm Na2HPO4, 0.5 m NaCl, 20 mM imidazole, 1 mm MgCl2, pH 7.0). The enzyme fractions were used for enzyme reactions with the natural substrate FDP (50 mg, final concentration c = 0.2 mg/mL). The incubation experiment was performed at 28°C for 3 hr and was extracted three times with hexane. The combined organic layers were dried over MgSO4 and concentrated under reduced pressure. Column chromatography on silica gel (pentane: diethyl ether 5:1) yielded the terpene alcohol (5.0 mg) as colorless oil.
E. coli expression and headspace analysis
Request a detailed protocolThe two plasmids pEXP5-CT/TOPO::DdTPS8 and pRSFDuet1:: CYP:: redB were cotransformed into E. coli Bl21. The resultant strain was cultured overnight and then induced for protein expression by adding isopropyl β-D-1-thiogalactopyranoside. Volatiles emitted from the E. coli culture were collected by solid phase microextraction for 1 hr, and analyzed by GC-MS. Protein expression and headspace collections were repeated three times.
Enzyme reactions with isotopically labeled substrates
Request a detailed protocolIncubation experiments with DdTPS8 and labeled substrates were performed with the pure protein fractions obtained from 300 mL E. coli BL 21 pET32a_DdTPS8 liquid culture as reported above. All incubation experiments were done with the isotopically labeled substrate (0.6–1 mg, c = 0.1 mg/mL) at 28°C for 3 hr. The reaction mixtures were extracted with benzene-d6 (0.8 mL), the organic layers were separated and directly analyzed by GC/MS and NMR. For the determination of the absolute configuration of the DdTPS8 product (R)-(1-13C,1-2H) and (S)-(1-13C,1-2H)geranyl diphosphate (each 0.8 mg) were elongated with FDP synthase and isopentenyl diphosphate (0.5 mg) in the same reaction vessel and extracted after 3 hr incubation at 28°C.
Data availability
The sequence for CYP521A1 has been deposited in the GenBank database (accession no. MH923436).
References
-
Natural products from social amoebaeChemistry - a European Journal 24:4202–4214.https://doi.org/10.1002/chem.201703694
-
Structural and chemical biology of terpenoid cyclasesChemical Reviews 117:11570–11648.https://doi.org/10.1021/acs.chemrev.7b00287
-
Cytochrome P450: nature's most versatile biological catalystAnnual Review of Pharmacology and Toxicology 45:1–25.https://doi.org/10.1146/annurev.pharmtox.45.120403.100030
-
A novel type of geosmin biosynthesis in myxobacteriaThe Journal of Organic Chemistry 70:5174–5182.https://doi.org/10.1021/jo050449g
-
Plant P450s as versatile drivers for evolution of species-specific chemical diversityPhilosophical Transactions of the Royal Society B: Biological Sciences 368:20120426.https://doi.org/10.1098/rstb.2012.0426
-
Biosynthesis of the earthy odorant geosmin by a bifunctional Streptomyces coelicolor enzymeNature Chemical Biology 3:711–715.https://doi.org/10.1038/nchembio.2007.29
-
Fungal secondary metabolism - from biochemistry to genomicsNature Reviews Microbiology 3:937–947.https://doi.org/10.1038/nrmicro1286
-
BookDictyostelium: Evolution, Cell Biology, and the Development of MulticellularityCambridge University Press.
-
Structures and mechanisms of polyketide synthasesThe Journal of Organic Chemistry 74:6416–6420.https://doi.org/10.1021/jo9012089
-
Pristinol, a sesquiterpene alcohol with an unusual skeleton from Streptomyces pristinaespiralisAngewandte Chemie International Edition 55:10141–10144.https://doi.org/10.1002/anie.201605425
-
Isolation and functional characterization of CYP71AJ4 encoding for the first P450 monooxygenase of angular furanocoumarin biosynthesisJournal of Biological Chemistry 284:4776–4785.https://doi.org/10.1074/jbc.M807351200
-
Two bacterial diterpene synthases from Allokutzneria albata produce bonnadiene, phomopsene, and allokutznereneAngewandte Chemie International Edition 57:8280–8283.https://doi.org/10.1002/anie.201803800
-
Cell signaling during development of DictyosteliumDevelopmental Biology 391:1–16.https://doi.org/10.1016/j.ydbio.2014.04.001
-
Jinkoh-eremol and jinkohol II, two new sesquiterpene alcohols from agarwoodJournal of the Chemical Society, Perkin Transactions 1 1:601–604.https://doi.org/10.1039/p19830000601
-
Elucidation of gibberellin biosynthesis in bacteria reveals convergent evolutionNature Chemical Biology 13:69–74.https://doi.org/10.1038/nchembio.2232
-
Traversing the fungal terpenomeNatural Product Reports 31:1449–1473.https://doi.org/10.1039/C4NP00075G
-
Conformational analysis, thermal rearrangement, and EI-MS fragmentation mechanism of (1(10)E,4E,6S,7R)-germacradien-6-ol by (13)C-labeling experimentsAngewandte Chemie International Edition 54:13448–13451.https://doi.org/10.1002/anie.201507615
-
Terpene cyclases from social amoebaeAngewandte Chemie International Edition 55:15420–15423.https://doi.org/10.1002/anie.201608971
-
Mechanistic investigations on six bacterial terpene cyclasesBeilstein Journal of Organic Chemistry 12:1839–1850.https://doi.org/10.3762/bjoc.12.173
-
Mechanistic investigations of two bacterial diterpene cyclases: spiroviolene synthase and tsukubadiene synthaseAngewandte Chemie International Edition 56:2776–2779.https://doi.org/10.1002/anie.201612439
-
Lessons from 1,3-hydride shifts in sesquiterpene cyclizationsAngewandte Chemie International Edition 55:13593–13596.https://doi.org/10.1002/anie.201608042
-
Mechanisms of the diterpene cyclases β-pinacene synthase from Dictyostelium discoideum and hydropyrene synthase from Streptomyces clavuligerusChemistry - a European Journal 23:10501–10505.https://doi.org/10.1002/chem.201702704
-
Evolution of developmental signalling in dictyostelid social amoebasCurrent Opinion in Genetics & Development 39:29–34.https://doi.org/10.1016/j.gde.2016.05.014
-
Biosynthesis of terpenoid natural products in fungiAdvances in Biochemical Engineering/biotechnology 148:19–61.https://doi.org/10.1007/10_2014_283
-
Cell type regulation in response to expression of ricin A in DictyosteliumDevelopmental Biology 160:85–98.https://doi.org/10.1006/dbio.1993.1288
-
Biosynthesis of psoralen: mechanism of a cytochrome P450 catalyzed oxidative bond cleavageAngewandte Chemie International Edition 38:400–402.https://doi.org/10.1002/(SICI)1521-3773(19990201)38:3<400::AID-ANIE400>3.0.CO;2-K
-
The role of DIF-1 signaling in Dictyostelium developmentMolecular Cell 6:1509–1514.https://doi.org/10.1016/S1097-2765(00)00147-7
Article and author information
Author details
Funding
University of Tennessee Institute of Agriculture
- Feng Chen
The funders had no role in study design, data collection and interpretation, or the decision to submit the work for publication.
Acknowledgements
We thank Christopher Thompson and the GWDI team for providing the DdTPS8 mutant before the public release of the mutant collection and Dr. Guo Wei for discussions about CYP enzyme assays.
Copyright
© 2019, Chen et al.
This article is distributed under the terms of the Creative Commons Attribution License, which permits unrestricted use and redistribution provided that the original author and source are credited.
Metrics
-
- 2,015
- views
-
- 389
- downloads
-
- 11
- citations
Views, downloads and citations are aggregated across all versions of this paper published by eLife.
Citations by DOI
-
- 11
- citations for umbrella DOI https://doi.org/10.7554/eLife.44352