Compartmentalized oskar degradation in the germ plasm safeguards germline development
Abstract
Partitioning of mRNAs into ribonucleoprotein (RNP) granules supports diverse regulatory programs within the crowded cytoplasm. At least two types of RNP granules populate the germ plasm, a cytoplasmic domain at the posterior of the Drosophila oocyte and embryo. Germ granules deliver mRNAs required for germline development to pole cells, the germ cell progenitors. A second type of RNP granule, here named founder granules, contains oskar mRNA, which encodes the germ plasm organizer. Whereas oskar mRNA is essential for germ plasm assembly during oogenesis, we show that it is toxic to pole cells. Founder granules mediate compartmentalized degradation of oskar during embryogenesis to minimize its inheritance by pole cells. Degradation of oskar in founder granules is temporally and mechanistically distinct from degradation of oskar and other mRNAs during the maternal-to-zygotic transition. Our results show how compartmentalization in RNP granules differentially controls fates of mRNAs localized within the same cytoplasmic domain.
Introduction
The earliest stages of animal development generally occur in the absence of transcription. Thus, spatial and temporal control of gene activity required during this crucial period must be imposed post-transcriptionally. Due in part to its relatively late zygotic genome activation (ZGA) and consequent reliance on maternally synthesized transcripts, Drosophila has served as a valuable model for studying post-transcriptional gene regulation. Mechanisms including mRNA localization, translational control, and mRNA degradation are critical for establishing the body axes, for germline development, and for the elimination of maternal transcripts during the maternal-to-zygotic transition (MZT) when maternal products are replaced by zygotic gene products.
Post-transcriptional control can be coordinated through the partitioning of mRNAs into ribonucleoprotein (RNP) granules. These non-membrane bound bodies serve to compartmentalize regulatory events within the cytoplasm, such as sequestration of translationally repressed mRNAs in stress granules and P bodies (Guzikowski et al., 2019). RNP granules can also promote colocalization and co-regulation of mRNAs that function in the same biological process and the segregation of different mRNAs with conflicting regulatory needs (Buchan, 2014). Such roles in colocalization and segregation are exemplified by granules in the Drosophila germ plasm.
Hundreds of mRNAs are highly enriched in the germ plasm, a specialized cytoplasm that forms at the posterior of the Drosophila oocyte and persists in the early embryo, where it induces the formation of the germ cell progenitors, called pole cells, and specifies germline fate (Jambor et al., 2015; Lécuyer et al., 2007; Mahowald, 2001). Many of these mRNAs are co-packaged in RNP assemblies called germ granules, which coordinate their segregation to the transcriptionally quiescent pole cells (Lerit and Gavis, 2011; Little et al., 2015; Trcek et al., 2015). Among these are mRNAs like nanos that function in germline development (Kobayashi et al., 1996). Not all germ plasm enriched mRNAs are partitioned into germ granules, however. One such transcript, oskar, encodes a core germ granule protein whose synthesis at the posterior of the oocyte initiates germ plasm assembly. Production of Oskar is targeted to the posterior of the oocyte by transport of oskar mRNA to the posterior, where its translation is activated. Oskar protein then nucleates formation of germ granules with the recruitment of additional core granule proteins (Lehmann, 2016). As oogenesis progresses, nanos and numerous other transcripts populate the germ granules (Forrest and Gavis, 2003; Little et al., 2015). By contrast, oskar mRNA is not incorporated into germ granules but forms large RNP bodies, together with the double-stranded RNA-binding protein Staufen, that contain several to hundreds of oskar transcripts (Little et al., 2015). Because germ plasm formation begins with oskar mRNA localization (Lehmann, 2016), we refer to the oskar-containing RNPs in the germ plasm as founder granules. Notably, although both germ granule destined mRNAs and oskar are highly enriched in the germ plasm by virtue of their respective granule association, the localized transcripts represent only a small fraction of their total masses in the embryo (≤4% and 18%, respectively) (Bergsten and Gavis, 1999; Little et al., 2015; Trcek et al., 2015). The majority remain distributed throughout the cytoplasm, where they are silenced by additional layers of post-transcriptional regulation and ultimately degraded during the MZT.
The Drosophila embryo develops as a syncytium in which nuclei undergo 13 rounds of semi-synchronous divisions (nuclear cycles; nc) before the major wave of ZGA and cellularization occur. The pole cells form precociously, however, during nc10 when cell membrane buds enclose nuclei that have entered the germ plasm (Figure 1—figure supplement 1). Whereas both germ granules and founder granules persist in the germ plasm after fertilization, only the germ granules become enriched in the pole cells. This distinction is important, as targeting oskar to germ granules and consequent accumulation of oskar in pole cells impairs germline development, decreasing the number of pole cells formed and disrupting their migration to the gonad during gastrulation (Little et al., 2015). We now report that oskar mRNA itself, and not its capacity to produce Oskar protein, is toxic to pole cells. Such deleterious effects caused by mis-incorporation of oskar in pole cells highlight the need to sequester oskar from germ granule mRNAs within the germ plasm and to prevent its entry into pole cells.
Here, we investigate how incorporation of oskar mRNA into pole cells is minimized, and the role of founder granules in this process. We show that, in contrast to germ granule mRNAs, oskar is degraded in the germ plasm beginning in nc7. This selectivity occurs through the recruitment of mRNA decay factors specifically by founder granules. The subsequent degradation of oskar results in founder granule destabilization and ultimately limits uptake of oskar by pole cells. Founder granules impart another level of spatiotemporal control by promoting oskar degradation in the germ plasm well in advance of the degradation of unlocalized oskar during the MZT. Finally, we demonstrate that degradation of oskar in the germ plasm occurs by a process distinct from the degradation of unlocalized oskar during the MZT and requires the Piwi protein Aubergine. Together, these results show how the fates of different mRNAs with similar localizations can be controlled by their compartmentalization into distinctive RNPs.
Results
Incorporation of untranslatable oskar mRNA into pole cells impairs germline development
The adverse effects on pole cell development caused by forcing oskar into germ granules (Little et al., 2015) could be due to oskar mRNA itself or to an excess of Oskar protein in the pole cells. To distinguish between these possibilities, we targeted an untranslatable oskar transcript to pole cells. oskar mRNA is translated to produce two isoforms, Long Oskar and Short Oskar, using two alternative start codons. We engineered mutations that change both start codons to arginines (M1, 139R) in the osk∆i1,2-nos3′UTR (o∆n) transgene, which expresses oskar mRNA carrying the nanos 3′UTR for germ granule targeting instead of its own localization elements (Figure 1—figure supplement 2A). The o∆n and oM1,139R∆n transcripts were expressed at comparable levels (Figure 1—figure supplement 1B), but only o∆n produced Oskar protein in addition to the endogenous Oskar present in the embryos (Figure 1—figure supplement 2C,D). Similarly to o∆n mRNA, oM1,139R∆n colocalized with germ granules and was incorporated into pole cells (Figure 1A–F).
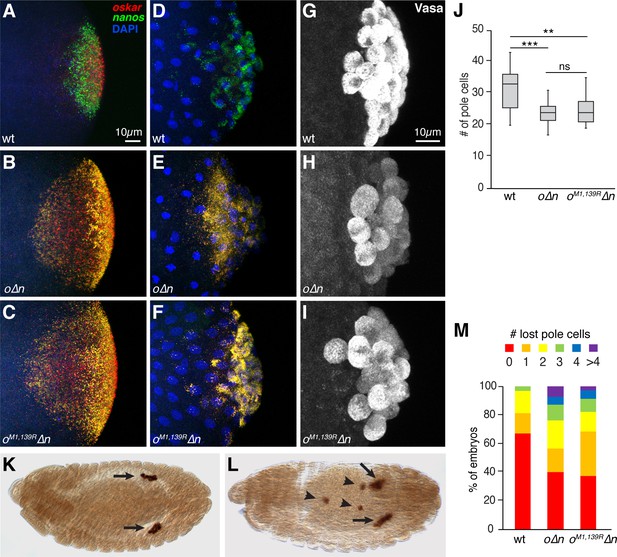
Incorporation of oskar mRNA into pole cells hinders their development independent of translation.
(A–F) Confocal z-series projections of the posterior region of wild-type (wt) embryos (A,D), o∆n embryos (B, E) and oM1,139R∆n (C,F). oskar (red) and nanos (green) mRNAs were detected by FISH in embryos prior to (A–C) and after (D–F) pole cell formation. Both endogenous oskar (in founder granules) and the transgenic oskar transcripts (co-localized with nanos in germ granules) are detected in (B,C,E,F). (G–I) Confocal z-series projections of pole cells, marked by anti-Vasa immunofluorescence, in wt (G), o∆n (H), and oM1,139R∆n (I) embryos. (J) Quantification of the number of pole cells formed in genotypes shown in (G–I), n = 15 embryos each. Box and whisker plot shows median, upper and lower quartiles, and range. (K, L) Anti-Vasa immunostaining of stage 13 oM1,139R∆n embryos showing examples of proper pole cell migration (K) and defective migration (L). Arrows indicated pole cells coalescing into gonads. Arrowheads indicate ‘lost’ pole cells that fail to reach the gonad. (M) Quantification of the frequency of embryos with the indicated numbers of lost pole cells observed in late-stage embryos; n = 91–136 embryos each. **p<0.01, ***p<0.001 as determined by Student's t-test. In this and all subsequent figures, embryos are oriented with the posterior end to the right, dorsal side toward the top.
-
Figure 1—source data 1
Related to Figure 1J and M.
- https://cdn.elifesciences.org/articles/49988/elife-49988-fig1-data1-v2.xlsx
Like embryos from females expressing o∆n, embryos from females expressing oM1,139R∆n formed fewer pole cells than wild-type embryos (Figure 1G–J). The oM1,139R∆n embryos also showed pole cell migration defects similar to those of o∆n embryos, with many pole cells failing to reach the gonad (Figure 1K–M). Thus, mis-incorporation of oskar mRNA itself in germ granules interferes with germline development, independently of Oskar protein production. The toxicity of oskar mRNA could result from titration of one or more factors involved in germline development, similarly to the proposed noncoding function of oskar during oogenesis (Jenny et al., 2006; Kanke et al., 2015). These data strongly suggest the requirement for a mechanism to regulate oskar at the mRNA level in the embryonic germ plasm to prevent its potential threat to germline development.
Founder granules have limited motility and do not disperse during pole cell formation
In wild-type embryos, only low levels of oskar are detected in pole cells (Figure 2A). Our results therefore suggest that segregation of oskar from germ granule mRNAs by packaging into founder granules prevents enrichment of oskar in pole cells, where it impairs their development. We next investigated how this regulation is accomplished. Germ granules become enriched in the pole cells by virtue of their accumulation at centrosomes associated with posterior nuclei. This accumulation occurs by dynein-mediated transport on astral microtubules and triggers the budding of the plasma membrane around the nuclei and associated germ granules to form the pole cells (Lerit and Gavis, 2011). During mid-oogenesis, oskar RNPs are transported to the posterior of the oocyte by kinesin (Brendza et al., 2000; Gáspár et al., 2017). Thus, in contrast to the behavior of germ granules, founder granules might be dispersed by kinesin-mediated transport away from the pole cell nuclei, preventing their accumulation in pole cells.
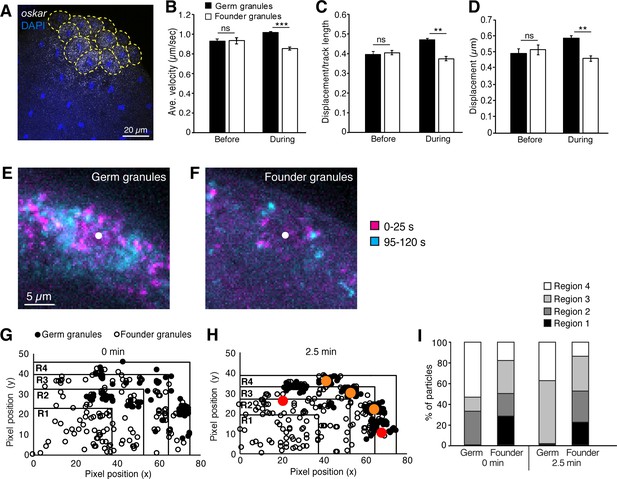
Founder granules are less motile than germ granules during pole cell formation.
(A) Confocal z-series projection showing oskar mRNA in an embryo at nc10. Yellow dashed lines outline pole cells. (B–D) Quantification of germ granule and founder granule motility prior to and during pole cell formation: average velocity (B); linearity (C); and displacement (D). For each time point, three 2 min movies, 491–3221 tracks each, were analyzed. (E, F) Maximum projections of the first 25 s (magenta) and last 25 s (cyan) of a 2 min movie taken during pole cell formation showing the positions of germ granules (E) and founder granules (F) at the start and end of each movie. The white dot indicates the center of the nucleus. Images are cropped to show one pole cell each from Video 1. (G–I) Quantification of the distribution of germ granules and founder granules before and during pole cell formation. Plots of the locations of germ granules (filled circles) and founder granules (open circles) as the onset of (G) and 2.5 min into pole cell formation (H). Red and orange dots indicate approximate positions of nuclei in and out of the frame, respectively. Plots are divided into 4 regions of equal area with region four closest to the cortex and region one farthest from the cortex. The number of each type of granule within each region is quantified in (I); n = 108–248 particles. Values are mean ± s.e.m.; **p<0.01; ***p<0.001 as determined by Student's t-test.
-
Figure 2—source data 1
Related to Figure 2B.
- https://cdn.elifesciences.org/articles/49988/elife-49988-fig2-data1-v2.xlsx
-
Figure 2—source data 2
Related to Figure 2C.
- https://cdn.elifesciences.org/articles/49988/elife-49988-fig2-data2-v2.xlsx
-
Figure 2—source data 3
Related to Figure 2D.
- https://cdn.elifesciences.org/articles/49988/elife-49988-fig2-data3-v2.xlsx
-
Figure 2—source data 4
Related to Figure 2G–I.
- https://cdn.elifesciences.org/articles/49988/elife-49988-fig2-data4-v2.xlsx
To address this possibility, we compared the behavior of founder granules marked with Staufen-GFP and germ granules marked with Vasa-mCherry in live embryos. As previously observed (Lerit and Gavis, 2011), germ granules exhibited directed motility toward embryonic nuclei at the onset of pole cell formation. By contrast, most founder granules appeared to jiggle in place (Video 1). Tracking of both germ granules and founder granules showed that founder granules moved more slowly, with smaller displacements, and with fewer linear trajectories than germ granules (Figure 2B–D). Whereas germ granules became clustered around nuclei as a result of their directed motility, the less motile founder granules remained uniformly distributed in the germ plasm (Figure 2E–I). Consequently, founder granules were detected among the germ granules clustered around nuclei (Figure 2F,H) and near the cortex (Figure 2H,I), suggesting that they can be passively incorporated in pole cells. Moreover, since founder granules do not disperse from the vicinty of the forming pole cells, uptake of oskar by pole cells must be limited by another mechanism.
Behavior of germ granules and founder granules prior to pole cell formation.
Time-lapse movie of germ granules labeled with Vasa-mCherry (green) and founder granules labeled with GFP-Staufen (red). The movie starts as nuclei have reached the posterior of the embryo and induce release of granules from the cortex. Embryo is oriented with the posterior toward the top of the frame. Images were captured at five frames/second for a total of 600 frames and the movie is shown at 100 frames/second.
Germ plasm-localized oskar is degraded during pole cell formation
In our live imaging experiments, we observed an apparent decrease in the number of founder granules as pole cells formed, suggesting they were destabilized. To further investigate this behavior, we visualized founder granule and germ granule constituent mRNAs, oskar and nanos respectively, before, during, and after pole cell formation by quantitative fluorescence in situ hybridization (FISH). As measured by total fluorescence intensity, the amount of oskar mRNA in the germ plasm decreased by 98% from before pole cell formation to after pole cell formation (Figure 3A,C). Consistent with previous studies showing that germ granule mRNAs are maintained in the germ plasm throughout pole cell formation (Bashirullah et al., 2001), there was no significant change in the amount of nanos (Figure 3B,C). We also compared the behavior of oskar targeted to germ granules with that of oskar in founder granules. To discrimminate germ granule associated oskar from endogenous oskar in the same embryos, we used an osk∆i-sfgp-nos3'UTR transgene (o∆gn), in which oskar is tagged with superfolder gfp sequences and compared it to a control osk-sfgfp transgene (og). FISH analysis showed that the amount of germ plasm localized og mRNA decreased by 90% from before to after pole cell formation, whereas o∆gn mRNA remained largely unchanged (Figure 3—figure supplement 1). These results are consistent with the specific degradation of founder granule associated oskar mRNA during pole cell formation.
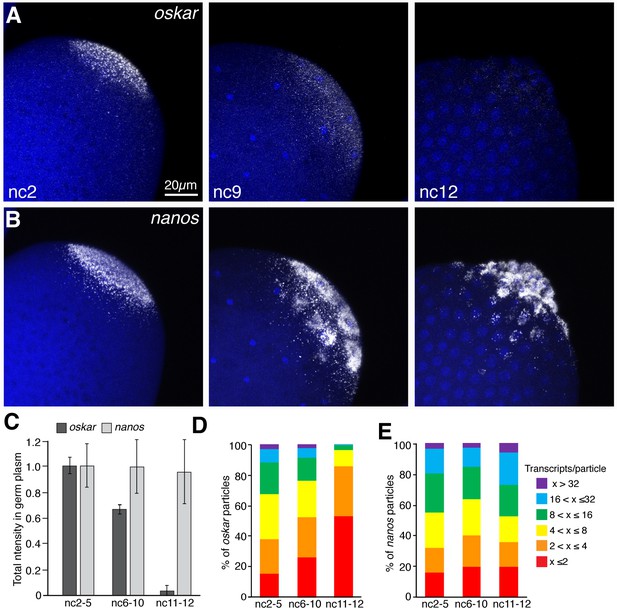
oskar mRNA is preferentially degraded during pole cell formation.
(A,B) Confocal z-series maximum intensity projections of the posterior pole of Drosophila embryos at nc2, nc9, and nc12, with oskar (A) or nanos (B) mRNAs detected by FISH. By nc9, nuclei have arrived at the cortex and by nc12, pole cells have formed. (C) Quantification of average total fluorescence intensities of germ plasm localized oskar and nanos during nc2-5, nc6-10, and nc11-12; n = 7–10 embryos per time period for oskar, n = 5 embryos per time period for nanos. Values are mean ± s.d., normalized to the values at nc2-5 for each mRNA. (D,E) Quantification of the size distributions of founder granules (D) and germ granules (E) as measured by the number of oskar or nanos transcripts per particle detected, respectively, from nuclear cycles 2 through 12; (n = 4–20 embryos per time period for germ granules, n = 11–28 embryos per time period for founder granules). Values are mean ± s.d.; ***p<0.001 for oskar values at nc6-9 and nc11-12 compared to nc2-5 as determined by Student's t-test.
-
Figure 3—source data 1
Related to Figure 3C.
- https://cdn.elifesciences.org/articles/49988/elife-49988-fig3-data1-v2.xlsx
-
Figure 3—source data 2
Related to Figure 3D,E.
- https://cdn.elifesciences.org/articles/49988/elife-49988-fig3-data2-v2.xlsx
mRNA degradation typically involves removal of the 5’ cap followed by exonuclease digestion. In Drosophila, decapping is carried out by Decapping Protein 2 (DCP2) and its cofactor Decapping Protein 1 (DCP1) (Siwaszek et al., 2014). To confirm that oskar is degraded in the germ plasm, we visualized DCP1 by immunofluorescence together with oskar by FISH in embryos from nc2 to nc12. Within the germ plasm, the frequency of detecting a particle containing one or more oskar transcripts colocalized with DCP1 began to increase at nc5 and continued to increase through pole cell formation (Figure 4A–C and data not shown). Consistent with the differential stability of oskar and germ granule mRNAs in the germ plasm, we did not detect colocalization of DCP1 with a representative germ granule mRNA, cyclin B (Figure 4—figure supplement 1). In addition, we found that 77% and 49% of oskar particles that colocalized with DCP1 also colocalized with the decapping complex associated protein Me31B (Figure 4D,D',F) and the 5' to 3' exonuclease Pacman (Figure 4E,E',G), respectively. Temporal analysis showed that recruitment of Pacman was delayed relative to DCP1 (Figure 4C), indicating that the degradation machinery is recruited in a sequential manner. Notably, DCP1 did not colocalize with o∆gn mRNA in germ granules (Figure 4H–I), suggesting that the degradation machinery is specifically recruited to founder granule associated oskar. We conclude that oskar degradation dramatically reduces the amount of oskar mRNA available for incorporation into pole cells.
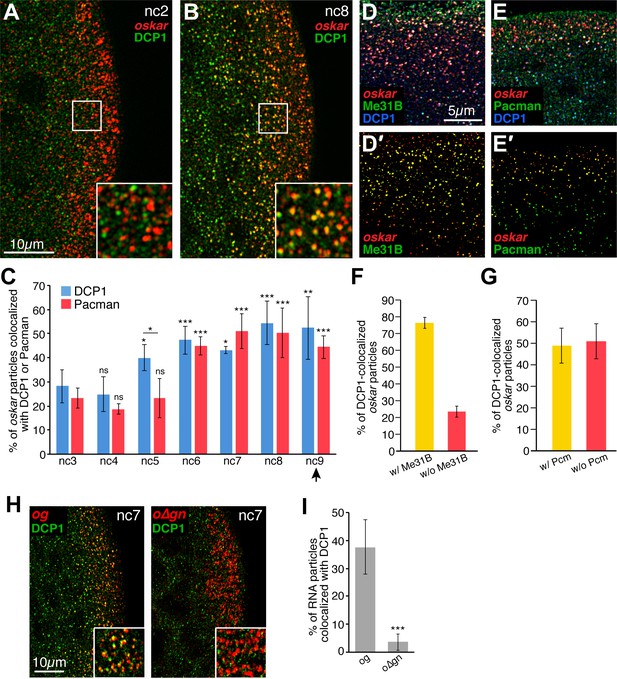
Founder granules associate with decapping and degradation factors in the germ plasm in advance of pole cell formation.
(A,B) Confocal z-series projections of the posterior of nc2 (A) and nc8 (B) embryos. Anti-DCP1 immunofluorescence (green) was performed together with FISH for oskar mRNA (red). Enlargements of the boxed germ plasm regions show colocalization of oskar and DCP1 in particles at nc8 but not at nc2. (C) Quantification of the proportion of oskar particles detected that also contain DCP1 (i.e., are colocalized with DCP1; blue) or Pacman (red) from nc3 to nc9; n = 5–8 embryos for each nc. Arrowhead indicates time of pole cell formation. (D,D',E,E') Confocal z-series projections showing oskar mRNA detected by FISH (red) together with DCP1 immunofluorescence (blue) and either direct Me31B-EGFP fluorescence (green; D,D') or Pacman immunofluorescence (green; E,E') in the germ plasm of nc7 embryos. Images in (D',E') are masked by the DCP1 channel so that only the oskar and either Me31B (D') or Pcm (E') fluorescence signals that overlap with the DCP1 fluorescence signals are visible. (F,G) Quantification of the proportion of oskar particles colocalized with DCP1 and Me31B (F) or DCP1 and Pacman (Pcm; G) as compared to the proportion colocalized only with DCP1 in the same images; n = 7 embryos each for Pacman, n = 5 embryos each for Me31B. (H) Confocal z-series projections of the posterior of og and o∆gn embryos at nc 7. Anti-DCP1 immunofluorescence (green) was performed together with FISH to detect sfgfp sequences (red). Enlargements of the boxed germ plasm regions show colocalization of DCP1 with og but not o∆gn RNA particles in the germ plasm. (I) Quantification of the proportion of og and o∆gn RNA particles detected that also contain DCP1; n = 8 embryos each. Values are mean ± s.d.; **p<0.01, ***p<0.001 as determined by Student's t-test. In (C) each DCP1 or Pacman is compared to the corresponding value at nc3. DCP1 and Pacman differ significantly at nc5 (p=0.001).
-
Figure 4—source data 1
Related to Figure 4C.
- https://cdn.elifesciences.org/articles/49988/elife-49988-fig4-data1-v2.xlsx
-
Figure 4—source data 2
Related to Figure 4F,G,I.
- https://cdn.elifesciences.org/articles/49988/elife-49988-fig4-data2-v2.xlsx
oskar mRNA is degraded within founder granules
We next considered what accounts for the specific vulnerability of oskar in the germ plasm. In addition to oskar mRNA levels, the sizes of founder granules, as measured by the number of oskar mRNAs they contain (see Materials and methods), decreased over the course of pole cell formation (Figure 3D). By contrast, germ granule size distributions, measured by the number of nanos transcripts they contain, were unchanged (Figure 3E). Two scenarios are consistent with the observed decrease in founder granule size. In one, founder granules disassemble, leaving oskar accessible to the degradation machinery. In contrast, other mRNAs that remain packaged in RNP granules, like those in germ granules, would remain protected from the degradation machinery in the germ plasm. Alternatively, founder granules could serve as the site of oskar degradation, thereby compartmentalizing the activity of the degradation machinery to preserve germ granule mRNAs.
To distinguish whether founder granule disassembly occurs before or after oskar degradation, we compared the timing of: 1) oskar association with degradation factors; 2) changes in total oskar levels; and 3) changes in founder granule composition from nc2 to nc12. As described above, colocalization of oskar with DCP1 first began to increase at nc5 (Figure 4C). The amount of oskar in the germ plasm decreased subsequently at nc7 (Figure 5A), and the average size of oskar particles declined sharply at nc9, when pole cells form (Figure 5B). The amount of Staufen in founder granules, measured by immunofluorescence intensity, began to decrease at nc11 (Figure 5C) along with a dramatic decrease in the percentage of Staufen particles that remained associated with oskar (Figure 5D). This order of events is consistent with association of the degradation factors with intact founder granules leading to oskar degradation, followed by the destabilization of founder granules. Furthermore, the percentage of oskar particles colocalized with Staufen remained unchanged until nc10, when there was a small decrease in colocalization (Figure 5E).
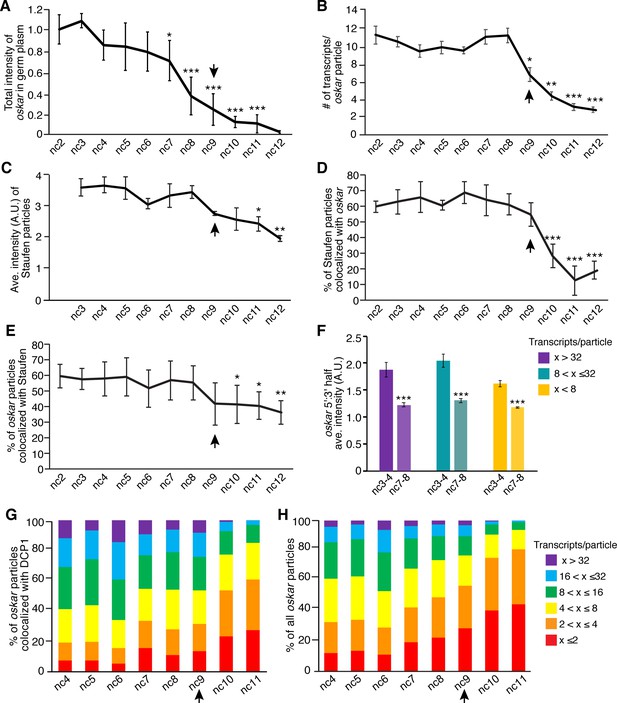
Founder granule disassembly follows oskar degradation.
(A) Quantification of total fluorescence intensity of oskar in the germ plasm from nc2 to nc12 normalized to the average intensity at nc2; n = 5–13 embryos for each nc. (B) Quantification of the average number of mRNAs per detected oskar particle from nc2 to nc12; n = 4–10 embryos for each nc. (C) Quantification of the average fluorescence intensity (size) of Staufen particles in the germ plasm from nc3 to nc12; n = 3–8 embryos for each nc. (D) Quantification of the proportion of detected Staufen particles colocalized with oskar from nc2 to nc12; n = 4–10 embryos for each nc. (E) Quantification of the proportion of detected oskar particles colocalized with Staufen from nc2 to nc12; n = 4–10 embryos for each nc. (F) Ratio of the average fluorescence intensities for 5' and 3' oskar probes measured particles of the indicated sizes in nc3-4 versus nc7-8 embryos (n = 10 embryos each time period). (G,H) Proportion of oskar particles in the germ plasm containing the indicated number of mRNAs that are colocalized with DCP1 (G) and proportion of all oskar particles in the germ plasm with the indicated number of mRNA (H); n = 6–9 embryos for each nc. Values in (A), (D), and (E) are mean ± s.d; values in (B,C,F) are mean ± s.e.m. Student's t-tests were performed comparing each nuclear cycle to nc2 (A,B,D,E), nc3 (C), and nc3-4 (F); *p<0.05, **p<0.01, ***p<0.001 as determined by Student's t-test. Arrowheads indicates time of pole cell formation.
-
Figure 5—source data 1
Related to Figure 5A,B.
- https://cdn.elifesciences.org/articles/49988/elife-49988-fig5-data1-v2.xlsx
-
Figure 5—source data 2
Related to Figure 5C–E.
- https://cdn.elifesciences.org/articles/49988/elife-49988-fig5-data2-v2.xlsx
-
Figure 5—source data 3
Related to Figure 5F.
- https://cdn.elifesciences.org/articles/49988/elife-49988-fig5-data3-v2.xlsx
-
Figure 5—source data 4
Related to Figure 5G,H.
- https://cdn.elifesciences.org/articles/49988/elife-49988-fig5-data4-v2.xlsx
In addition, we analyzed DCP1 association with founder granules containing both oskar mRNA and Staufen. Staufen and DCP1 were detected by immunofluorescence together with oskar mRNA by FISH and the frequencies of colocalization were quantified. This analysis revealed that 35.0 ± 5.9% of Staufen particles that colocalized with oskar were also colocalized with DCP1 at nuclear cycle 8 (data not shown), consistent with the measurement for oskar mRNA (Figure 4C). Of the DCP1 particles colocalized with Staufen, 90.0 ± 5.4% were also colocalized with oskar (data not shown). Thus, DCP1 associates with intact founder granules. We also analyzed the sizes of oskar particles that colocalized with DCP1. If founder granules disassemble prior to oskar degradation, DCP1 should preferentially colocalize with small oskar particles. However, we found that DCP1 associated with oskar particles of all sizes (Figure 5G,H) suggesting that oskar mRNA is not released from founder granules prior to the onset of degradation.
Finally, we took advantage of the 5' to 3' directionality of mRNA degradation to monitor the integrity of oskar in founder granules before (nc3-4) and after (nc7-8) degradation ensues by performing FISH with differentially labeled probes targeting either the 5' or 3' half of the oskar transcript. The ratio of the fluorescence intensities of the 5' and 3' probes (5':3') was then determined for individual oskar particles within the germ plasm. For both larger and smaller particles, the 5':3' ratio decreased significantly between nc3-4 and nc7-8, reflecting the loss of sequences from the 5' end of oskar (Figure 5F). Together, the data presented here support a model in which oskar degradation occurs within founder granules.
oskar mRNA degradation in the germ plasm is distinct from degradation of unlocalized oskar
Widespread clearance of maternal mRNAs occurs during the MZT and is controlled by both maternal and zygotic degradation factors. The maternal decay machinery is set in motion by egg activation whereas the zygotic pathway is engaged following ZGA (Laver et al., 2015b). Genome-wide microarray analysis showed that oskar is degraded during the MZT by both maternal and zygotic pathways (Thomsen et al., 2010). However, because only 18% of oskar mRNA in the embryo is localized to the germ plasm and packaged in founder granules (Bergsten and Gavis, 1999; Little et al., 2015), this analysis predominantly measured the unlocalized population of oskar mRNA in the bulk embryonic cytoplasm. Moreover, previous studies differ in their estimates of the onset of maternal mRNA degradation (Despic and Neugebauer, 2018; Laver et al., 2015b). Therefore, we sought to determine how degradation of oskar in the germ plasm relates to degradation of oskar during the MZT.
As a first step, we measured the change in the amount of oskar per volume of germ plasm and the change in the amount of oskar per volume of bulk cytoplasm in embryos from nc2 to nc12 (Figure 6A,B). The first significant decrease in germ plasm localized oskar occurred at nc7 (Figure 6C), consistent with our analysis of the total amount of localized oskar (Figure 5A). For unlocalized oskar, the first significant decrease occurred at nc11. Consistent with the temporal difference in degradation of the two oskar populations, association of unlocalized oskar with DCP1 is delayed relative to germ plasm localized oskar (Figure 6D,E). Together, these results indicate that degradation of oskar in the germ plasm begins well in advance of degradation of oskar during the MZT.
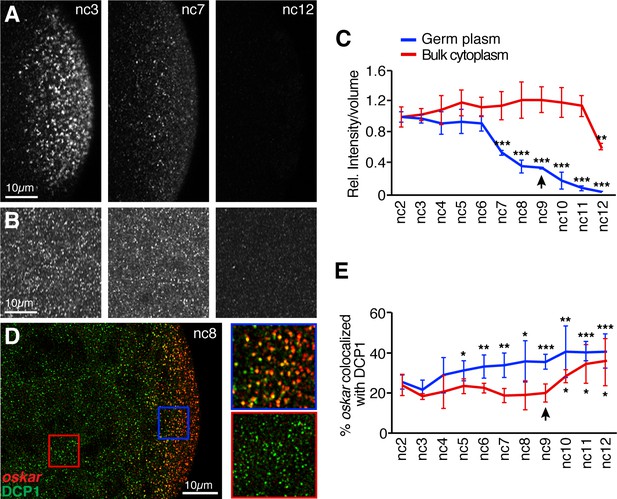
oskar mRNA is degraded earlier in the germ plasm than in the bulk cytoplasm.
(A,B) Confocal z-series projections taken at posterior pole (germ plasm, A) or the middle region (bulk cytoplasm, B) of embryos at nc3, nc7, and nc12 with oskar mRNA detected by FISH. Images in (B) were captured with higher laser power than images in (A) in order to detect small oskar RNPs (see Materials and methods). (C) Quantification of oskar FISH fluorescence intensity per volume in the germ plasm and bulk cytoplasm from nc2 to nc12 normalized to the value at nc2. The first significant changes from nc2 in the germ plasm and bulk cytoplasm occur at nc7 and nc12, respectively; n = 5 embryos for each nc. (D) Confocal z-series projection encompassing both the bulk cytoplasm and germ plasm. Anti-DCP1 immunofluorescence (green) was performed together with FISH for oskar mRNA (red). Enlargements of the boxed regions show colocalization of oskar and DCP1 in the germ plasm but not in the bulk cytoplasm. (E) Quantification of the proportion of detected oskar particles colocalized with DCP1 in the bulk cytoplasm and in the germ plasm; n = 6–9 embryos for each nc. Values are mean ± s.d. Student's t-tests were performed comparing each nuclear cycle to nc2 for the same region of the embryo; *p<0.05, **p<0.01, ***p<0.001. Asterisks for bulk cytoplasm data points are positioned below the error bars for visibility. Arrowheads indicates time of pole cell formation.
-
Figure 6—source data 1
Related to Figure 6C.
- https://cdn.elifesciences.org/articles/49988/elife-49988-fig6-data1-v2.xlsx
-
Figure 6—source data 2
Related to Figure 6E.
- https://cdn.elifesciences.org/articles/49988/elife-49988-fig6-data2-v2.xlsx
Next, we asked whether oskar degradation in the germ plasm is initiated by the same factors that act later during the MZT. Two RNA-binding proteins, Brain tumor and Pumilio have been shown to direct clearance of oskar and numerous other maternal mRNAs in the bulk cytoplasm during the MZT (Laver et al., 2015a). The Piwi protein Aubergine has also been implicated (Barckmann et al., 2015). We therefore asked whether these proteins also regulate degradation of oskar in the germ plasm. In brain tumor and pumilio mutant embryos, oskar was degraded in the germ plasm similarly to wild-type embryos (Figure 7A,B,D and data not shown) indicating that Brain tumor and Pumilio do not target oskar for degradation in the germ plasm as they do in the bulk cytoplasm. However, oskar was partially stabilized in the germ plasm of aubergine mutant embryos (Figure 7A,C,D). Because most aubergine mutant embryos arrest development prior to pole cell formation, we assessed oskar degradation in wild-type unfertilized eggs and found that oskar was degraded independently of progression through embryogenesis (Figure 7—figure supplement 1). Thus, oskar degradation requires only maternal factors and the stabilization of oskar observed in aubergine mutant embryos is not simply a consequence of their developmental arrest. Moreover, we found that DCP1 and oskar did not colocalize in the germ plasm of aubergine mutant embryos (Figure 7E–G). Introduction of a functional gfp-aub transgene into aubergine mutant females rescued both oskar degradation and DCP1 colocalization (Figure 8). These results suggest a role for Aubergine in recruitment of DCP1 to founder granules, resulting in decapping and subsequent degradation of oskar. In addition, oskar particle sizes were stabilized in the germ plasm of aubergine mutants (Figure 7H,I), consistent with oskar degradation causing founder granule destabilization.
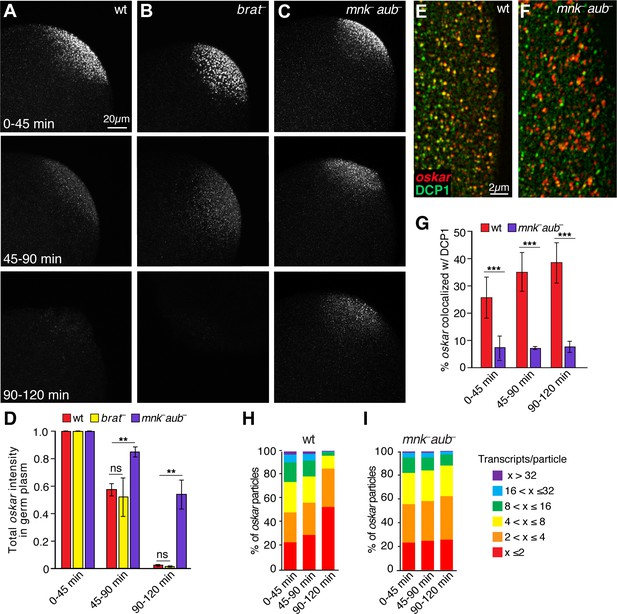
Aubergine is required for oskar degradation in the germ plasm.
(A–C) Confocal z-series projections of the germ plasm of wild-type (wt; A), brain tumor mutant (brat–; B), and aubergine mutant (mnk– aub–; C) embryos with oskar mRNA detected by FISH. The mnk mutation (in chk2) bypasses the indirect effect of aubergine mutants on oskar translation during oogenesis and consequent defects in oskar mRNA localization (Becalska et al., 2011; Klattenhoff et al., 2007); see Materials and methods). (D) Quantification of total oskar fluorescence intensity in the germ plasm in wt, mnk– aub– and brat–embryos. Three biological replicates were performed, each with n = 5–10 embryos per time period for wt; n = 3–7 embryos for mnk– aub–; n = 3–8 embryos for brat–. (E,F) Confocal z-series projections of a region of the germ plasm in age matched wt (E) and mnk– aub– (F) embryos; oskar detected by FISH (red) with anti-DCP1 immunofluorescence (green). (G) Quantification of colocalization of oskar and DCP1 in wt and mnk– aub– embryos (n = 5–44 embryos each time period). (H,I) Quantification of the proportion of detected oskar particles with the indicated number of mRNAs per particle number in the germ plasm of wt (H) and mnk– aub– (I) embryos; (n = 4–5 embryos each time period for mnk– aub–, n = 11–28 embryos for wt). Values are mean ± s.e.m., normalized to the value at 0–45 min for each genotype (D) and mean ± s.d. (G); *p<0.05, **p<0.01, ***p<0.001 as determined by Student's t-test.
-
Figure 7—source data 1
Related to Figure 7D.
- https://cdn.elifesciences.org/articles/49988/elife-49988-fig7-data1-v2.xlsx
-
Figure 7—source data 2
Related to Figure 7G.
- https://cdn.elifesciences.org/articles/49988/elife-49988-fig7-data2-v2.xlsx
-
Figure 7—source data 3
Related to Figure 7H,I.
- https://cdn.elifesciences.org/articles/49988/elife-49988-fig7-data3-v2.xlsx
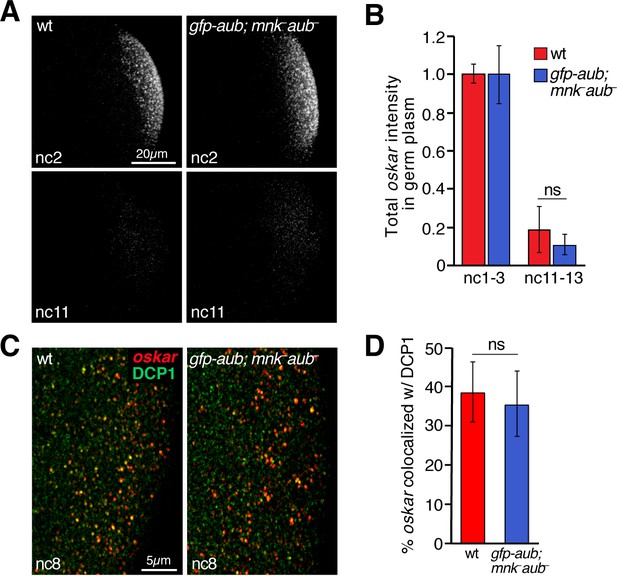
Rescue of oskar degradation and DCP1 recruitment by gfp-aub.
(A) Confocal z-series projections of the germ plasm of wild-type (wt) embryos and aubergine mutant embryos expressing gfp-aub (gfp-aub; mnk– aub–), with oskar mRNA detected by FISH. (B) Quantification of oskar fluorescence intensity in the germ plasm in wt and gfp-aub; mnk– aub– embryos (n = 7–11 embryos per time period). (C) Confocal z-series projections of a region of the germ plasm in age matched wt and gfp-aub; mnk– aub– embryos; oskar detected by FISH (red) with anti-DCP1 immunofluorescence (green). (D) Quantification of colocalization of oskar and DCP1 in wt and gfp-aub; mnk– aub– embryos (n = 5 embryos each). Values are mean ± s.d.; ***p<0.001 as determined by Student's t-test.
-
Figure 8—source data 1
Related to Figure 8B,D.
- https://cdn.elifesciences.org/articles/49988/elife-49988-fig8-data1-v2.xlsx
Discussion
mRNAs that accumulate in the Drosophila germ plasm during oogenesis are partitioned into at least two types of RNPs, germ granules and founder granules. Germ granules contain mRNAs necessary for germline development and promote the inheritance of these mRNAs by pole cells. Founder granules contain oskar mRNA, whose translation at the posterior of the oocyte is crucial for germ plasm assembly and germ granule formation but whose accumulation in pole cells is detrimental to germline development. We show that oskar RNA itself is toxic to pole cells, necessitating an early-acting mechanism to deplete oskar from the germ plasm. Our results indicate that founder granules minimize inheritance of oskar by pole cells by recruiting factors for compartmentalized oskar degradation.
mRNA degradation is a key feature of early embryonic development, with the majority of the maternal transcriptome turned over and replaced by the zygotic transcriptome during the MZT. Founder granule-associated oskar degradation is distinct from degradation of unlocalized oskar, nanos, and other maternal transcripts during the MZT both temporally and mechanistically. Degradation of oskar in the germ plasm occurs well in advance of pole cell formation whereas the majority of MZT-related mRNA degradation occurs after pole cells have formed. Consistent with this timing, oskar degradation in the germ plasm is mediated solely by maternal factors whereas oskar degradation in the bulk cytoplasm relies on both maternal and zygotic machineries (Thomsen et al., 2010). Unlocalized oskar and nanos are degraded with the same kinetics (Thomsen et al., 2010) and degradation factors (Laver et al., 2015a) during the MZT. By contrast, only oskar is degraded in the germ plasm and this degradation does not require Brain tumor or Pumilio, but depends in part on Aubergine. Moreover, redirecting oskar to germ granules results in its stabilization, akin to nanos mRNA. These results, together with evidence that oskar degradation precedes founder granule disassembly, indicate that the differential suceptibility of oskar and germ granule mRNAs in the germ plasm is imparted by properties of the granules in which they reside.
The selective degradation of oskar among the hundreds of different mRNAs that share the germ plasm derives from the exclusive ability of founder granules to recruit decapping and degradation machinery. An outstanding question is what triggers the recruitment of DCP1 to founder granules around nuclear cycle 5. Such a timer could be provided by an as yet unidentified protein whose translation is activated at fertilization and reaches a critical threshold by nuclear cycle 5, similar to temporal control conferred by Smaug during the MZT (Benoit et al., 2009). Although DCP1 recruitment requires Aubergine, Aubergine protein is maternally contributed suggesting that it is not the timer (Kronja et al., 2014). The requirement for Aubergine in oskar degradation is itself surprising. First, Aubergine is a core germ granule protein and has been shown to stabilize germ granule mRNAs through an interaction with the poly(A) polymerase Wispy (Dufourt et al., 2017). Second, Aubergine has been proposed to mediate degradation of mRNAs during the MZT by both piRNA-mediated cleavage and recruitment of the RNA-binding protein Smaug and the CCR4-NOT deadenylase complex (Barckmann et al., 2015). oskar degradation is Smaug-independent, however (Tadros et al., 2007). Whether Aubergine might also direct piRNA-mediated degradation of oskar in founder granules remains a question for future study but its requirement for association of DCP1 with founder granules suggests that piRNA-mediated degradation may not be the primary mode. Moreover, we did not detect colocalization of Aubergine with founder granules suggesting that Aubergine acts indirectly, perhaps by sequestering stability factors. Alternatively, cytoplasmic Aubergine might associate with founder granules only transiently or in small quantities.
Compartmentalization of oskar degradation in founder granules may also serve to increase efficacy. The concentration of up to hundreds of oskar molecules in founder granules may enhance interaction of oskar with the decapping and degradation machinery, increasing reaction rates so that the germ plasm load of oskar is sufficiently reduced prior to pole cell formation. Moreover, as non-membrane bound organelles, founder granules would create a hub, bringing the various components of the decapping and degradation machinery into close proximity. Our finding that founder granules are stabilized when oskar is stabilized, as in aubergine mutants, suggests that oskar RNA might regulate the properties of these granules, similarly to the role of RNA in dictating the physical properties of liquid droplets in vitro and in Ashbya cells in vivo (Zhang et al., 2015).
Co-packaging in RNPs can facilitate the coordinated regulation of cohorts of mRNAs. Germ granules serve such a role by coordinating the delivery of mRNAs to pole cells. Do founder granules contain mRNAs in addition to oskar that are toxic to pole cells? Interestingly, under certain conditions, retrotransposon mRNAs can mimic oskar and localize to the germ plasm using the same transport machinery (Tiwari et al., 2017). Founder granules might also therefore sequester and destroy retrotransposon mRNAs in the germ plasm, preventing their reinsertion into the genome and thus maintaining genome integrity. We suggest that whereas germ granules provide the pole cells with a maternal endowment needed for germline development, founder granules serve an opposing function to protect the germline from inheriting deleterious transcripts.
Materials and methods
Drosophila strains and genetics
Request a detailed protocolThe following D. melanogaster alleles and transgenes were used: y1, w67c23 (Bloomington Drosophila Stock Center [BDSC] 6599) as the wild-type strain; osk54 (Lehmann and Nüsslein-Volhard, 1991), oskA87 (Vanzo and Ephrussi, 2002), FRT40 brat11 (Frank et al., 2002); mnkP6, aubHN and mnkP6, aubQC (Klattenhoff et al., 2007); pumET3 (also called pum3) (Barker et al., 1992); pumMsc (Barker et al., 1992); mCherry-vas (Lerit and Gavis, 2011); GFP-stau (Schuldt et al., 1998); Me31B-EGFP (Buszczak et al., 2007); UAS-gfp-aub (Harris and Macdonald, 2001). brat11 germline clones were generated by the autosomal FLP-DFS method (Chou and Perrimon, 1996). Double mutants for aub and chk2 (mnk) were used to bypass the indirect effect of aubergine mutants on oskar translation during oogenesis and consequent defects in oskar mRNA localization (Becalska et al., 2011; Klattenhoff et al., 2007). UAS-gfp-aub was expressed using the nos-Gal4-VP16 driver (Van Doren et al., 1998) recombined on the same chromosome (gift of P. Macdonald). The matα-Gal4-VP16 driver (BDSC 7063) was used to express the UASp-osk∆i1,2-nos3′UTR (Little et al., 2015) and UASp-oskM1,139R∆i1,2-nos3′UTR transgenes (this study) in females that also express endogenous, wild-type oskar. All genotypes described in the text refer to the maternal genotype, that is the genotype of the mother that generated the embryos analyzed.
Transgene construction
Request a detailed protocolThe pattB-UASp-oskM1,139R∆i1,2-nos3′UTR transgene plasmid was generated from pUASp-osk∆i1,2-nos3′UTR (Little et al., 2015) by using site-directed mutagenesis to change the start codons for both Long and Short Oskar protein from ATG to CGC. The UASp-osk∆i1,2-nos3′UTR and UASp-oskM1,139R∆i1,2-nos3′UTR sequences were then inserted into the pattB vector (http://www.flyc31.org/sequences_and_vectors.php). The pattB-osk-sfgfp and pattB-osk∆i1,2-sfgfp-nos3'UTR transgene plasmids are based on the osk::gfp (p2368) construct, which contains an 8 kb oskar rescue fragment (Snee et al., 2007). The gfp sequence fused to the 3' end of oskar in osk::gfp was replaced by the Drosophila codon optimized sequence encoding superfolder GFP (sfgfp) used in the FlyFos phosmid library (Sarov et al., 2016). pattB-osk∆i1,2-sfgfp-nos3'UTR also contains sequences encoding a 2xTy1 epitope tag before the sfgfp sequence (Sarov et al., 2016). All four transgenes were integrated into the attP40 site by phiC31-mediated recombination.
Embryo collection and fixation
Request a detailed protocolEmbryos were collected on apple juice agar plates at room temperature or in a 25°C incubator and staged by nuclear cycle (nc) when possible. When staging by nc was not possible, embryos were collected in a 25°C incubator and staged according to time after egg laying (0–45 min = nc 1–5, 45–90 min = nc 6–10, 90–135 min = nc 11–13). Embryos were dechorionated, fixed, and devitellinized as described in Abbaszadeh and Gavis (2016), then stored in methanol at −20°C for up to 1 month. Embryos used for RT-qPCR were dechorionated, flash frozen in liquid nitrogen, and stored at −80°C.
Single molecule FISH (smFISH)
Request a detailed protocolsmFISH probe sets consisting of 20 nt long oligonucleotides with two nt spacing complementary to oskar (CG10901; 99 oligos), nanos (CG5637; 63 oligos), cyclin B (CG3510; 48 oligos), or sfgfp (31 oligos) were designed with the Stellaris Probe Designer (LGC Biosearch Technologies). For oskar, nanos, and cyclinB, oligonucleotides with a 3′ amine modification were obtained from Biosearch Technologies, conjugated to Atto 647N or Atto 565 dye (Sigma-Aldrich), then purified by HPLC as previously described (Raj et al., 2008). To visualize the 5' and 3' halves of oskar, 48 oligos from the complete probe set covering the 5' half of oskar were coupled to Atto 565 dye and 48 oligos covering the 3' half were coupled to Atto 647N dye. The sfgfp probe set was purchased already conjugated to Quasar 670 dye (Biosearch Technologies). smFISH was performed as described in Abbaszadeh and Gavis (2016). Embryos were mounted under #1.5 glass coverslips (VWR) in Vectashield Mountant (Vector Laboratories) for quantification of total localized fluorescence intensity or Prolong Diamond Antifade Mountant (Thermo Fisher Scientific) for colocalization and particle intensity analyses.
Immunofluorescence
Request a detailed protocolEmbryos were rehydrated stepwise into PBST (PBS, 0.1%Tween-20 [Sigma-Aldrich]) and then blocked in Image it FX (Thermo Fisher Scientific) for 30 min at room temperature followed by washing 3 × 15 min in PBHT (PBS, 0.1% Tween-20, 0.25 mg/ml heparin [Sigma-Aldrich], 50 µg/ml tRNA [Sigma-Aldrich]). Embryos were then incubated in primary antibody diluted in PBHT over night at 4°C with rocking. Primary antibodies: 1:2000 rabbit anti-Staufen (St Johnston et al., 1991), 1:1000 mouse anti-DCP1 (Miyoshi et al., 2009), 1:500 rabbit anti-Pacman #428 (Grima et al., 2008), 1:2000 rabbit anti-Vasa (Liang et al., 1994), 1:500 rabbit anti-GFP-Alexa 488 (Invitrogen). Embryos were washed sequentially for 5, 10 and 20 min in PBHT at room temperature, then incubated with the appropriate secondary antibody diluted in PBHT in the dark for 2 hr at room temperature with rocking. Secondary antibodies: 1:1000 goat anti mouse-Alexa 568 (Thermo Fisher Scientific), 1:1000 goat anti mouse-Alexa 488 (Thermo Fisher Scientific), 1:500 goat anti rabbit-Alexa 568 (Thermo Fisher Scientific). Embryos were washed 3 × 10 min in PBST at room temperature with rocking followed by 2 min incubation in 1.25 µg/ml DAPI in PBST and rinsed four times with PBST. The embryos were then mounted as described above. For double immunofluorescence/smFISH experiments, embryos were refixed in 4% PFA for 30 min at room temperature with rocking and rinsed 4 × with PBST before proceeding with smFISH as described above.
Quantification of fluorescence intensity
Request a detailed protocolConfocal imaging was performed using a Leica SP5 laser scanning microscope with a 63 × 1.4 NA oil immersion objective and GaAsP ‘HyD’ detectors in standard detection mode except for the experiments shown in Figure 3—figure supplement 1, Figure 4H,I and Figure 8C,D, which were imaged on a Nikon A1 laser scanning microscope with a 60 × 1.4 NA oil immersion objective and GaAsP detectors. All imaging parameters were kept identical within each experiment. For quantification of total intensity, z-series with a 2 µm step size were used to capture the germ plasm-localized signal. Image processing and analysis were done in FIJI. Z-projections were made with the ‘sum slices’ function and the threshold adjusted so the entire localized signal was included. The total fluorescence intensity of the localized signal (integrated density function in FIJI) was then measured. The average total intensity for each nc or time period was normalized to the average intensity for the earliest nc or time period in the experiment.
To quantify the fluorescence intensity of RNA within the germ plasm (localized) vs RNA within the bulk cytoplasm (unlocalized), two images were captured for each embryo: one at lower laser power optimized for the brighter germ plasm RNA signal and one at higher laser power optimized for the unlocalized RNA signal. Image processing and analysis were done in FIJI. Z-projections spanning 12 µm (6 µm above and below the mid-point of the germ plasm in the z-axis) were made with the ‘sum slices’ function. A ROI of approximately 1600 µm3 within the germ plasm and a ROI of approximately 83100 µm3 within bulk cytoplasm was selected for each embryo. A large ROI was chosen for the bulk cytoplasm to miminize variation in signal due to interference from yolk. The integrated density of the ROI region was divided by the corresponding volume.
Quantification of particle size and colocalization
Request a detailed protocolConfocal imaging was performed using a Leica SP5 laser scanning microscope with a 63 × 1.4 NA oil immersion objective and GaAsP ‘HyD’ detectors in photon counting mode except for the experiment shown in Figure 3H,I, which was imaged on a Nikon A1 laser scanning microscope with a 60 × 1.4 NA oil immersion objective and GaAsP detectors. An optical zoom of 1.52× (SP5) or 2.9× (A1) was applied to achieve a pixel size of 72 × 72 nm. An approximate 5 µm z-series was taken of the posterior third of the embryo with a step size of 340 nm. The fluorescence intensities of individual particles of oskar, nanos, and Staufen and were quantified as previously described (Niepielko et al., 2018), with unlocalized nanos and oskar particles assumed to contain an average of 1 and 2 transcripts, respectively, as previously determined (Little et al., 2015). With these assumptions, the average intensities of unlocalized particles were used to determine the number of transcripts in germ plasm localized particles (particle size) of the same image. In addition to allowing the determination of the number of transcripts in RNP granules, this method internally normalizes each image allowing direct comparisons. Internally normalized data points for embryos obtained from up to four independent experiments were pooled to obtain sufficient sample sizes; data are reported as the average of the average particle size for each embryo (mean) ± standard error of the mean. Colocalization between oskar and DCP1, Me31B, Pacman, or Staufen and between cyclin B and DCP1 was measured as previously described (Niepielko et al., 2018). Data points for embryos from up to four independent experiments were pooled to obtain sufficient sample sizes.
Live imaging and particle tracking
Request a detailed protocolLive imaging of founder granules and germ granules was performed on a Nikon Ti-E with Yokogawa spinning disc module (CSU-21) with a 60 × 1.4 NA oil immersion objective. Embryos were dechorionated and mounted in halocarbon oil 95 (Halocarbon Products Corporation) under a #1.5 glass coverslip (VWR) on a Lumox dish (Sarstedt), oriented with the dorsal side closest to the coverslip. Movies were acquired at 100 ms exposure in two channels with a frame rate of 5 frames per second. Particles were tracked using the Autoregressive Motion algorithm of Imaris version 9.1.2 (Bitplane).
Immunohistochemistry
Request a detailed protocolFixed embryos were rehydrated stepwise into PBST, then blocked by incubation in BBT (PBST supplemented with 0.1% globulin free BSA [Sigma-Aldrich]), 5 × 25 min at room temperature with rocking. The embryos were then incubated with 1:2000 rabbit anti Vasa antibody (Liu et al., 2003) diluted in BBT overnight at 4°C with rocking, followed by 10, 20 and 30 min washes with BBT at room temperature with rocking. The embryos were blocked again for 30 min with BBT + 2% normal goat serum (NGS), then incubated with 1:2000 biotin goat anti rabbit antibody (Jackson Immuno Research Laboratories Inc) in BBT + 2% NGS for 2 hr at room temperature with rocking. The embryos were then washed for 10, 20, and 2 × 30 min in PBSTx (PBS, 0.1% TritonX100 [Sigma-Aldrich]) at room temperature with rocking. Secondary antibody amplification was performed with Vectastain reagent according to the manufacturer's instructions (Vectastain Elite PK6100 ABC kit; Vector Labs) and detected using peroxidase immunohistochemistry. Embryos were mounted in 80% glycerol and imaged using Nomarski optics.
Immunoblotting
Request a detailed protocolOvaries were dissected from female fed with yeast paste for 2 days at 25°C, then frozen in liquid N2. Frozen ovaries were homogenized in boiling 2 × sample buffer (0.125 M Tris-HCl pH 6.8, 4% SDS, 20% glycerol, 5 M urea, 0.1 M DTT, 0.01% bromophenol blue) and boiled for 5 min. Samples were spun for 15 min at 4°C and the supernatant was run on a 10% SDS-PAGE gel. The membrane was blocked 3 × 10 min in Blotto (10 mM Tris-HCl pH 7.5, 150 mM NaCl, 0.1% Tween-20, 5% nonfat dry milk). The membrane was then cut just below the 100 kDa marker; the top portion was incubated at 4°C overnight in Blotto with 1:10,000 rabbit anti-Kinesin heavy chain (Cytoskeleton Inc) and the bottom portion was incubated similarly with 1:2000 rabbit anti-Oskar (gift of A. Ephrussi). After washing 3 × 15 min in Blotto at room temperature, the membranes were incubated in 1:2000 HRP donkey anti rabbit antibody for 1.5 hr at room temperature. The membrane was washed 3 × 15 min in TBST (10 mM Tris-HCl pH 7.5, 150 mM NaCl, 0.1% Tween-20). Antibodies were detected using the Lumi-Light Plus Western Blotting Substrate (Roche) and the blot was imaged using an iBright FL1000 Imaging System (Invitrogen).
RT-qPCR
Request a detailed protocolRNA was extracted from dechorionated embryos using the RNeasy kit (Qiagen). 1 µg total RNA was used to generate cDNA using the Quantitect RT kit (Qiagen). 2 µl cDNA was combined with 25 µl 2 × TaqMan Gene Expression Master Mix (Thermo Fisher Scientific), 2.5 µl of 20 × TaqMan Gene Expression Assay (Thermo Fisher Scientific, oskar custom – Dm02134535, 4351372 or RpL7 Dm01817653, 4351372), and 20.5 µl of nuclease free H2O. qPCR was performed on an Applied Biosystems 7900HT standard 96-well qPCR instrument. Three biological replicates were performed with three technical replicates each, all using a CT threshold of 0.6613619. Technical replicates were averaged and copy number determined using previously established standard curves (Eagle et al., 2018). Copy number of the three biological replicates was normalized to the RpL7 control and shown as mean ± s.d.
Data availability
Source data files have been provided for all figures and figure supplements.
References
-
Aubergine is a component of a Nanos mRNA localization complexDevelopmental Biology 349:46–52.https://doi.org/10.1016/j.ydbio.2010.10.002
-
Role for mRNA localization in translational activation but not spatial restriction of nanos RNADevelopment 126:659–669.
-
The autosomal FLP-DFS technique for generating germline mosaics in Drosophila melanogasterGenetics 144:1673–1679.
-
RNA tales – how embryos read and discard messages from momJournal of Cell Science 131:jcs201996.https://doi.org/10.1242/jcs.201996
-
The Drosophila melanogaster gene brain tumor negatively regulates cell growth and ribosomal RNA synthesisDevelopment 129:399–407.
-
Stress-induced mRNP granules: form and function of processing bodies and stress granulesWiley Interdisciplinary Reviews: RNA 10:e1524.https://doi.org/10.1002/wrna.1524
-
Aubergine encodes a Drosophila polar granule component required for pole cell formation and related to eIF2CDevelopment 128:2823–2832.
-
Regulation and function of maternal gene products during the Maternal-to-Zygotic transition in DrosophilaCurrent Topics in Developmental Biology 113:43–84.https://doi.org/10.1016/bs.ctdb.2015.06.007
-
Germ plasm biogenesis--an Oskar-Centric perspectiveCurrent Topics in Developmental Biology 116:679–707.https://doi.org/10.1016/bs.ctdb.2015.11.024
-
The maternal gene Nanos has a central role in posterior pattern formation of the Drosophila embryoDevelopment 112:679–691.
-
Localization of vasa protein to the Drosophila pole plasm is independent of its RNA-binding and helicase activitiesDevelopment 120:1201–1211.
-
Independent and coordinate trafficking of single Drosophila germ plasm mRNAsNature Cell Biology 17:558–568.https://doi.org/10.1038/ncb3143
-
Assembly of the Drosophila germ plasmInternational Review of Cytology 203:187–213.https://doi.org/10.1016/s0074-7696(01)03007-8
-
Miranda mediates asymmetric protein and RNA localization in the developing nervous systemGenes & Development 12:1847–1857.https://doi.org/10.1101/gad.12.12.1847
-
Drosophila germ granules are structured and contain homotypic mRNA clustersNature Communications 6:7962.https://doi.org/10.1038/ncomms8962
-
Oskar anchoring restricts pole plasm formation to the posterior of the Drosophila oocyteDevelopment 129:3705–3714.
-
RNA controls PolyQ protein phase transitionsMolecular Cell 60:220–230.https://doi.org/10.1016/j.molcel.2015.09.017
Article and author information
Author details
Funding
National Institutes of Health (R01 GM067758)
- Elizabeth R Gavis
National Institutes of Health (R35 GM126967)
- Elizabeth R Gavis
National Institutes of Health (T32 GM007388)
- Elizabeth R Gavis
The funders had no role in study design, data collection and interpretation, or the decision to submit the work for publication.
Acknowledgements
We are grateful to P Lasko, S Newbury, H Siomi, and D St Johnston for antibodies, P Macdonald for plasmid DNA, and P Macdonald, W Theurkauf, and E Wieschaus for fly stocks. We thank G Laevsky for assistance with confocal imaging. S Chatterjee for technical assistance, and W Eagle, F Hughson, and M Niepielko for critical comments on the manuscript. This work was supported by National Institute of Health (NIH) grants R01 GM067758 and R35 GM126967 to ERG. CEE was supported by NIH training grant T32 GM007388.
Copyright
© 2020, Eichler et al.
This article is distributed under the terms of the Creative Commons Attribution License, which permits unrestricted use and redistribution provided that the original author and source are credited.
Metrics
-
- 2,095
- views
-
- 334
- downloads
-
- 41
- citations
Views, downloads and citations are aggregated across all versions of this paper published by eLife.
Citations by DOI
-
- 41
- citations for umbrella DOI https://doi.org/10.7554/eLife.49988