Robust perisomatic GABAergic self-innervation inhibits basket cells in the human and mouse supragranular neocortex
Figures
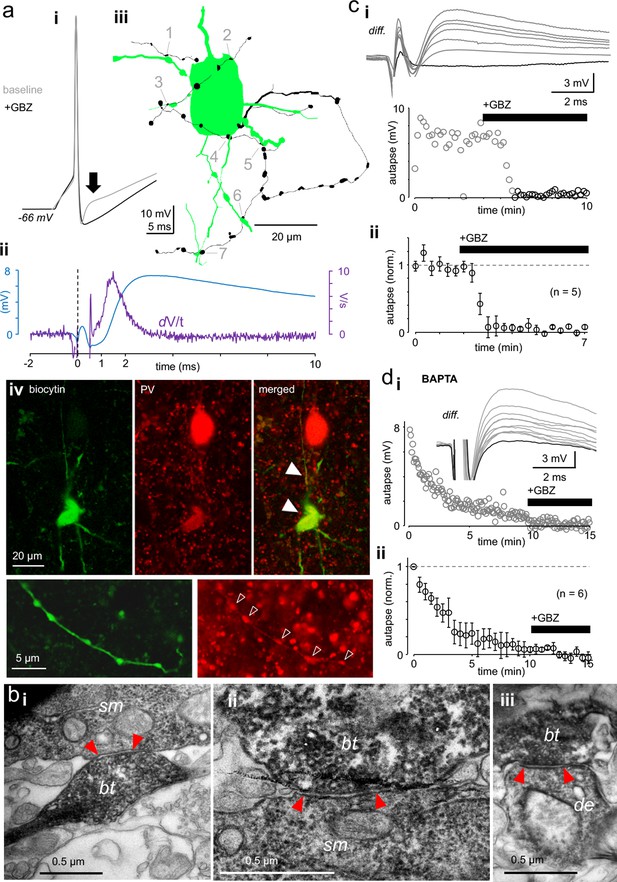
Autaptic GABAAR-mediated innervation in human supragranular pvBCs.
(a) Elevated intracellular chloride reveals GABAAR-mediated self-innervation in layer 2/3 parvalbumin-immunopositive basket cells (pvBCs). (i) Gray trace shows unitary spike-evoked depolarizing autaptic potential (peak indicated by arrow) measured in current clamp. Black trace shows its blockage by gabazine (GBZ, 10 μM). Traces are averages of six. (ii) Autaptic potential (blue trace) shown as a differential of the response under control conditions and in the presence of GBZ. The first derivate (dV/t) of this potential reveals <1 ms onset delay to the action potential peak (at 0 time point). (iii) Light microscopic reconstruction of the same cell reveals a self-innervating axon (black), forming boutons (1-7) in close apposition to its own soma and proximal dendrites. (iv) Confocal fluorescence images of the same cell (biocytin-Alexa488) show pv (Cy3) immunopositive soma, dendrites (solid arrowheads) and axon (open arrowheads). (b) Innervation pattern of autaptic boutons. Electron microscopic images illustrate a sample biocytin-filled pvBC axon forming autaptic appositions to the soma and proximal dendrite. (i-ii) Sample autaptic boutons (bt) terminating on soma (sm). (iii) A bouton forming autaptic contact in the same cell to proximal dendrite (de). Red arrows indicate the active zone. (c) Autaptic response is systematically blocked by gabazine (GBZ, 10 μM). (i) Top: Unitary spike-evoked autaptic potentials shown as differential as in aii (diff.). Traces are during baseline and show the effect of GBZ wash-in (interval 10 s). Black trace shows full blockade. Bottom: Plot shows the GBZ effect on the autaptic potential peak amplitude in the same experiment. (ii) Mean ± sem of 5 similar experiments (bin 30 s, amplitude baseline-normalized). (d) Intracellular BAPTA (10 mM) abolishes autaptic potential gradually. (i) Slow inhibition of a spike-evoked autaptic potential peak amplitude in one experiment (interval 5 s). GBZ (10 μM) was applied at the end. Inset: Traces show vanishing autaptic potential (diff.) by course of experiment with BAPTA-containing filling solution. The lowest trace (full blockade) is in the presence of GBZ. (ii) Mean ± sem of 6 similar experiments (30 s bin, amplitude normalized by the average of the first 30 s).
-
Figure 1—source data 1
Source data for Figure 1C, D.
- https://cdn.elifesciences.org/articles/51691/elife-51691-fig1-data1-v2.xlsx

Identification of human L2/3 fast-spiking basket cells.
(a) Basket cells were identified by their axon forming characteristic basket-like terminals onto the somata of nearby neurons. Light microscopic reconstruction of a fast-spiking interneuron in 50 μm-thick section (red, soma and dendrites; green, axon). Insets: Confocal fluorescence microscope images (Cy3, from another section of the same cell) show axon boutons arranged around unlabeled L2/3 cell somata (somata indicated by asterisks). Scale 10 μm. Axon boutons were confirmed immunopositive for parvalbumin in 28 of 39 successfully visualised basket cells (in 11 basket cells pv immunoreaction was unsuccessful or non-conclusive) (Supplementary file 1). (b) Basket cells show rapid action potential inward current kinetics compared to most other interneurons. (i) Action potential escape current trace (induced by a depolarizing step from Em in a voltage clamp) showing the inward current component in a parvalbumin immunopositive basket cell (pvBC). The inward current is slower in a nonFSIN interneuron immunonegative for parvalbumin (nonFSIN). Arrows indicate the action inward current width (black arrow, onset. Red and blue arrows, inward current recovers to the onset level). (ii) Histograms showing action inward current width in all basket cells (red, n = 64 including the supplementary data cells) and in nonFSINs (blue, n = 22) (bin 50 μs). Red and blue bars indicate anatomically identified basket cells and nonFSINs, respectively. Black bars indicate unsuccessfully visualized putative basket cells and a nonFSIN. (iii) Cumulative histogram of same data (D = 0.922, p=0.0001, two-sample Kolmogorov-Smirnov test). (c) Fast-spiking axo-axonic cells (AACs) were identified and excluded. Systematic visualization of recorded neurons allowed anatomical identification of occasional AACs and their exclusion from fast-spiking cell data. (i) AAC action potential inward current is fast (width from black to green arrow). (ii) Light microscopic reconstruction of one AAC in a 50 μm-thick section (green, soma and dendrites; black, axon). Note vertical axon terminal structures (some indicated by red arrows) characteristic of AAC. (iii) Confocal fluorescence images show biocytin-filled axon terminals (Cy3) of one recorded AAC forming bouton cartridges in close apposition to L2/3 pyramidal cell initial segments. Initial segments were visualized by anti-ankyrinG immunoreaction (Alexa 488) in 3 AACs (see Methods). In line with a previous animal study (Tamás et al., 1997), the human AACs also failed to show evidence (functional, n = 3; anatomical as close appositions, n = 5) for autapses (n = 5 AACs). It suggests that the seven unsuccessfully visualized fast-spiking interneurons with autaptic GABAergic transmission are likely to be basket cells (see Supplementary file 1).
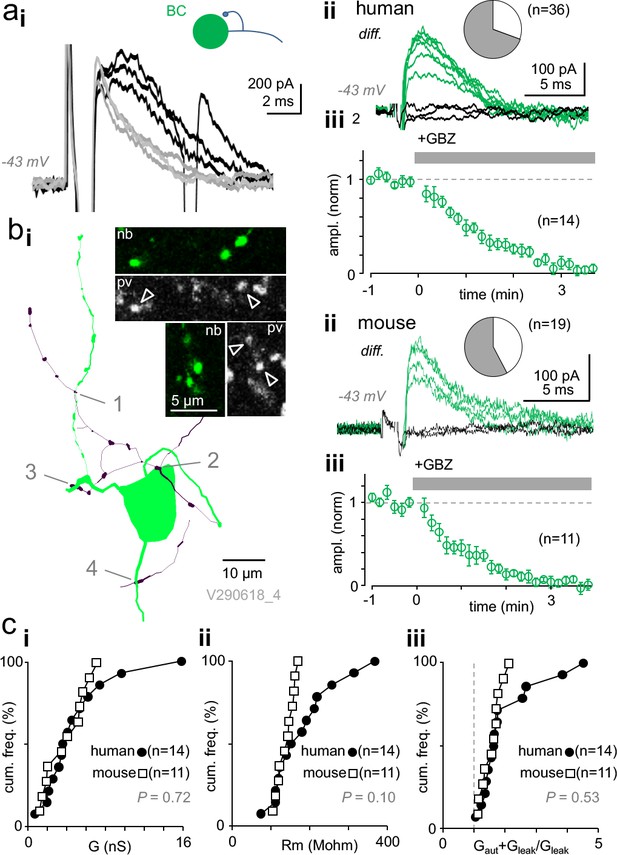
Autaptic self-inhibition strength in human and mouse pvBCs evoked by unitary spikes.
(a) A voltage clamp recording in human pvBCs shows autaptic GABAAR-mediated inhibitory current (intracellular chloride 8 mM). (i) Unitary spike-evoked responses in a human pvBC during baseline (black) and after wash-in of gabazine (GBZ, 5 min, gray) (interval 10 s, at −43 mV). Note the second action potential escape current generated occasionally. (ii) Subtraction of the traces reveals GABAergic current. Traces show the autaptic GBZ-sensitive current (diff.) in baseline (green) and when fully blocked (black, subtraction of traces in GBZ). Inset pie chart shows the proportion of cells with GBZ-sensitive autapse (green) in all pvBCs studied in voltage- or current clamp (in 25 of 36). (iii) Mean ± sem of GBZ wash-in in all pvBCs (n = 14, amplitude baseline-normalized, 30 s bin). (b) GABAAR-mediated self-innervation and autaptic current in mouse pvBCs. (i) A visualized mouse pvBC perisomatic area showing axon (black) boutons forming close appositions (1-4) to its own soma and proximal dendrites. Confocal microscopic micrographs illustrate the pv immunopositive (pv, Cy5) axon of the cell (nb, Alexa488). (ii) GBZ-sensitive autaptic current (diff.) under control conditions (green). Black traces are the subtraction of traces in GBZ. Inset pie chart shows the proportion of mouse pvBCs with autapses (in 11 of 19 cells). (iii) Mean ± sem of 11 experiments showing GABAergic current blockade by wash-in of GBZ (amplitude baseline-normalized). (c) Autaptic self-inhibition efficacy in human and mouse pvBCs. Cumulative presentation of solid and open symbols indicates the average in cells. (i) Self-inhibition peak conductance (G) in human and mouse pvBCs. (ii) Input resistance (Rm) in humans and mice. (iii) Calculated total input conductance of a cell during the autaptic current peak (Gaut + Gleak), divided by its resting conductance (Gleak). The ratio shows how much self-inhibition increases membrane leakage.
-
Figure 2—source data 1
Source data for Figure 2A, B, C.
- https://cdn.elifesciences.org/articles/51691/elife-51691-fig2-data1-v2.xlsx

GABAergic self-innervation is comparable to synaptic connection from pvBC to L2/3 neurons.
(a) A paired recording of a synaptically connected pvBC (green)-to-PC (red) pair. (i) Close apposition pvBC axon boutons (black) showing some autaptic (1-2) and synaptic (1-6) contacts. (ii) Left: plot shows autaptic (green symbols) and synaptic (red symbols) currents measured simultaneously in a paired recording. After a baseline, wash-in of gabazine (GBZ, 10 μM) blocks both currents. Right: superimposed individual traces of the autaptic and synaptic currents under control conditions. (b) Average autaptic GABAAR-mediated peak conductance is comparable to synaptic conductance from pvBC to PC. Autapses and synapses were recorded in separate experiments (GBZ not washed in 15 of 20 synaptic pair recordings). (i) On average, pvBC self-innervation (green, 14 pvBCs) shows similar peak conductance as monosynaptic IPSCs in L2/3 PCs (red, 20 pvBC-PC synaptic pairs). The autapse data are the same as those presented in Figure 2. (ii) Input resistance (Rm in resting condition) is higher in pvBCs (green) than in PCs (red) (p=0.001, Mann–Whitney U -test). This result suggests a theoretically stronger average shunting inhibition effect by autapses in pvBCs than by synapses in PCs. (c) Triple recording from a pvBC with autapse and synaptic connection to a PC and to a FSIN. Note the similar rise kinetics of the autaptic current and IPSC in FSIN.
-
Figure 2—figure supplement 1—source data 1
Source data for Figure 2—figure supplement 1B.
- https://cdn.elifesciences.org/articles/51691/elife-51691-fig2-figsupp1-data1-v2.xlsx

Action potential afterhyperpolarization peak conductance (Gahp) in human pvBCs shows large variation.
It correlates with pvBC membrane leak conductance (Pearson's r = 0.671, p=0.009, n = 14).
-
Figure 2—figure supplement 2—source data 1
Source data for Figure 2—figure supplement 2.
- https://cdn.elifesciences.org/articles/51691/elife-51691-fig2-figsupp2-data1-v2.xlsx
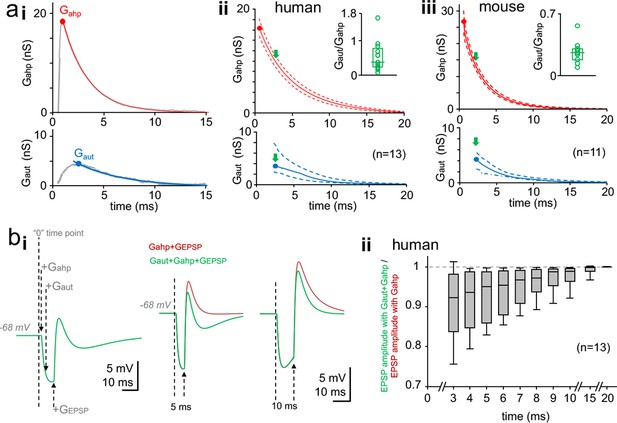
Time window for autaptic self-inhibition in pvBCs following a spike.
(a) Autaptic GABAAR-mediated conductance (Gaut) overlaps with AHP conductance (Gahp). (i) The two conductances Gahp (top) and Gaut (bottom) in a human pvBC (gray lines, both averages of six). Colored (top, red; bottom, blue) curves demonstrate monoexponential decay from the peak (solid circle). Abscissa ‘0’ shows the timing of spike inward current peak. (ii) Monoexponential decay curves for Gahp and Gaut from peak amplitude (solid circle) in human pvBCs. Red solid line shows mean and dotted lines ± sem (parametric amplitude and decay tau distribution, Shapiro-Wilk normality test). Blue solid line shows median and the dotted lines indicate upper and lower quartiles (non-parametric data distribution) (n = 13, a pvBC with smallest Gaut excluded). Abscissa indicates delay to a spike inward current peak. Inset: high variance in Gaut/Gahp between cells (circles, average in each cell; box plot, median and quartiles of the averages). The Gaut/Gahp plotted at Gaut peak time point (green arrows in the main plot). (iii) Monoexponential decay curves for Gahp and Gaut in mouse pvBCs (n = 11). Inset shows Gaut/Gahp variation between individual cells. (b) Basket cell inhibition by Gaut following a spike. Simulation study with a single-cell perisomatic model uses parameters recorded in the human pvBCs (see Supplementary file 2). (i) Membrane potential traces from a pvBC simulation demonstrate experimental design. Left: Vertical dotted lines show the schematic timing of conductances applied. ‘0’ means the time point where action potential inward current peak would occur. +Gahp and +Gaut show application of these conductances with cell-specific amplitude, delay and decay time. +GEPSP shows initiation of EPSP (using kinetics and amplitude common in human pvBCs). Middle: Superimposed traces show EPSP (generated with 5 ms delay to spike) in two conditions. Green trace shows the EPSP in the presence of Gaut and Gahp. Brown trace shows increased EPSP amplitude without Gaut. Right: GEPSP applied 10 ms after spike (‘0’ time point) in the two conditions. Turning off Gaut still increases EPSP amplitude. Resting membrane potential (Em) = −68 mV. AHP reversal potential = −90 mV. EGABA-A = 10.84 ± 0.53 mV negative to Em. (ii) Summary of the simulation in 13 human pvBCs. EPSP was evoked in the two conditions with increasing delay to spike. Box plot shows ‘the green trace EPSP’ amplitude divided by ‘the brown trace EPSP’ amplitude at time points with increasing delay to spike (median, upper and lower quartiles with 5 and 95 percentiles).
-
Figure 3—source data 1
Source data for Figure 3A, B.
- https://cdn.elifesciences.org/articles/51691/elife-51691-fig3-data1-v2.xlsx
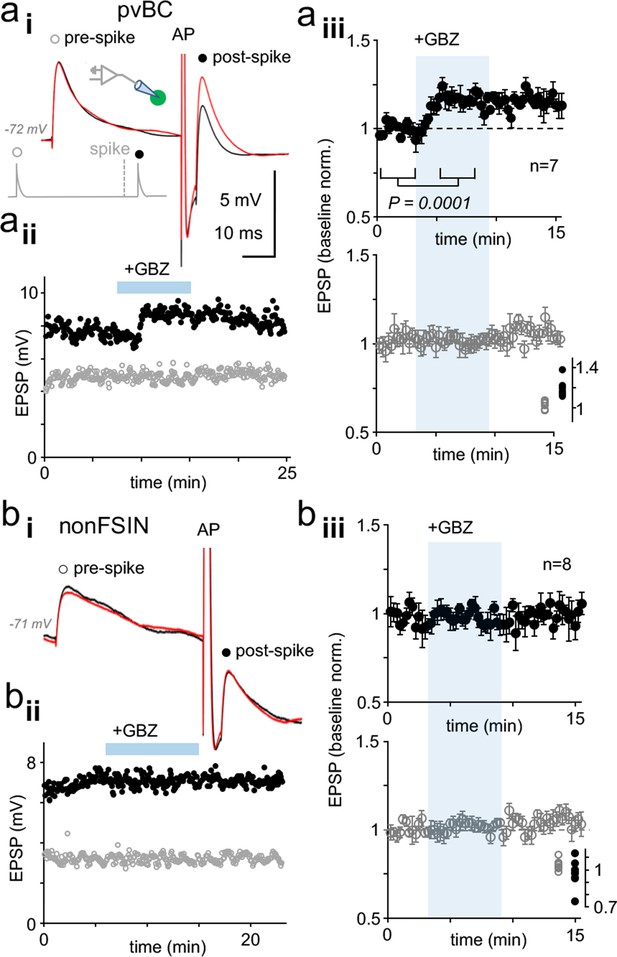
Dynamic clamp reveals autaptic inhibition of EPSPs in human pvBCs but not in nonFSINs.
(a) GABAAR-mediated self-inhibition of EPSP in pvBCs. A dynamic clamp experiment with two subthreshold EPSPs generated 40 ms before (prespike) and 5 ms after (postspike) a spike in pvBCs. Spike was evoked by a brief (0.5 ms) suprathreshold step. (i) Sample EPSPs (average of 6) in baseline conditions (black) and after wash-in of GBZ (10 μM) (red). Note the selective increase of the post-EPSP amplitude by GBZ. Inset schematic shows experimental design with prespike (open symbol), postspike (solid symbol) and suprathreshold (middle) conductance commands. The initiation of postspike EPSC conductance was time-locked to the peak of action potential evoked. (ii) Amplitude of the pre- (open symbols) and postspike EPSPs (solid symbols) in the same experiment (amplitude from onset to peak). GBZ wash-in is indicated by a horizontal bar. (iii) Mean ± sem of 7 pvBCs (baseline-normalized). The shaded area indicates the wash-in of GBZ. Inset summarizes the baseline-normalized pre- and postspike amplitudes in the presence of GBZ in individual experiments. (b) NonFSINs fail to show GABAAR-mediated self-inhibition of somatic EPSP. (i) Traces illustrate pre- and postspike EPSP (average of 6) in a nonFSIN during baseline (black) and in GBZ (10 μM) (red). (ii) Amplitude of the pre- and postspike EPSPs plotted in the same experiment. (iii) Mean ± sem of 8 nonFSINs. Inset summarizes postspike EPSP amplitudes in GBZ (baseline-normalized).
-
Figure 4—source data 1
Source data for Figure 4A, B.
- https://cdn.elifesciences.org/articles/51691/elife-51691-fig4-data1-v2.xlsx
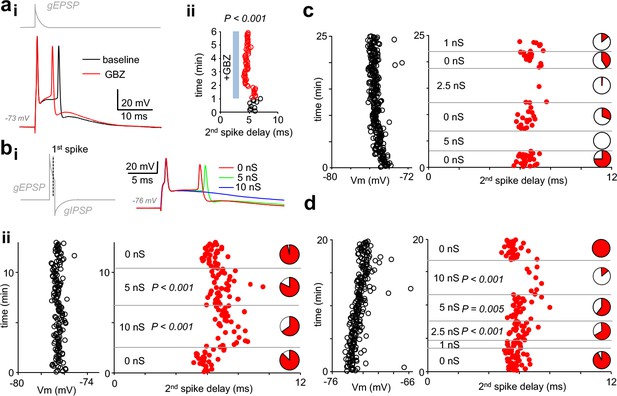
Autaptic inhibitory conductance regulates firing in human pvBCs.
(a) GBZ shortens a time delay between two spikes evoked by EPSP in dynamic clamp. (i) Sample traces in a pvBC evoked by EPSP in dynamic clamp under control conditions during baseline and after wash-in of GBZ (10 μM). Trace on top (gray) shows EPSP conductance command (gEPSP). (ii) Delay from the 1st to the 2nd spike plotted in the same experiment under control conditions (black line circles) and during wash-in of GBZ (red line circles). Cycle interval 5 s. (b) Inhibition of doublet spiking by natural autaptic conductance demonstrated with dynamic clamp in human pvBCs. Experiments in the presence of GBZ show decreased probability and increased delay of the second spike when autaptic conductance is introduced with dynamic clamp. Spikes are evoked by large single EPSP as in (a). (i) Left: dynamic clamp command schematic with EPSP conductance followed by GABAAR autaptic IPSC conductance (with 1 ms onset delay to 1st spike generated by EPSP). Right: traces showing the evoked spikes. (ii) Left: pvBC membrane potential during an experiment. Middle: red dots indicate the second spike onset delay to the first spike elicited by dynamic clamp EPSP (interval 5 s) in the absence of autapse, and when it was followed by autaptic IPSC conductance (5 nS or 10 nS). P-values show spike delay increased by autaptic conductance compared to baseline (ANOVA on ranks, post hoc Dunn’s pairwise test against all 0 nS data as control). Right: pie charts show the 2nd spike probability (red area) in all cycles with the specific IPSC conductance. Note the increased failure rate (white pie chart area) with 5 nS or 10 nS inhibitory autapse. (c-d) Similar experiments as in (b) in two additional pvBCs in which autaptic inhibition (1 nS −10 nS) was more clearly seen in the probability of the 2nd spike (white areas in pie charts indicate spike failures). P-values show increased spike delay compared to baseline (ANOVA on ranks with post hoc Dunn’s pairwise test against all 0 nS data as control).
-
Figure 5—source data 1
Source data for Figure 5B, C, D.
- https://cdn.elifesciences.org/articles/51691/elife-51691-fig5-data1-v2.xlsx
Additional files
-
Supplementary file 1
Details of recorded human neurons and information on the resected human cortical tissue.
Columns from left to right show the figure in which the cell recording data are shown (cells showing no evidence for autapse are indicated as ‘no aut’), cell filing code, indication for successful recovery of cell with streptavidin visualization (strept) (NA indicates unsuccessful cell recovery), immunoreaction (positive as + and negative as -) for parvalbumin (pv) and vesicular GABA transported (vGAT), action potential inward current width (acw), firing frequency accommodation shown in cells where it was tested (first number shows firing frequency as ‘Hz’ during first 100 ms of a robust although not always maximal depolarizing pulse. Second number shows firing frequency accommodation ‘acc.’ = firing frequency at 400–500 ms during the depolarization divided by firing frequency during first 100 ms), and resting membrane potential (Em). Details of resected tissue are shown in blue, showing patient gender, age, hemisphere, cortical area and diagnosed primary pathology. There is ‘no info’ about exact neocortical area in some ventriculostomy operations.
- https://cdn.elifesciences.org/articles/51691/elife-51691-supp1-v2.docx
-
Supplementary file 2
Recorded parameters used in computational simulation of human pvBCs.
- https://cdn.elifesciences.org/articles/51691/elife-51691-supp2-v2.xlsx
-
Transparent reporting form
- https://cdn.elifesciences.org/articles/51691/elife-51691-transrepform-v2.docx