Chemoptogenetic ablation of neuronal mitochondria in vivo with spatiotemporal precision and controllable severity
Figures
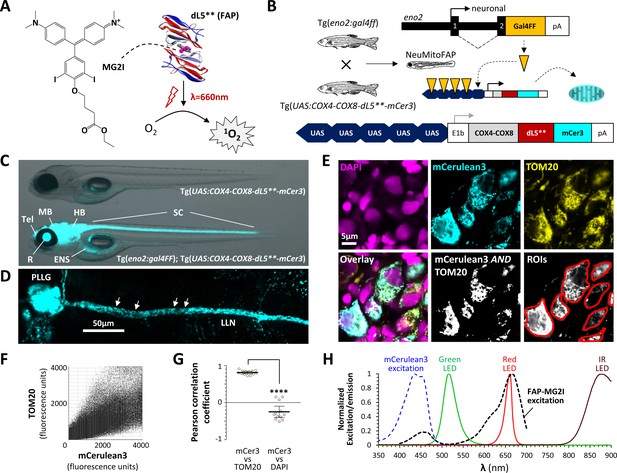
Generation of NeuMitoFAP zebrafish.
(A) When the fluorogen MG2I (chemical structure shown on left) is bound to the fluorogen-activating protein (FAP) dL5** (right), excitation by far-red light causes generation of singlet oxygen. (B) Diagrams of transgene constructs eno2:gal4FF (above) and UAS:COX4-COX8-dL5**-mCer3 (below). Transactivation of the UAS enhancer by Gal4 in the neurons of double transgenic Tg(eno2:gal4ff); Tg(UAS:COX4-COX8-dL5**-mCer3) ‘NeuMitoFAP’ zebrafish results in expression of the dL5**-mCerulean3 fusion protein in the mitochondrial matrix. (C) Merged phase contrast and mCerulean3 epifluorescence images, showing live Tg(UAS:COX4-COX8-dL5**-mCer3) (above) and Tg(eno2:gal4ff); Tg(UAS:COX4-COX8-dL5**-mCer3) (NeuMitoFAP; below) zebrafish larvae at 5 days post-fertilization. mCerulean3-expressing structures are labeled (Tel, telencephalon; MB, midbrain; HB, hindbrain; SC, spinal cord; R, retina, ENS enteric nervous system). (D) Confocal z-plane projection showing mCerulean3 expression in the posterior lateral line ganglion (PLLG) and lateral line nerve (LLN) of a NeuMitoFAP zebrafish. Individual axonal mitochondria are indicated (arrows). (E) Brain sections from NeuMitoFAP zebrafish were labeled for nuclei (DAPI; magenta), dL5**-mCerulean3 (cyan) and mitochondria (TOM20; yellow). Single confocal planes of the individual channels are shown in the upper row. The lower row shows: the three channels overlaid; the output of a Boolean (mCerulean3 AND TOM20) map; and representative regions of interest that were analyzed in panels F and G. (F) Scatter plot of TOM20 signal (y-axis) versus mCerulean3 signal (x-axis) in each pixel within regions of interest. (G) Pearson correlation coefficient of signal intensity for mCerulean3 versus TOM20 (left) compared with mCerulean3 versus DAPI (right). Each data point shows a region of interest corresponding to an individual mCerulean3-expressing cell, bars show mean ± SE; ****p<0.0001, 2-tailed t-test. (H) Normalized excitation and emission spectra of the fluorophores and light sources used in this study.
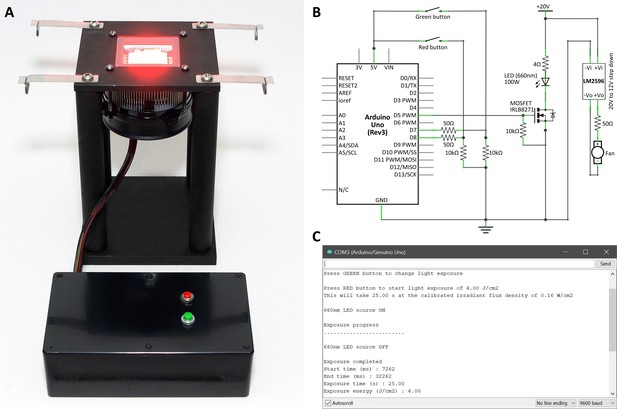
High-power LED light source to deliver far-red light to larval zebrafish.
(A) A LED light source (100W, 660 nm; Chanzon, Amazon.com) was mounted on a CPU heatsink and fan for cooling. The LED/fan assembly was suspended beneath an optical glass window on a custom stand, so that samples placed on the glass could be exposed to high-intensity light without significant heating through conduction or convection from the LED array. Clips on the platform support a shade that covers specimens during light exposure for operator comfort. (B) The LED was controlled using an Arduino Uno ATmega328P microcontroller board. The digital output signal from the board switches the gate voltage of a MOSFET that controls the LED power circuit. This allows: (i) precise automated exposure timing, to control the amount of light energy delivered; and (ii) LED dimming, using pulse width modulation, to control the power at which light energy is delivered. The control box also contains a voltage step-down to allow the LED (20V) and the fan (12V) to be operated from the same 20V power source, and two buttons providing digital logic inputs to the board that are used to trigger functions in the software. (C) The microcontroller board was programmed using the Arduino integrated development environment. The control box was connected to a PC via a USB connection and the Arduino serial monitor was employed as an interactive interface for light calibration, and control of exposure time and power.
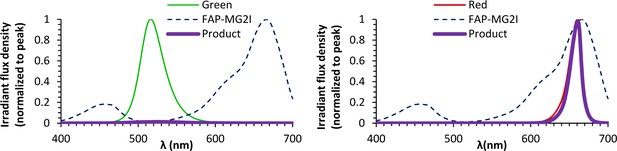
Energy transfer spectra between LED sources and dL5**-MG2I.
The dL5**-MG2I excitation spectrum (Figure 1H) was multiplied by emission spectra from the green and red LEDs (Figure 1H) to generate energy transfer spectra. Quantitative properties of the energy transfer spectra are shown in Table 2. In the context of the low power emitted from the green lights used for handling the NeuMitoFAP zebrafish and eliciting neurobehavioral responses, the small amount of overlap between the green light emission spectrum and the dL5**-MG2I excitation spectrum caused negligible energy transfer during the time course of the experiments.
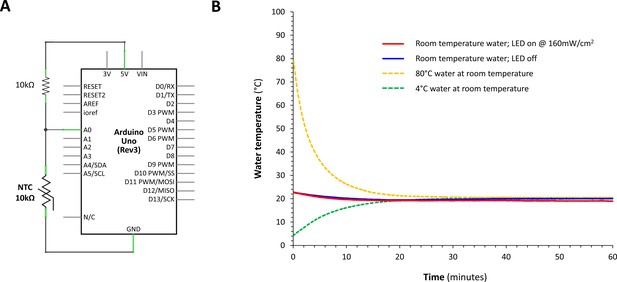
LED light source did not cause appreciable heating of water bath.
(A) A waterproof resin-coated negative temperature coefficient thermistor was used to provide continuous monitoring of bath temperature. The resistance of the thermistor was determined in a voltage divider circuit as shown. The potential difference across the thermistor was measured through the analog input to an ATmega328P microcontroller board. The temperature was determined by first calculating the thermistor resistance as , where Ain is the 10-bit digital value converted from the input voltage at the analog input pin. The temperature was then calculated as , where R0 = 10kΩ at T0 = 25°C, and B = 3950, the coefficient of the thermistor. Since the thermistor was coated in black resin, it was wrapped in reflective aluminum foil to prevent direct warming by radiation from the LED source, thereby allowing specific and sensitive measurement of bath water temperature during light exposure. The thermistor circuit in this configuration provided accurate water temperature measurements within ± 0.5°C compared with a digital thermometer. (B) Continuous monitoring of bath temperature over 60 min is shown at a sampling rate of 1 Hz. The room temperature was 20°C and the starting bath temperature was 21°C. Regardless of whether the LED light source was on at full power (160 mW/cm2; solid red line) throughout the measurement period, or off (solid blue line), the bath temperature did not change significantly over 60 min. Controls with no light and starting water temperatures of 80°C (dotted orange line) and 4°C (dotted green line) showed that the foil-wrapped thermistor detected bath temperature changes rapidly and accurately. Together these data show that heat transfer from the LED light source was not sufficient to cause water temperature changes during zebrafish light exposure.
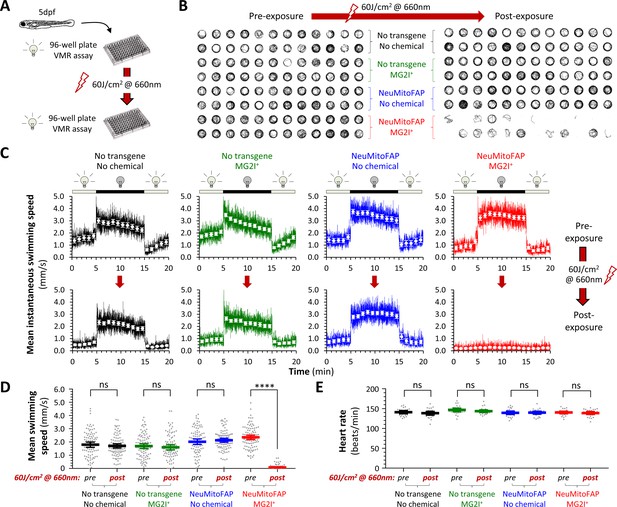
Acute loss of neurological function in NeuMitoFAP zebrafish exposed to MG2I and far-red light.
(A) Design of experiments shown in panels (B - D). Experimental groups: WT zebrafish (black); WT zebrafish exposed to MG2I (green); NeuMitoFAP zebrafish (blue); NeuMitoFAP zebrafish exposed to MG2I (red). (B) 1 min swimming vectors of zebrafish in a 96-well plate, before (left) and after (right) exposure to 60 J/cm2 far-red light (160 mW, λpeak=661nm). Each group includes 24 zebrafish, occupying two rows of the 96-well plate as indicated. (C) The visual motor response (VMR) was elicited by alternating 10 min periods of green light illumination (250 Lux, λpeak=516 nm; does not activate dL5**-MG2I) and darkness (0 Lux). The graphs show mean instantaneous group swimming speed (y-axis) against time (x-axis). Responses were averaged over three cycles of dark/light stimuli (solid colored lines; illumination cycle shown above each graph); responses of individual zebrafish were further averaged into 1 min time bins (white markers, error bars show ±95% CI). Responses of the same zebrafish are shown before (top row) and after (bottom row) exposure to 60 J/cm2 far-red light. n = 24 zebrafish/group. (D) Mean swimming speed during the dark phase of the VMR (y-axis) was quantified before and after exposure to 60 J/cm2 far-red light. Data points show responses of individual zebrafish (n = 96/group from four combined replicate experiments, bars show mean ± 95% CI). ****p<10−15, pre- versus post-light exposure swimming speed, 2-way ANOVA with Šidák multiple comparisons test. (E) Heart rate (y-axis) was quantified before and after exposure to 60 J/cm2 far-red light (data points show individual zebrafish, bars show mean ± 95% CI).
-
Figure 2—source data 1
Source data for Figure 2C.
- https://cdn.elifesciences.org/articles/51845/elife-51845-fig2-data1-v1.xlsx
-
Figure 2—source data 2
Source data for Figure 2E.
- https://cdn.elifesciences.org/articles/51845/elife-51845-fig2-data2-v1.xlsx
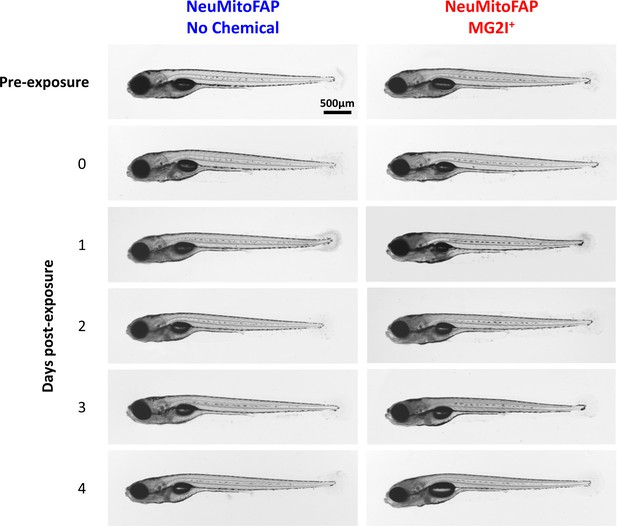
Normal morphology of NeuMitoFAP zebrafish exposed to MG2I and light.
Transmitted light micrographs of NeuMitoFAP zebrafish: tThe right column shows larvae that were exposed to MG2I from 3 dpf; the left column shows untreated zebrafish. The first row shows larvae at 5dpf prior to light exposure. The second row shows larvae at 5dpf immediately after exposure to 60 J/cm2 light at λpeak=661nm. The subsequent rows show larvae at daily intervals (6–9 dpf) following light exposure at 5dpf. Although the MG2I-treated, light-exposed larvae showed loss of motor responses that did not recover during the time course of the experiment (see text), there were no major morphological abnormalities at any time point.
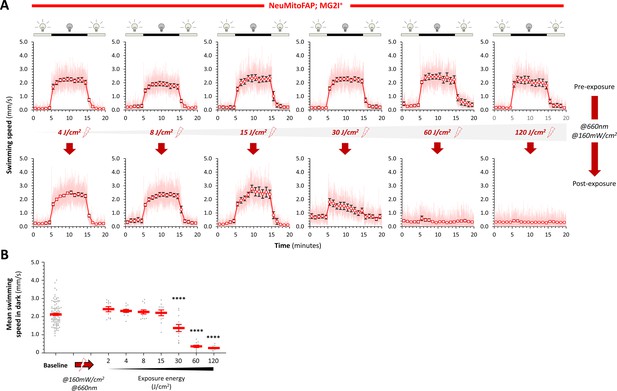
The motor phenotype of NeuMitoFAP-MG2I zebrafish is dependent on far-red light exposure energy.
(A) Visual motor responses were evaluated in Tg(eno2:gal4FF); Tg(UAS:COX4-COX8-dL5-mCer3) (‘NeuMitoFAP’) zebrafish treated with MG2I. Motor response to cycles of green light (200 Lux; 10 min) and abrupt darkness (10 min) were measured in 96-well plates, using an infrared machine vision/automated tracking system (Zhou et al., 2014; Cario et al., 2011). In each graph, the y-axis shows swimming speed (mm/s) and the x-axis time (minutes). The light-colored trace shows the mean instantaneous swimming speed for 12 zebrafish at each frame transition (4 Hz), averaged over 3 cycles of light-dark responses; data for each minute of the response were binned and averaged, allowing calculation of the mean ± SE (round markers and error bars) swimming speed for the 12 zebrafish during each minute of the stimulus cycle. The timing of the light and dark segments of the stimulus cycle are shown above the graphs. Each group of 12 zebrafish was analyzed both before (upper row of graphs) and after (lower row) exposure to far-red light at the incremental total energy doses shown. Each pair of graphs shows analysis of the same animals under basal and post-exposure conditions. (B) Mean swimming speed during the dark phase of the visual motor response was calculated. The scatter plot shows values for individual zebrafish (gray data points) with group mean ± SE superimposed (red bars). Basal dark swimming speed did not differ between the groups, which are combined here for clarity. Swimming speed post-exposure was progressively reduced in the 30, 60 and 120 J/cm2 groups (****p<0.00001, compared with baseline, 1-way ANOVA, with Dunnett’s post hoc test; comparison of pre- and post-exposure data for each group yielded identical results). Together, these data show that the severity of the motility phenotype during the dark phase of the visual motor response in NeuMitoFAP zebrafish treated with MG2I is determined by the amount of light energy delivered.
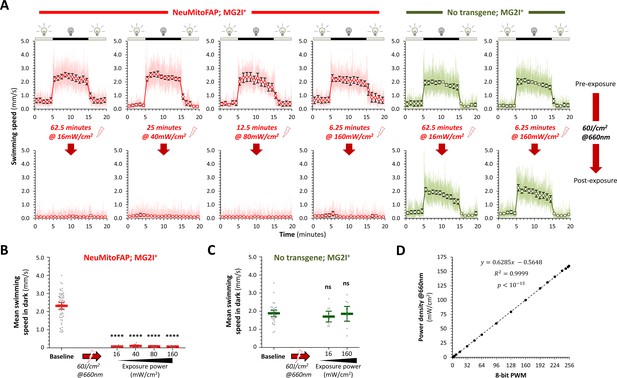
The motor phenotype of NeuMitoFAP-MG2I zebrafish exposed to 60 J/cm2 far-red light is not dependent on light power.
(A) Visual motor responses were evaluated in NeuMitoFAP (red) and non-transgenic (green) zebrafish treated with MG2I, identically to Figure 2—figure supplement 1. Each group of 12 zebrafish was analyzed both before (upper row of graphs) and after (lower row) exposure to red light at the same total energy dose of 60 J/cm2, delivered at incremental power densities between 16 mW/cm2 and 160 mW/cm2 as shown. Each pair of graphs shows analysis of the same animals under basal and post-exposure conditions. (B, C) Mean swimming speed during the dark phase of the visual motor response was calculated. The scatter plot shows values for individual zebrafish (gray data points) with group mean ± 95% CI superimposed (red bars). Basal dark swimming speed was identical in all groups, which have been combined for clarity. (B) Motility was almost completely abolished in NeuMitoFAP zebrafish exposed to MG2I after 60 J/cm2 red light exposure, regardless of exposure power. (C) In contrast, swimming was unaffected at any exposure power in non-transgenic animals exposed to MG2I. (****p<0.00001, compared with baseline, 1-way ANOVA, with Dunnett’s post hoc test; comparing pre- and post-exposure data for each group yielded identical results). (D) The graphs show the linear relationship between the light power output and the full range of the pulse width modulation output from the digital output pin of the controller board, allowing the use of PWM to control power for this experiment. Together, these data show that the severity of the neurological phenotype in NeuMitoFAP zebrafish treated with MG2I and exposed to 60 J/cm2 light is not dependent on the rate at which light energy is delivered.
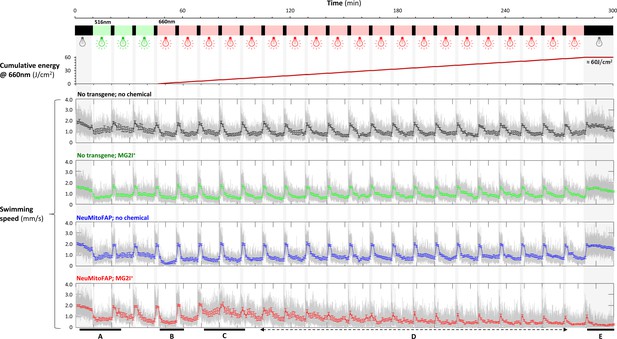
Real-time changes in motor function of NeuMitoFAP-MG2I zebrafish during far-red light exposure.
24 zebrafish from each experimental group (non-transgenic and NeuMitoFAP zebrafish, not exposed to chemical or treated with MG2I; same color key as Figure 2B) were placed in a 96 well plate at 5dpf. Motor function was measured by infrared videography and automated tracking during 5 hr of repeated cycles of 10 min ambient illumination and 2 min darkness (gray bands) to elicit the visual motor response (VMR). The first three cycles used green light illumination @516 nm to elicit the VMR without exciting production of 1O2. The remaining 20 cycles were illuminated with far-red light at λpeak=661nm (≈5 mW/cm2) to both (i) elicit the VMR and (ii) excite production of 1O2 in neuronal mitochondria simultaneously. At the conclusion of the experiment zebrafish had been exposed to a cumulative dose of approximately 60 J/cm2 far-red light (top panel; 5 mW/cm2 × 600 s/cycle = 3 J/cm2/cycle). The movement traces show the instantaneous mean swimming speed for each group of 24 zebrafish (gray); mean ± SE for 1 min time bins are superimposed in color. (A) At the start of the experiment, all four groups showed normal motor function. (B) The first cycle of far-red light also elicited a normal VMR in all four groups. (C) By the third cycle of far-red light, NeuMitoFAP-MG2I zebrafish, but not controls, became hyperkinetic during light and showed an attenuated response to the light-dark transition. (D) During later cycles of far-red light, NeuMitoFAP-MG2I zebrafish, but not controls, became progressively hypokinetic. (E) After a cumulative far-red light dose approximating that shown in Figure 2, NeuMitoFAP-MG2I zebrafish, but not controls, showed severely diminished swimming speed in the dark. These data show that motor function in NeuMitoFAP-MG2I zebrafish worsened progressively with cumulative far-red light exposure, and that a transient phase of hyperkinesia preceded the loss of motility.
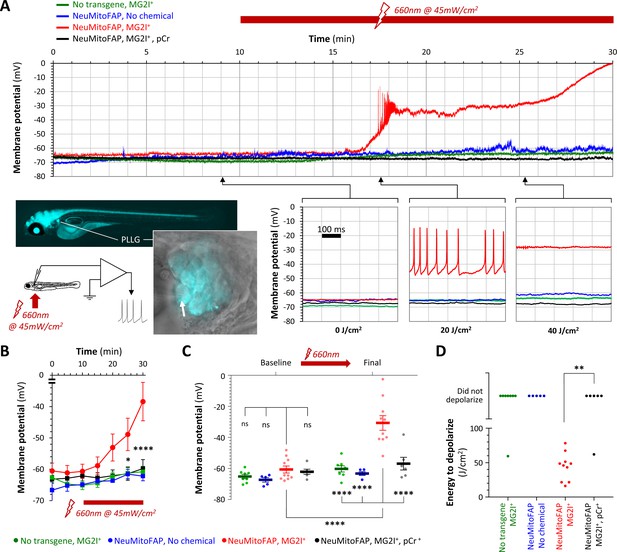
Acute neuronal depolarization in NeuMitoFAP zebrafish exposed to MG2I and far-red light.
(A) Whole-cell patch clamp recordings were made from posterior lateral line ganglion (PLLG) sensory neurons. The inset figure shows the preparation and experimental design (the arrow shows a patch-clamp pipette in contact with a mitoFAP-expressing neuron). Recordings were made for 30–40 min (10 min in darkness and then a further 20–30 min under illumination in far-red light at λpeak=661 nm). The graphs show example traces of membrane potential (y-axis) against time (x-axis), for the full 30 min recording (upper graph) and for three 500 ms sweeps after cumulative far-red light doses of 0, 20 or 40 J/cm2 as indicated. Experimental groups: WT zebrafish exposed to MG2I (green); NeuMitoFAP zebrafish (blue); NeuMitoFAP zebrafish exposed to MG2I (red); NeuMitoFAP zebrafish exposed to MG2I, recordings made with phosphocreatine (pCr) added to pipette solution (black). (B) Mean ± SE membrane potential (y-axis; 5–10 neurons per group) in each 5 min time bin (x-axis) during recording. *p<0.05, ****p<0.0001, NeuMitoFAP-MG2I versus other groups at same time point, 2-way repeated measures ANOVA with Tukey multiple comparisons test. (C) Membrane potential of lateral line ganglion neurons (y-axis) at baseline and final potential after far-red light exposure. Data points show individual neurons, bars show mean ± SE. ****p<0.0001, 1-way ANOVA with Tukey multiple comparison test. (D) Amount of far-red light energy necessary to decrease membrane potential by >20% from baseline value (y-axis). Data points show individual neurons. Neurons that did not depolarize during the recording period are shown above the graph. **p<0.01, Fisher’s exact test.
-
Figure 3—source data 1
Source data for Figure 3B-D.
- https://cdn.elifesciences.org/articles/51845/elife-51845-fig3-data1-v1.xlsx
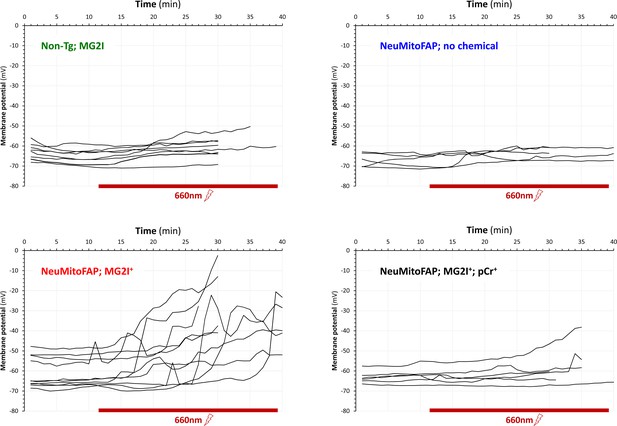
Far-red light-induced depolarization of lateral line ganglion neurons in NeuMitoFAP zebrafish treated with MG2I.
The graphs show membrane potential values (y-axis) of lateral line ganglion sensory neurons over time (x-axis) in whole cell patch clamp recordings from multiple zebrafish in each experimental group. Continuous recordings were analyzed by calculating the mean membrane potential in 60 s time bins. Exposure to far-red light was started 10 min after the establishment of stable recordings. There was no significant baseline membrane depolarization in any experimental group. During exposure to far-red light, multiple neurons from NeuMitoFAP zebrafish treated with MG2I showed dramatic membrane depolarization at varying time points after the onset of light exposure (bottom left). There was no significant membrane depolarization in either non-transgenic zebrafish treated with MG2I (top left) or in NeuMitoFAP zebrafish in the absence of MG2I (top right). The presence of phosphocreatine in the recording pipette prevented depolarization in NeuMitoFAP zebrafish treated with MG2I, showing that depolarization is caused by ATP depletion. Example recordings at high and low temporal resolution are shown in Figure 4A. A summary of these data with statistical analysis is shown in Figure 4B-D.
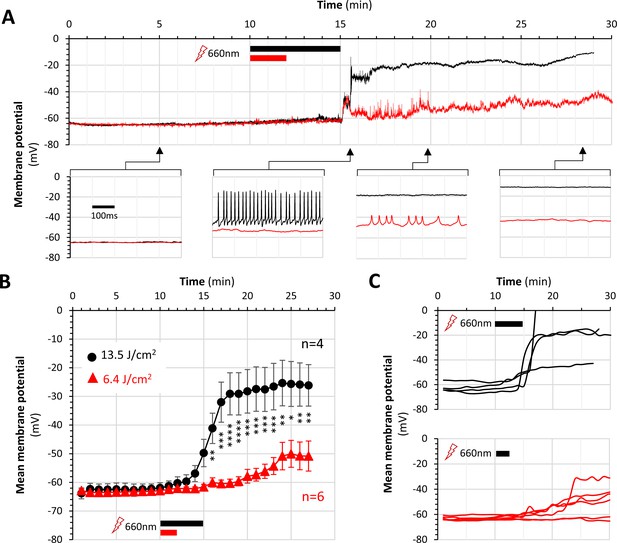
Severity of lateral line ganglion neuron depolarization in NeuMitoFAP-MG2I zebrafish is dependent on far-red light dose.
Whole-cell patch clamp recordings from lateral line ganglion neurons were made exactly as in Figure 4, with the exception that illumination at λpeak=661nm was stopped after 5 min (black; 13.5 J/cm2) or 2 min (red; 6.4 J/cm2) of exposure (the timings of far-red light exposures are shown as black or red bars in each panel). Recordings were made for 30 min (10 min in darkness, 2 or 5 min under illumination in far-red light as indicated, and the remainder in darkness). (A) The graphs show example traces of membrane potential (y-axis) against time (x-axis), for the full 30 min recording (upper graph) and for three 500 ms sweeps at the time points indicated. The neuron exposed to 13.5 J/cm2 far-red light (black) started to depolarize as illumination was discontinued and replicated the stages of depolarization seen during continuous far-red light exposure in Figure 4 – including firing trains of action potentials at high frequency during depolarization before becoming refractory. The neuron exposed to 6.4 J/cm2 far-red light depolarized later and to a more modest extent. (B) Mean ± SE membrane potential is shown for neurons exposed to 13.4 J/cm2 (black circles, n = 4) or 6.4 J/cm2 (red triangles, n = 6) in 1 min time bins before, during and after light exposure. ***p<0.001, **p<0.01, *p<0.05, 13.5 J/cm2 versus 6.4 J/cm2, 2-way repeated measures ANOVA with Šidák multiple comparisons test. (C) Mean membrane potential in each 1-minute time bin for each individual neuron analyzed in panel B. These data show that the severity and rapidity of neuronal depolarization were dependent on the amount of far-red light energy delivered.
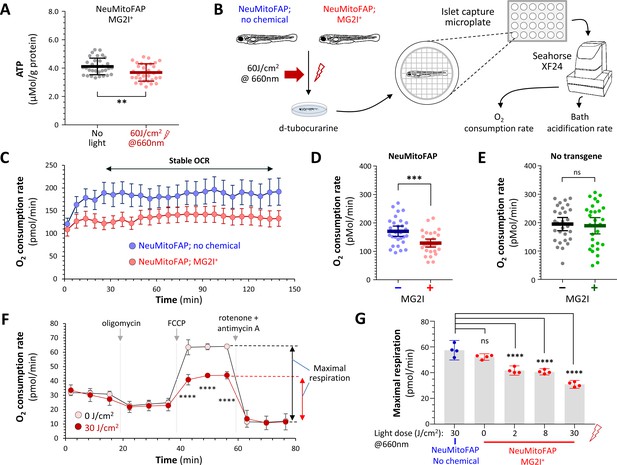
Disruption of mitochondrial function in NeuMitoFAP zebrafish exposed to MG2I and far-red light.
(A) ATP concentration was measured in lysates (each containing 5 whole zebrafish larvae) using a bioluminescent assay and normalized to protein content. Data points show ATP/protein in individual lysates, bars show group mean ± 95% CI. Experimental groups: NeuMitoFAP zebrafish treated with MG2I (black); NeuMitoFAP zebrafish treated with MG2I and exposed to 60 J/cm2 far-red light (red). **p<0.006, unpaired 2-tailed t-test. Graphs show results from four combined replicate experiments (n = 32 per group). (B) Design of experiments shown in panels C – E and Figure 4—figure supplement 2. (C) Oxygen consumption rate (OCR; y-axis) against time (x-axis) for NeuMitoFAP zebrafish exposed to far-red light in the presence (red) or absence (blue) of MG2I. Points show mean ± SE (n = 8 zebrafish larvae per group) in a single experiment. The period of stable OCR analyzed in panels D and E is indicated. (D, E) Stable OCR for (D) NeuMitoFAP and (E) WT zebrafish exposed to far-red light in the presence (+) or absence (−) of MG2I. Data points show values for individual zebrafish larvae. Bars show mean ± SE for 24 zebrafish per group, combined from 3 replicate experiments. ***p=0.00053, 2-tailed unpaired t-test. (F) OCR measurements for dissociated brain cells derived from NeuMitoFAP zebrafish. Cells were exposed to MG2I and far-red light (filled circles) or no light (open circles) after dissociation; OCR was measured dynamically at baseline and after exposure to oligomycin (ATP synthase inhibitor; shows proportion of OCR linked to ATP synthesis), FCCP (mitochondrial uncoupler; allows calculation of maximal respiration) and rotenone + antimycin-A (complex I and III inhibitors; shows proportion of measured OCR attributable to mitochondrial respiration). The calculation for maximal respiratory rate = OCR(FCCP) – OCR(rotenone + antimycin-A) is shown schematically to the right of the graph. Data points show mean ± SE for five samples per group. ***p<0.001, no light versus 30 J/cm2, 2-way repeated measures ANOVA with Šidák multiple comparisons test. (G) Dose-response curve for maximal respiration versus far-red light dose in NeuMitoFAP dissociated brain cells in the presence (right, red) or absence (left, blue) of MG2I. Bars show mean ± 95% CI, data points show replicate assays n = 5 per point. ****p<0.0001 versus no chemical control, 1-way ANOVA with Dunnett’s post hoc test.
-
Figure 4—source data 1
Source data for Figure 4A.
- https://cdn.elifesciences.org/articles/51845/elife-51845-fig4-data1-v1.xlsx
-
Figure 4—source data 2
Source data for Figure 4B.
- https://cdn.elifesciences.org/articles/51845/elife-51845-fig4-data2-v1.xlsx
-
Figure 4—source data 3
Source data for Figure 4D-E.
- https://cdn.elifesciences.org/articles/51845/elife-51845-fig4-data3-v1.xlsx
-
Figure 4—source data 4
Source data for Figure 4F.
- https://cdn.elifesciences.org/articles/51845/elife-51845-fig4-data4-v1.xlsx
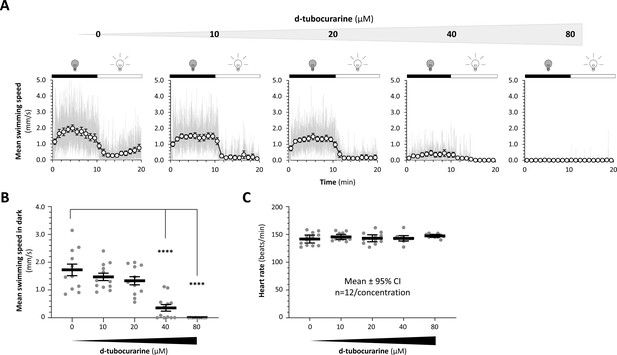
Viability of zebrafish larvae following neuromuscular paralysis with curare.
It was necessary to prevent swimming movements during oximetry measurements (Figure 4) because: (i) movements of larvae could disrupt measurement of dissolved O2 in the water; and (ii) the large difference in motor activity between paralyzed NeuMitoFAP-MG2I animals and controls could cause secondary differences in O2 consumption attributable to muscle O2 consumption and unrelated to changes in neuronal respiration. (A) Larval zebrafish were paralyzed using d-tubocurarine at increasing concentrations as shown above the graphs. Visual motor responses were evaluated identically to Figure 2—figure supplement 1 in groups of 12 zebrafish at each concentration. The dark and light phases of the stimulus is show above each graph. The light tracing shows instantaneous swimming speed at each frame transition. The dark circles show mean ± SE swimming speed for all 12 zebrafish in each minute of the recording. Swimming movements in both light and dark phases of the response were abolished at higher concentrations of d-tubocurarine. (B) Mean swimming speed during the dark phase of the visual motor response was calculated. The scatter plot shows values for individual zebrafish (gray data points) with group mean ± SE superimposed (black bars). Motility was completely abolished in zebrafish exposed to 80 μM d-tubocurarine (****p<0.00001, compared with 0 μM, 1-way ANOVA, with Dunnett’s post hoc test). (C) Regardless of neuromuscular paralysis, zebrafish at all concentrations of d-tubocurarine were viable and showed normal heart rate, indicating that 80 μM tubocurarine was adequate to immobilize zebrafish in the Seahorse instrument for O2 consumption measurements without altering viability.
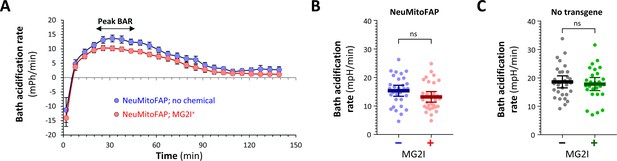
No compensatory increase in glycolysis after mitochondrial targeting in NeuMitoFAP zebrafish.
Bath acidification rate (BAR) was quantified at the same time as the oxygen consumption rate measurements shown in Figure 4, using a Seahorse XF24 flux analyzer. (A) In the first 30 min of measurement, bath acidification rapidly increased, reaching its peak at 25–45 min. This phasic response was identical in all four experimental groups. For quantification and statistical comparison in panels B and C, we analyzed the peak BAR between 25–45 min. (B, C) Peak BAR for (B) NeuMitoFAP and (C) WT zebrafish exposed to far-red light in the presence (+) or absence (−) of MG2I. Data points show values for individual zebrafish larvae. Bars show mean ± SE for 24 zebrafish per group, combined from 3 replicate experiments.
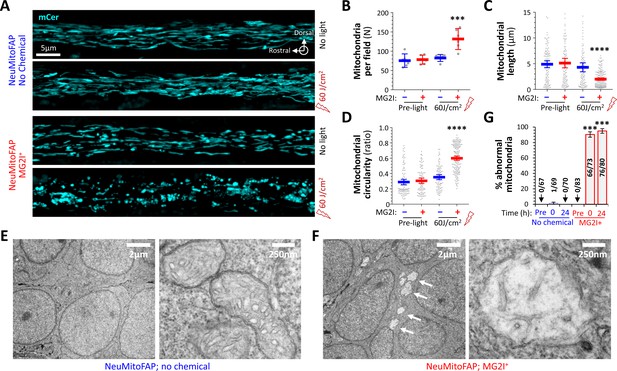
Disruption of mitochondrial structure in NeuMitoFAP zebrafish exposed to MG2I and far-red light.
(A) Confocal Z-plane projections showing mCerulean-labeled mitochondria in the lateral line nerves of live NeuMitoFAP zebrafish in the absence (upper images) or presence (lower images) of MG2I, before (upper image of each pair) or after (lower image of each pair) exposure to 60 J/cm2 light at λpeak=661 nm. (B - D) The (B) number of mitochondria per field, (C) mitochondrial length, and (D) mitochondrial circularity were quantified in z-plane projections of the entire medio-lateral extent of the lateral line nerve in 6 zebrafish per group. Bars show mean ± 95% CI for NeuMitoFAP zebrafish in the absence (blue) or presence (red) of MG2I, before or after exposure to far-red light. Data points show individual zebrafish (panel B) or individual mitochondria (panels C, D). ***p<0.001, ****p<0.0001, NeuMitoFAP-MG2I post-light versus each other group individually, 1-way ANOVA with Tukey multiple comparisons test. (E, F) Transmission electron micrographs of sections from the telencephalon of NeuMitoFAP zebrafish immediately after exposure to far-red light in the (F) presence or (E) absence of MG2I. The left image of each pair shows a low-magnification view, and the right image shows a high-magnification view illustrating the ultrastructure of individual neuronal mitochondria. Arrows in panel F show swollen, damaged mitochondria. (G) The proportion of abnormal mitochondria was quantified by a blinded observer in 12 electron micrographs each from 6 experimental groups (NeuMitoFAP with or without MG2I, before, 0 or 24 hr after 60 J/cm2 far-red light exposure). Bars show mean ± SE% abnormal mitochondria per section in each group. Numbers of total and abnormal mitochondria in each group are shown. ***p<0.001, compared individually with each control (pre-light and no chemical) group, 1-way ANOVA with Tukey multiple comparisons test.
-
Figure 5—source data 1
Source data for Figure 5B.
- https://cdn.elifesciences.org/articles/51845/elife-51845-fig5-data1-v1.xlsx
-
Figure 5—source data 2
Source data for Figure 5C.
- https://cdn.elifesciences.org/articles/51845/elife-51845-fig5-data2-v1.xlsx
-
Figure 5—source data 3
Source data for Figure 5D.
- https://cdn.elifesciences.org/articles/51845/elife-51845-fig5-data3-v1.xlsx
-
Figure 5—source data 4
Source data for Figure 5G.
- https://cdn.elifesciences.org/articles/51845/elife-51845-fig5-data4-v1.xlsx
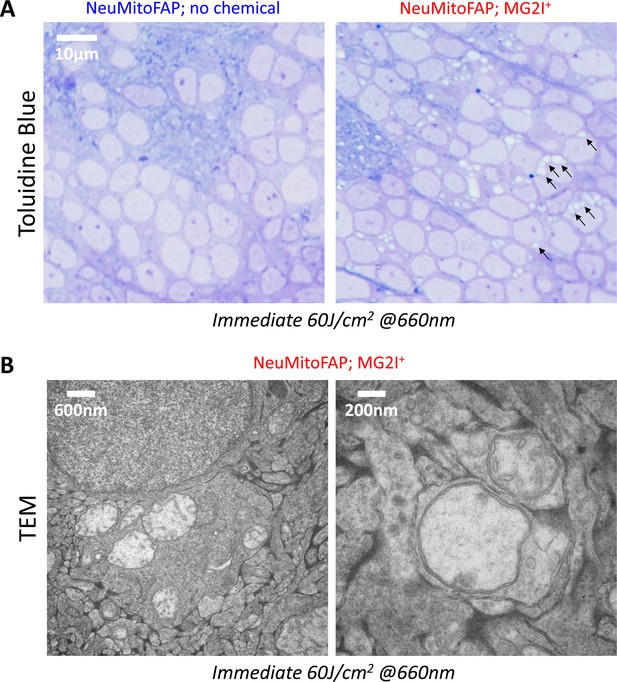
Widespread disruption of mitochondrial morphology in NeuMitoFAP zebrafish exposed to MG2I and far-red light.
NeuMitoFAP zebrafish larvae were treated with MG2I or no chemical from 3 to 5 dpf and then exposed to 60 J/cm2 light at λpeak=661 nm, following which they were fixed immediately for electron microscopy. (A) Toluidine Blue-labeled thin sections of the telencephalon, showing the widespread appearance of lucent areas in the cytoplasm of NeuMitoFAP-MG2I neurons following light exposure (right panel; examples of lucent areas are indicated with arrows). These abnormalities were not present in controls that were not treated with MG2I (left panel). (B) Transmission electron microscopy images showing that the lucent areas correspond to severely damaged mitochondria that were swollen and lacked cristae. Multiple abnormal mitochondria were frequently seen within the same cell (left). Severely damaged mitochondria were also apparent within axons and terminals (right) corresponding to the fragmented and swollen axonal mitochondria seen in Figure 5A.
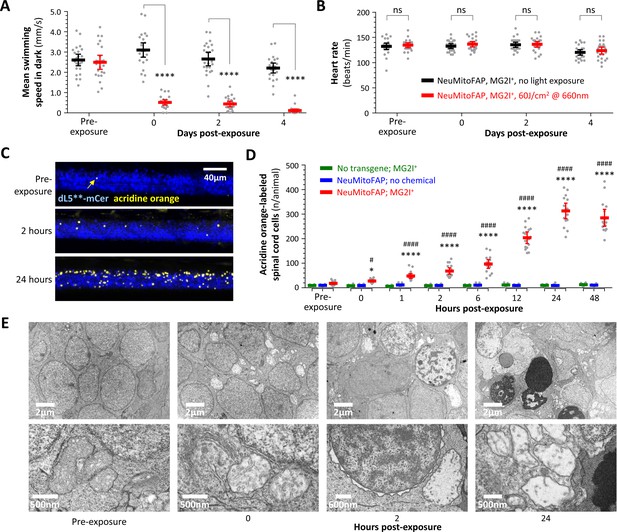
Persistent neurological deficits and cell death in NeuMitoFAP zebrafish exposed to MG2I and far-red light.
(A) Mean swimming speed of NeuMitoFAP zebrafish treated with MG2I was quantified during the dark phase of the visual motor response (y-axis) after exposure to 60 J/cm2 far-red light (red) or no exposure (black). Data points show responses of individual zebrafish before light exposure and afterwards at the time points indicated (x-axis). Bars show group mean ± 95% CI. ****p<0.0001, 2-way ANOVA with Šidák multiple comparisons test. (B) Heart rate (y-axis) was quantified in the same experimental groups and time points as panel A. Data points show heart rates of individual zebrafish, bars show group mean ± 95% CI. (C) Degenerating cells in MG2I-treated NeuMitoFAP zebrafish were labeled with acridine orange, before, and 2 and 24 hr after exposure to 60 J/cm2 far-red light. The images show confocal z-plane projections through the spinal cord of immobilized live zebrafish larvae. dL5**-mCerulean3 is pseudocolored blue and acridine orange-labeled cells yellow. (D) Acridine orange-labeled cells in the spinal cord of live zebrafish were counted (y-axis), before far-red light exposure and at the indicated time points afterwards (x-axis). Experimental groups: WT zebrafish treated with MG2I (green), NeuMitoFAP zebrafish (blue), NeuMitoFAP zebrafish treated with MG2I (red). Data points show individual zebrafish, bars show mean ± SE. *p<0.05, ****p<0.0001, NeuMitoFAP-MG2I zebrafish versus other groups at the same time point; #p<0.05, ####p<0.0001, NeuMitoFAP-MG2I zebrafish at the indicated time point versus pre-exposure value; 2-way ANOVA with Tukey multiple comparisons test. (E) Transmission electron micrographs of sections from the telencephalon of NeuMitoFAP zebrafish treated with MG2I before, and at the indicated time points after, far-red light exposure. The upper image of each pair shows a low-magnification view, and the lower image shows a high-magnification view illustrating ultrastructural features.
-
Figure 6—source data 1
Source data for Figure 6D.
- https://cdn.elifesciences.org/articles/51845/elife-51845-fig6-data1-v1.xlsx
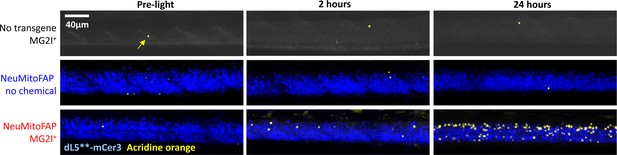
Delayed CNS cell death in NeuMitoFAP zebrafish exposed to MG2I and far-red light.
Similar micrographs are shown as in Figure 6C, and quantified in Figure 6D, but including the control groups in comparison with NeuMitoFAP-MG2I larvae, before exposure, 2 hr after, and 24 hr after exposure to 60 J/cm2 far-red light at λpeak=661 nm. Segments of the spinal cord of live anesthetized zebrafish larvae were imaged using confocal microscopy for mCerulean3 (pseudocolored blue) and Acridine Orange (pseudocolored yellow). Under the zebrafish eno2 promoter element (Bai et al., 2007), the dL5**-mCerulean3 fusion protein is expressed in the mitochondria of most neurons in NeuMitoFAP zebrafish, so the spinal cord is outlined by the mCerulean3 fluorescence signal, providing a convenient anatomical landmark. There is no mCerulean3 signal in non-transgenic zebrafish (top row). The number of Acridine Orange-labeled cells in the spinal cord increased dramatically in NeuMitoFAP-MG2I zebrafish, but not controls, following exposure to far-red light (see Figure 6D for quantification and statistical analysis).
Videos
Loss of motor function in NeuMitoFAP-MG2I zebrafish following far-red light exposure.
5dpf zebrafish swimming in the wells of an agarose-filled plate were illuminated from above by an infrared light, and video was recorded from below at 30 frame/s, during the dark phase of the visual motor response (VMR). Each well contains 5 zebrafish from the experimental groups indicated: top left – NeuMitoFAP zebrafish exposed to MG2I and 60 J/cm2 far-red light; top right – NeuMitoFAP zebrafish exposed to 60 J/cm2 far-red light in the absence of MG2I; bottom left – NeuMitoFAP zebrafish treated with MG2I but not exposed to far-red light; bottom right – WT zebrafish exposed to MG2I and 60 J/cm2 far-red light. Dramatic abnormalities of motor function are clearly visible in the NeuMitoFAP-MG2I-light group, but not in any of the controls.
Normal circulation and heartbeat in NeuMitoFAP-MG2I zebrafish following far-red light exposure.
(i) Phase contrast videomicrography of 5dpf zebrafish from the same experimental groups as Video 1, illustrating identical circulation of blood cells in the vascular system from all experimental groups (zebrafish oriented rostral towards left); (ii) high-power videomicrography from a zebrafish in the NeuMitoFAP-MG2I-light group that showed profound loss of neurological function. Anatomical landmarks are labeled for orientation (rostral left). (iii) The heart is shown from zebrafish in all four experimental groups, illustrating normal contraction and heart rate. These data show that abnormalities in NeuMitoFAP zebrafish exposed to MG2I and far-red light are confined to the nervous system and that the zebrafish remain alive and viable despite profound neurological disruption.
Persistent motor deficits in NeuMitoFAP-MG2I zebrafish following far-red light exposure.
7dpf zebrafish are shown 48 hr after far-red light exposure at 5dpf. Methods and experimental groups are identical to Video 1. The severe neurological abnormalities seen immediately after far-red light exposure at 5dpf persist in the NeuMitoFAP-MG2I-light group after two days of recovery.
Normal circulatory and cardiac development in NeuMitoFAP-MG2I zebrafish following far-red light exposure.
Phase contrast videomicrography of 7dpf zebrafish 48 hr after far-red light exposure at 5dpf. Methods and experimental groups are identical to Video 2. Despite the persistence of severe neurological abnormalities two days after light exposure, circulation and heartbeat in the NeuMitoFAP-MG2I-light group remain normal, showing that irreversible abnormalities are restricted to the nervous system.
Tables
Peak wavelength, centroid and full width at half height (FWHH) are shown for the red, green, and infrared LED sources used in the study, in comparison with the major and minor excitation peaks of the dL5**-MG2I complex (see Figure 1H).
Red | Green | IR | dL5**-MG2I (minor) | dL5**-MG2I (major) | |
---|---|---|---|---|---|
Peak λ (nm) | 661 | 516 | 877 | 456 | 666 |
Centroid λ (nm) | 656 | 520 | 880 | 452 | 649 |
FWHH (nm) | 18 | 36 | 51 | 55 | 61 |
Peak wavelength, peak height and area under the curve are shown for normalized energy transfer spectra between the 661 nm LED and the dL5**-MG2I complex (‘Red x dL5**-MG2I’) and between the 516 nm LED and the dL5**-MG2I complex (‘Green x dL5**-MG2I’; see Figure 1—figure supplement 2).
Red x dL5**-MG2I | Green x dL5**-MG2I | |
---|---|---|
Peak λ (nm) | 661 | 524 |
Height | 0.98 | 0.013 |
Area | 19.10 | 1.15 |