Feedback inhibition and its control in an insect olfactory circuit
Figures
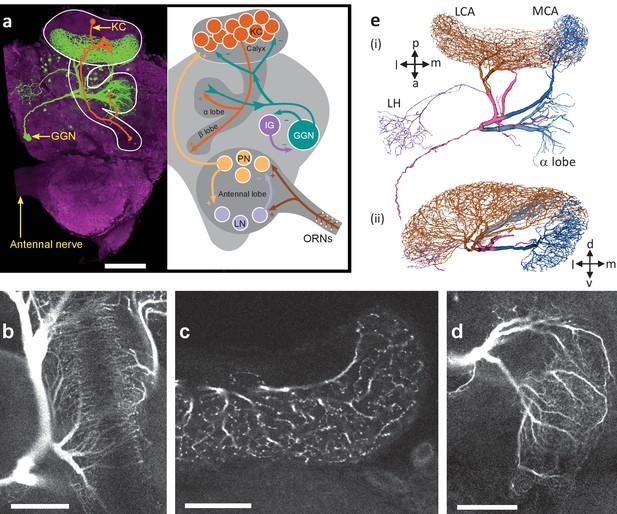
GGN is a very large neuron, one per hemisphere, spanning a large portion of the locust brain.
(a) Left hemisphere: Dye filled GGN (green) in a locust brain (magenta, visualized with nc82 antibody) with an overlaid KC tracing (orange, filled in a different brain). Dorsal: towards the viewer; ventral: into the page). Right hemisphere: Circuit diagram of the locust olfactory system. Arrows show known synaptic connections, + excitatory, - inhibitory. Scale bar: 200 μm. (b) Very thin, feather-like neurites from GGN wrap around and penetrate the peduncle of the mushroom body; these neurites are not included in our reconstruction. (c) GGN’s neurites in the calyx have many bouton-like protrusions (d) whereas GGN’s α lobe branches are relatively smooth. (b–d) scalebars: 100 μm. (e) 3D reconstruction of the same neuron shown in panel (a) viewed from (i) ventral and (ii) posterior side of the brain. Major branches shown in different colors. LCA: lateral calyx, MCA: medial calyx, LH: lateral horn, a: anterior, p: posterior, d: dorsal, v: ventral, l: lateral and m: medial.
3D view of the confocal stack of the dye filled GGN in Figure 1a.
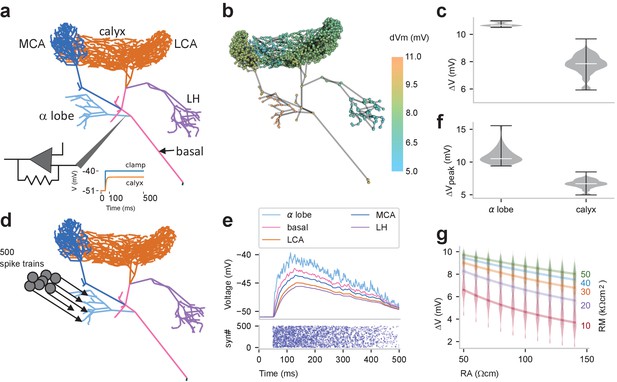
Passive voltage spread through GGN.
(a) Simulation schematic: GGN’s α lobe branch was clamped at its stem to −40 mV (from a holding voltage of −51 mV). (b) Conditions described in panel a lead to different steady state depolarizations of the GGN model at different locations; color indicates voltage change from resting potential; axial resistivity RA = 100 Ωcm; membrane resistivity RM = 33 kΩcm2. (c) Violin plots showing distribution of depolarization in the α lobe and the calyceal dendrites of GGN for conditions described in panel a; width of gray area indicates density, white line median, and whiskers data range. (d) Schematic of simulation of multiple, random synaptic inputs to GGN: 500 synapses were connected to GGN’s α lobe branch. (e) Bottom: raster plot of incoming spike times at all 500 synapses. The spikes arrived at each synapse at random times at linearly decreasing rate, starting with 20/s down to 0 after 500 ms. Top: for conditions shown in panel d, membrane potentials at randomly sampled terminal neurites in different regions of GGN. (f) Violin plots of peak depolarizations in the α lobe dendrites and the calyceal dendrites in model described in panel d. (g) Violin plots of distribution of depolarizations in the calyx for different values of axial resistivity (RA, bottom) and membrane resistivity (RM, right) for the voltage clamp simulation described in panel a. Lines connect the median results for specified RMs (right).
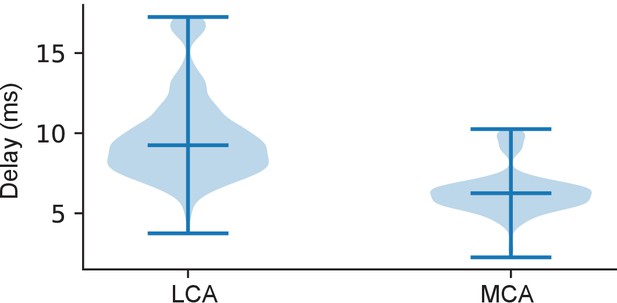
Signal propagation time throughout GGN.
Figure shows the time of peak depolarization at the lateral calyx (LCA) and medial calyx (MCA) branches of GGN with respect to that at the base of the α lobe branch in the simulation described in Figure 2d,e. Darker line in the middle indicates median and whiskers data range. The voltages were smoothed by 100 Hz lowpass filter.
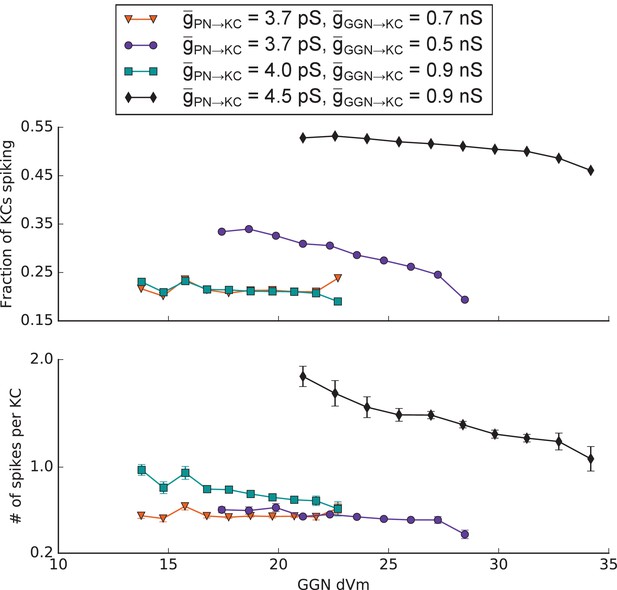
KC spiking vs GGN voltage deflection in simulations where all KCs received identical PN input for different values of maximum synaptic conductances onto KCs.
GGN segments were grouped by peak depolarization from the resting potential (dVm) and the total number of spiking KCs postsynaptic to these segments (top) or the total number of spikes in the KCs postsynaptic to these segments (bottom) were normalized by the total number of KCs (both spiking and nonspiking) post synaptic to these segments.
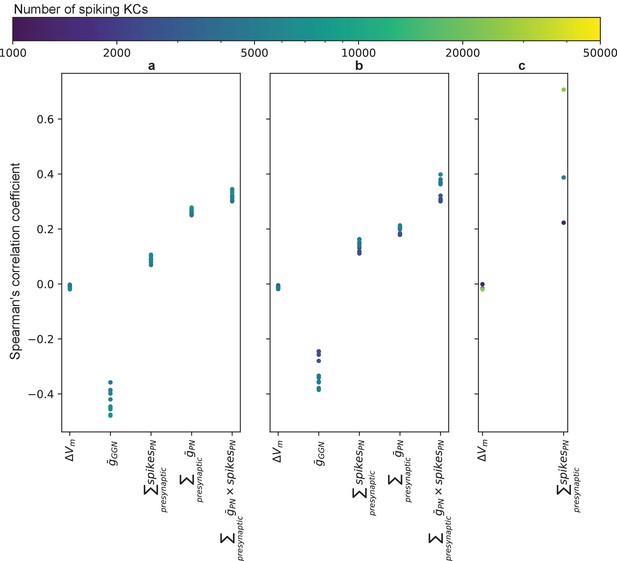
Correlations between number of spikes in a KC and various parameters influencing spiking: ΔVm – peak voltage deflection of its presynaptic GGN segment, – maximum conductance of the synapse from GGN, – total number of spikes over all its presynaptic PNs, – sum of maximum conductance of all PN synapses, – sum of the numbers of spikes in its presynaptic PNs weighted by the maximum conductances of their synapses onto this KC.
The negative correlation between the number of spikes in a KC and depolarization of its presynaptic GGN segment is very small in simulations of (a) models with temporally patterned PN response (as in Figure 5e) and synaptic strengths onto KCs selected from lognormal distributions, (b) models with steady activity in a fixed set of PNs (as in Figure 5b) and synaptic strengths onto KCs selected from lognormal distributions, and (c) models with steady activity in a fixed set of PNs and constant synaptic strengths onto KCs. Color indicates the number of KCs that spiked in the simulation.
3D view of depolarizations (color coded spheres) at various locations in GGN arbor in simulation described in Figure 2a.
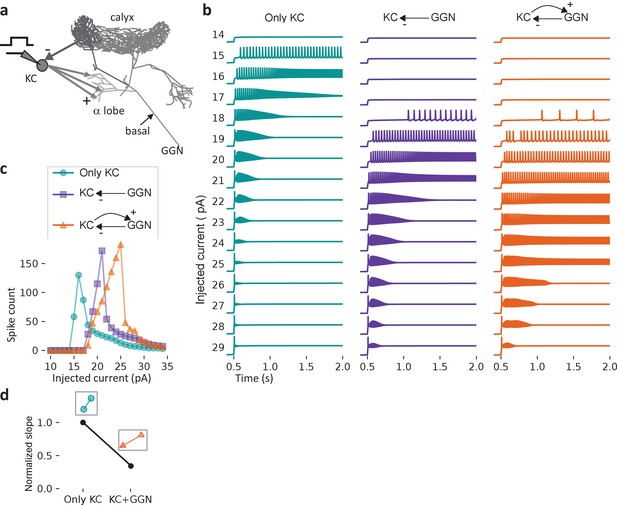
Feedback inhibition from GGN extends the dynamic range of KCs.
(a) Model schematic: A single KC sends 50,000 excitatory synapses with random delays of 0–60 ms into GGN’s α lobe branch. The KC receives feedback inhibition via a graded synapse from GGN’s calyceal branch. A step current is injected into the KC from 0.5 to 3 s (up to 2 s is shown). (b) The model KC was tested with current pulses of increasing amplitude in three configurations: Isolated KC (left); KC receiving only spontaneous inhibition from GGN (middle), and KC receiving spontaneous and odor-elicited feedback inhibition (right). As the amplitude of the current pulse increases, the KC’s spiking first increases, and then, as the membrane potential nears saturation, decreases. (c) Comparison of the number of spikes evoked by current steps in KC in the three configurations shown in b). (d) Slopes of the rising parts of the KC-only (left, cyan) and KC with GGN feedback (right, orange) curves in c), normalized by the KC-only slope.
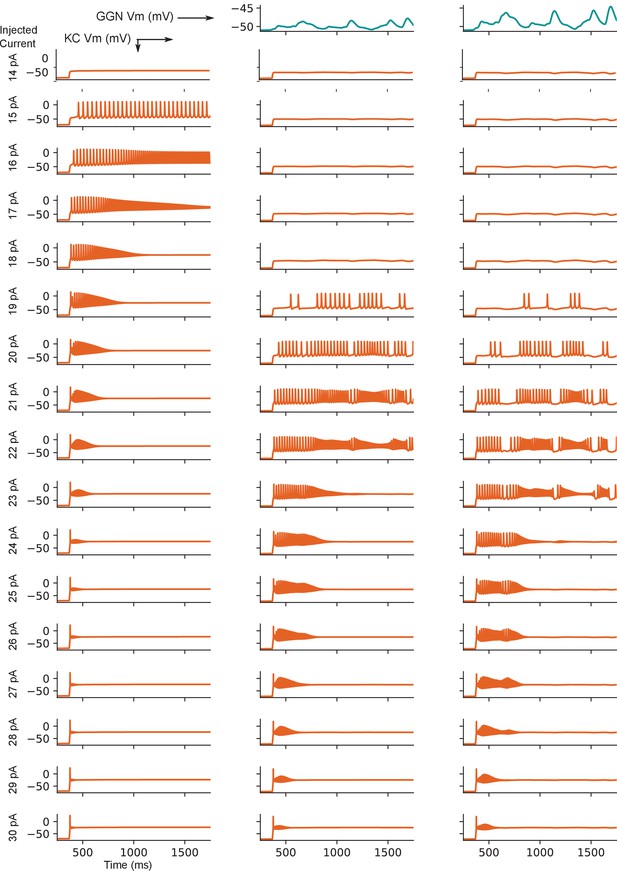
Model KC responses to current step injections while inhibited by pseudo-dynamic clamp of GGN voltage from a network model show inhibition expands dynamic range of KCs.
The test KC received no inhibition (left column), inhibition driven by weak GGN depolarization (middle column), or strong GGN depolarization (right column). The strong GGN voltage was obtained from the simulation of a full PN-KC-GGN network model. The weak GGN depolarization was derived by halving the deflections of the former from resting potential.
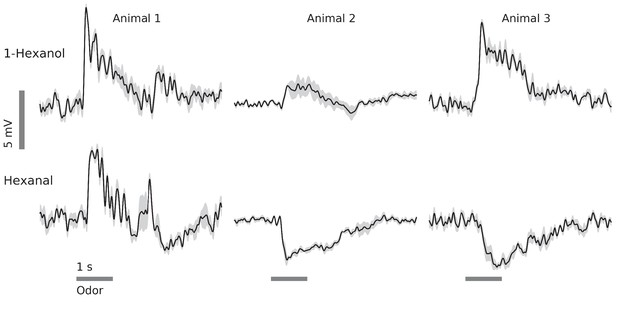
In vivo, GGN’s responses to odors vary with odor and animal.
Examples from 3 animals and two odors (horizontal gray bars, 1 s) (black traces: average of 5 trials, gray: standard error of the mean, data low-pass filtered at 49 Hz). Response features include depolarization or hyperpolarization upon stimulus onset and/or offset, and depolarization or hyperpolarization evoked by different odors in the same GGN.
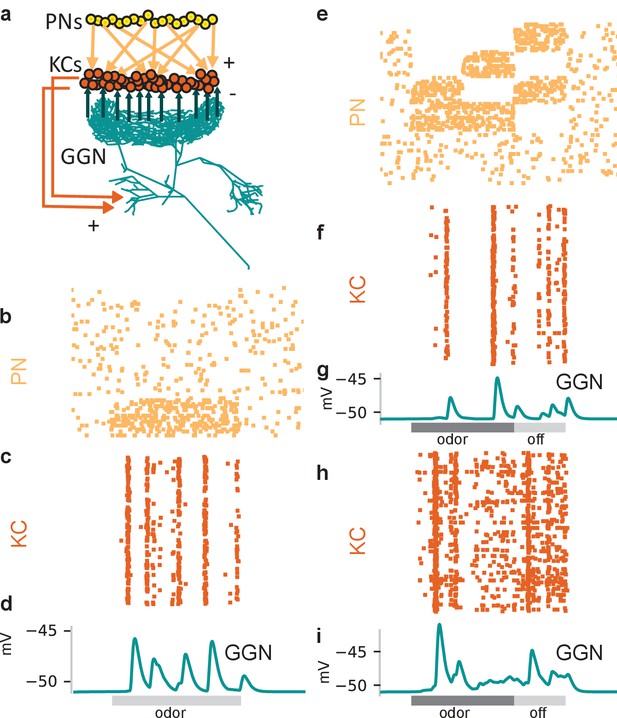
Tuning the olfactory network by reference to GGN’s olfactory responses required heterogeneous synaptic strengths onto KCs and structured patterns of activity in PNs.
(a) Schematic of mushroom body network model. Each of the 50,000 KCs receives input from 50% of the 830 PNs, which are modeled as spike trains. All KCs excite GGN in its α lobe branch and receive inhibition from a random calyceal branch of GGN. (b–d) Model with simplified, homogenous firing patterns in PNs and uniform synaptic strengths generates unrealistic membrane potential in GGN. (b) Raster plot of model PN spike trains (67 shown); dots in each row mark spike times in a PN. (c) Raster plot of spike trains evoked in the KCs (397 shown) when all of them receive identically strong inhibitory connections from GGN. (d) Unrealistic membrane potential in GGN features a few peaks corresponding to highly synchronized bouts of activity in KCs. Light gray bar: 1 s odor stimulation. (e–g) Model in which subpopulations of PNs have different temporal patterns of spiking during and after odor stimulation generates unrealistic membrane potential in GGN. (e) Rasters show different firing patterns in different PNs. (f) Simulation of model with PN activity pattern in panel e) along with uniform synaptic strengths onto KCs generate synchronized spiking in KCs and (g) unrealistic membrane potential in GGN. Simulation of a model with both structured PN activity patterns in panel e) and heterogeneous synaptic strengths gives rise to (h) temporally diffuse spiking in KC population and (i) sustained depolarization of GGN similar to that observed in vivo (e.g. Figure 4 animal 1). Dark gray bar: 1 s odor stimulation; light gray bar: 0.5 s ‘off response’ period.
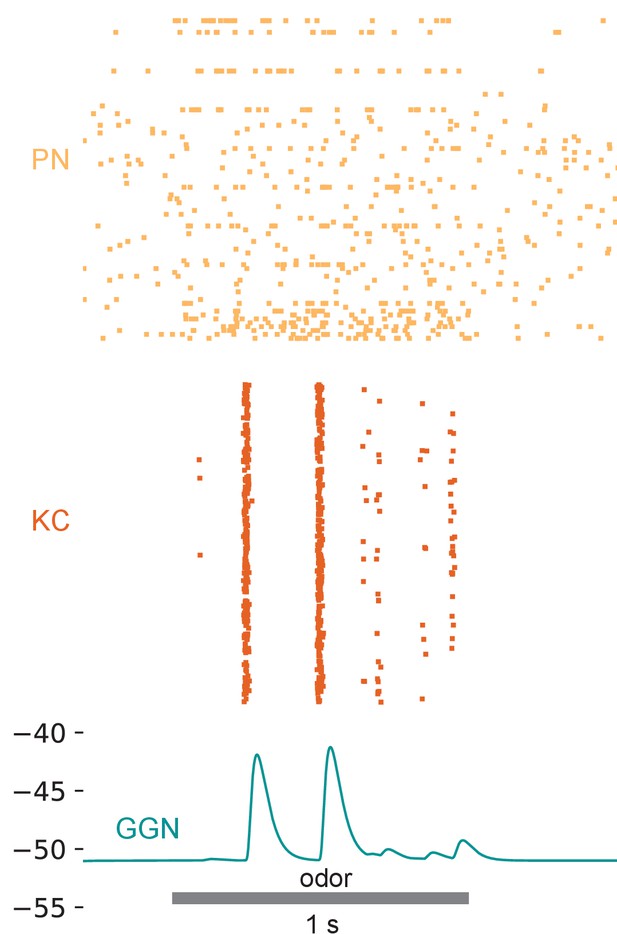
Introducing diverse delays in PN to KC synapses does not alleviate the unrealistic extent of synchronization of KC firing.
This model had architecture similar to that in Figure 5b–d, but here the synaptic delays from PNs to KCs were sampled from a normal distribution (mean = 7.5 ms, standard deviation = 3 ms).
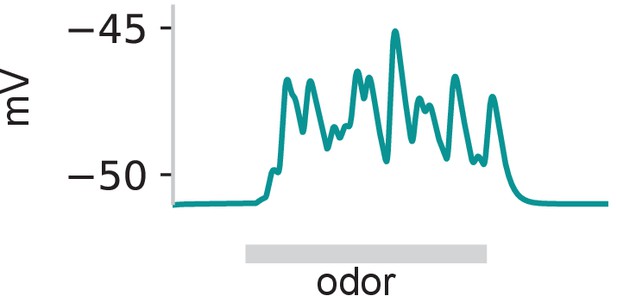
Model with steady activity in a fixed set of PNs (as in Figure 5b) can produce sustained depolarization of GGN when the synaptic strengths are lognormally distributed.
Gray: 1 s odor stimulus.
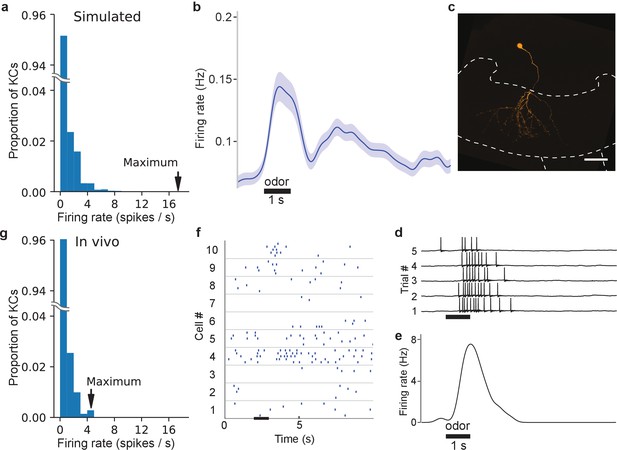
In vivo, some KC responses are hyperactive, as predicted by simulations.
(a) Distribution of KC firing rates in simulation producing realistic GGN depolarization. (b) KC firing rate averaged over 707 KC-odor pairs. Shaded region indicates standard error of the mean. Black horizontal bar: 1 s odor stimulation. (c) A hyperactive response KC filled with dye (mushroom body calyx and pedunculus outlined with dashed lines (scale bar: 50 μm) and (d) its recorded membrane potential in response to odor stimulus, and (e) its average firing rate elicited by this stimulus computed in a 100 ms window. (f) Sample KC spike trains. (g) Histogram of average firing rate of KCs across trials upon odor presentation measured in a 1.4 s window. The last bin in the rightward tail includes two different KCs.
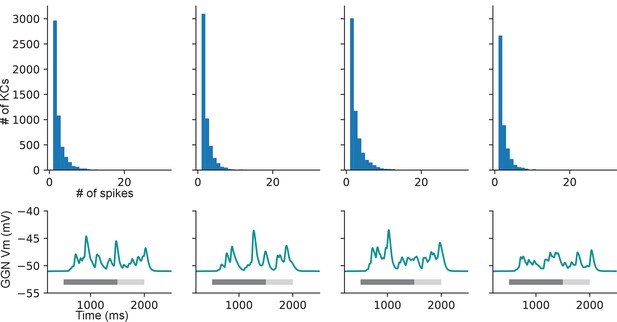
Example distributions of KC spike counts in four instantiations of the network architecture in Figure 6.
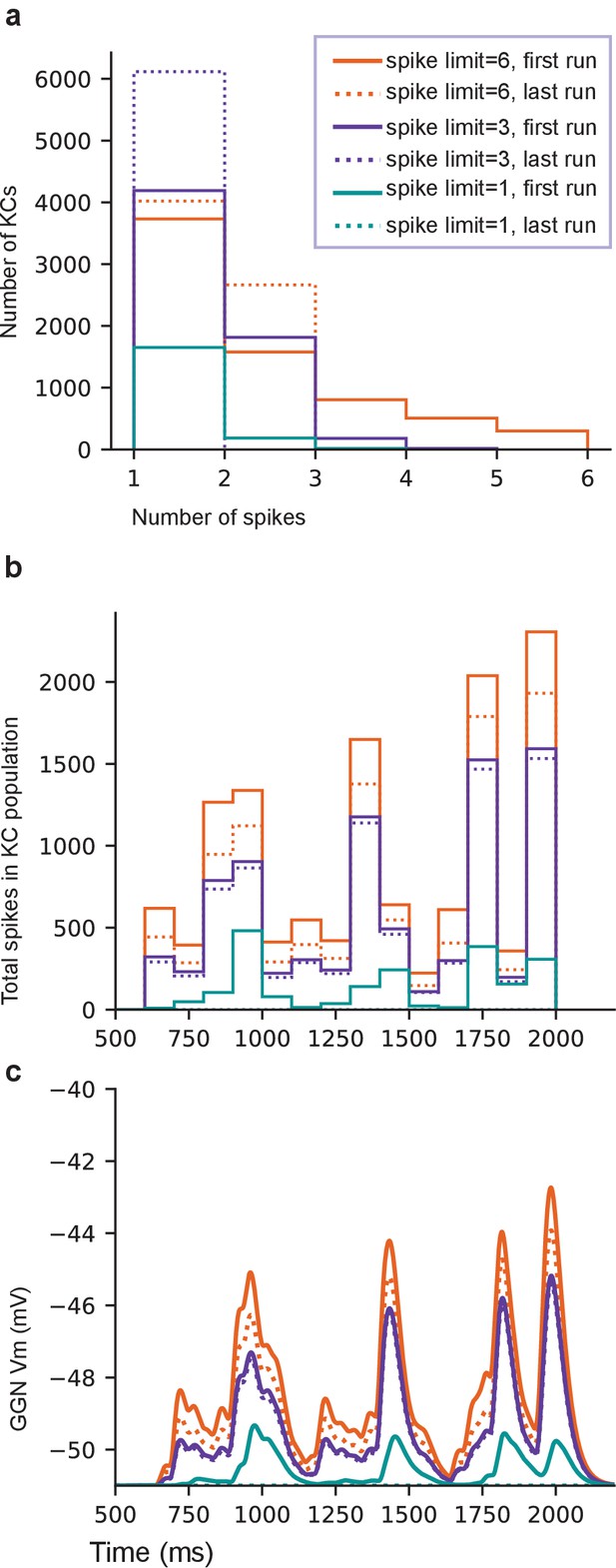
High spike-rate KCs contribute to sustaining GGN’s odor-elicited depolarization.
(a) Histogram of spike counts in KCs for one template network at the first and the last run of each spike count limit. (b) Population spike time histogram of KCs (100 ms bins) for the same simulations. (c) GGN membrane potential. See text for the procedure. Legend is same for a–c.
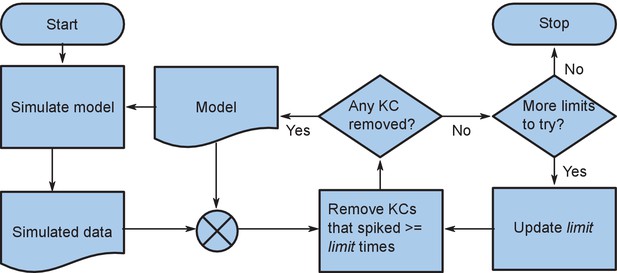
Flow chart of the reiterative simulation of the same model while removing high-firing KCs.
The KCs which spiked more than limit times in a simulation were eliminated from the model and this updated model was simulated again. The number of KCs producing more than limit spikes generally reduced after this, and as the process was repeated, at some point no KC met this criterion (i.e. no KC was removed from the model). Then we lowered the limit and repeated the process. This was continued until none of the KCs were able spiked.
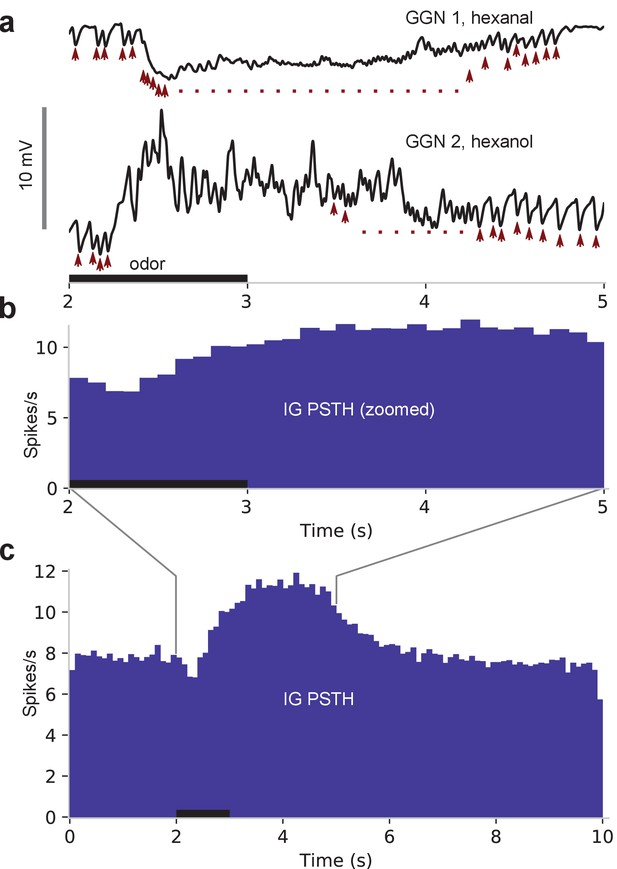
IPSPs in GGN suggest IG spikes upon odor presentation and is responsible for hyperpolarizations in GGN.
(a) In vivo recordings of GGN’s membrane potential from two animals showing IPSPs (arrowheads) believed to originate as spikes in IG. Vertical scale bar: 10 mV. (b) Peristimulus time histogram (PSTH) shows IPSP peak times from 1257 odor-trials across 47 GGNs, presumably reflecting spikes in IG. Because IG begins to spike before GGN’s membrane potential returns to baseline, IG’s odor response cannot be driven by disinhibition from GGN. Black horizontal bar: odor presentation in panels (a) and (b), which share the time axis. (c) Same as panel (b) but showing full responses.
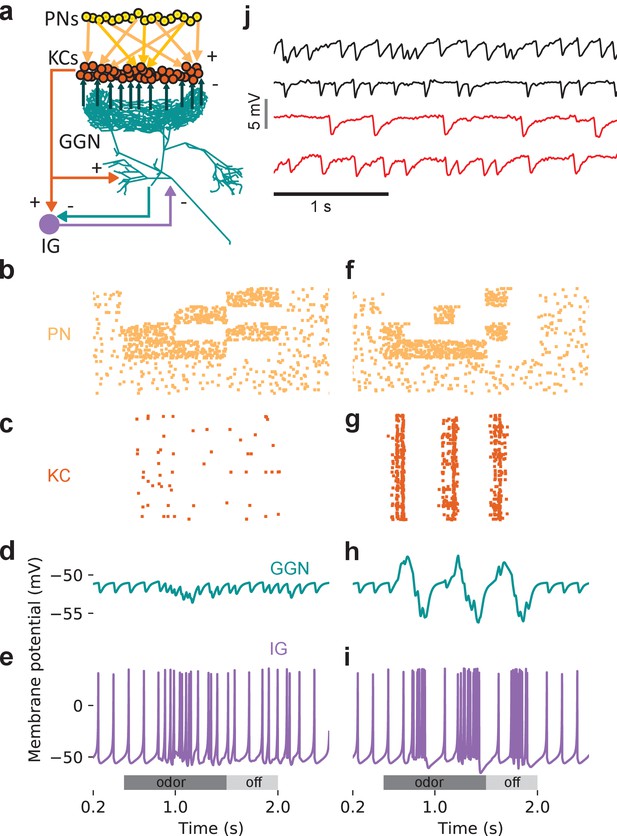
An additional, unidentified olfactory pathway to IG is needed to explain odor-elicited hyperpolarization in GGN.
(a) Schematic of model with IG receiving direct excitation from KCs and reciprocal inhibition from GGN. (b) Spike raster of PN activity producing GGN hyperpolarization. (c) Spike raster of KC population activity in the same simulation. (d) Simulated GGN membrane potential including odor-elicited hyperpolarization mimics responses observed in vivo (e.g. Figure 4, Animal 3, hexanal). (e) Corresponding simulated IG membrane potential. This simulation included a 200 ms synaptic delay from KCs to IG. (f–i) Varying the temporal pattern of PN population activity can produce different response pattern in the same network as e. (f) Raster plot of PN activity with a different temporal pattern from that in e. (g) Spike raster showing concomitant KC activity. (h) Simulated GGN membrane potential including odor evoked de- and hyperpolarization (similar to Figure 4, Animal one hexanal). (i) Corresponding simulated IG membrane potential. (j) Spontaneous activity in IG does not originate in the antennal lobe. Top two black traces show spontaneous IPSPs in GGN’s membrane voltage from two animals with intact olfactory systems. The bottom two red traces are from the left and the right GGN in another animal in which the antennal lobes had been silenced by cutting both antennal nerves. Vertical scale bar 5 mV, horizontal scale bar 1 s.
Tables
Reagent type (species) or resource | Designation | Source or reference | Identifiers | Additional information |
---|---|---|---|---|
Software, algorithm | NEURON | https://neuron.yale.edu/neuron/ | RRID:SCR_005393 | Versions 7.4 and 7.5 with Python 2.7 |
Software, algorithm | NeuroLucida 360 | https://www.mbfbioscience.com/neurolucida360 | RRID:SCR_016788 | |
Chemical compound, drug | Neurobiotin | Vector Laboratories | SP-1120 | 1–5% in 2–3M potassium acetate |
Chemical compound, drug | Avidin-Alexa complex | Thermo Fisher Scientific | S11226 | 0.01 mg/ml in 1X PBS |