Structural insights into sodium transport by the oxaloacetate decarboxylase sodium pump
Figures
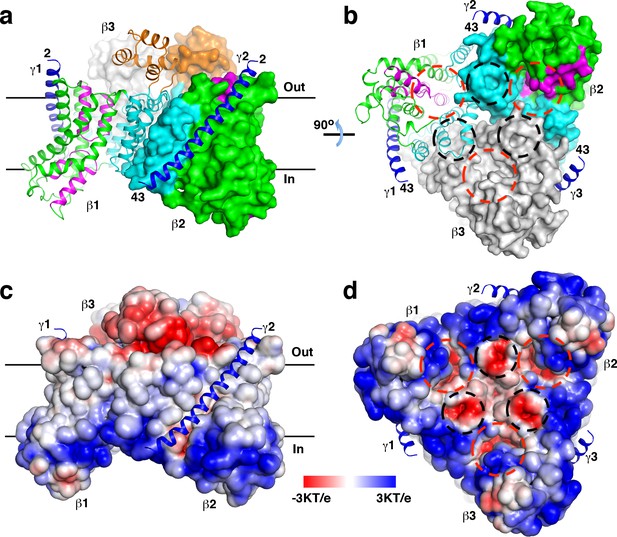
Overall structure of the StOAD βγ sub-complex.
(a)-(b) Structure of the StOAD β3γ3 hetero-hexamer. The γ subunits are colored in blue and shown in cartoon representation. The first and second β subunits (β1 and β2) are colored with the scaffold domain in cyan, core domain in green, domain E in orange and the helical hairpins in magenta. This coloring scheme is used throughout the manuscript unless otherwise indicated. The third β subunit (β3) is colored in gray. Cartoon representation of β1 and the molecular surfaces of β2 and β3 are shown. The first and last residues in the γ subunit visible in our structure are indicated (residues 2 and 43, respectively). Structural figures were prepared with PyMOL (www.pymol.org). (c)-(d) Electrostatic potential at the solvent assessable surface of the StOAD β subunit trimer. The views of panels (c) and (d) are identical to the views of panels (a) and (b), respectively. The γ subunits are presented in cartoon representation and colored in blue. The red and black circles in panels (b) and (d) indicate the first and second negatively charged regions in the β subunit cytoplasmic face, respectively. The black lines in panels (a) and (c) indicate the boundaries of the membrane bilayer.
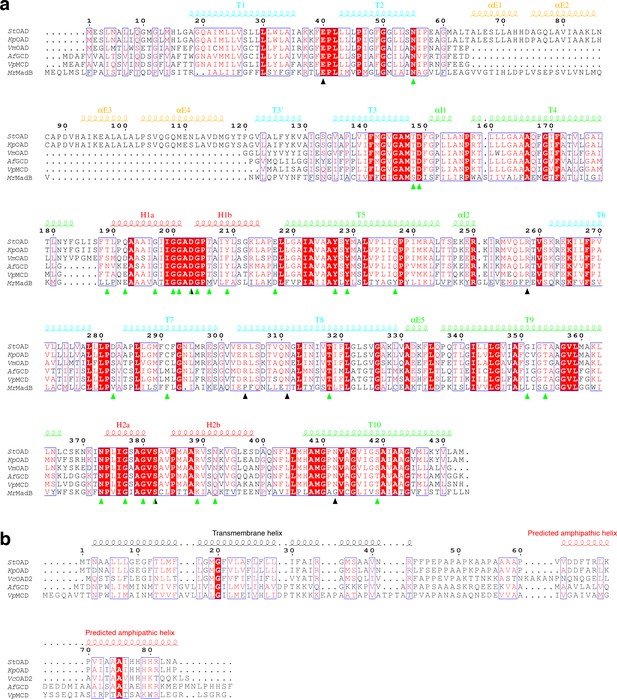
Sequence alignment of the OAD β (a) and γ (b) subunits and their equivalents in other decarboxylase sodium pumps.
Residues numbers and secondary structure elements for the Salmonella typhimurium OAD (StOAD) are shown. Secondary structure elements for the β subunit are colored as in Figure 1. The black and green triangles indicate residues characterized by our study and previous studies on the Klebsiella pneumonia OAD (KpOAD), respectively. The predicted amphipathic helix at the C-terminal region of the γ subunit is indicated in red. VmOAD, Vibrio mediterranei OAD; AfGCD, Acidaminococcus fermentans GCD; VpMCD, Veillonella parvula MCD; MrMadB, Malonomonas rubra MadB; VcOAD2, Vibrio cholerae OAD isoform 2.
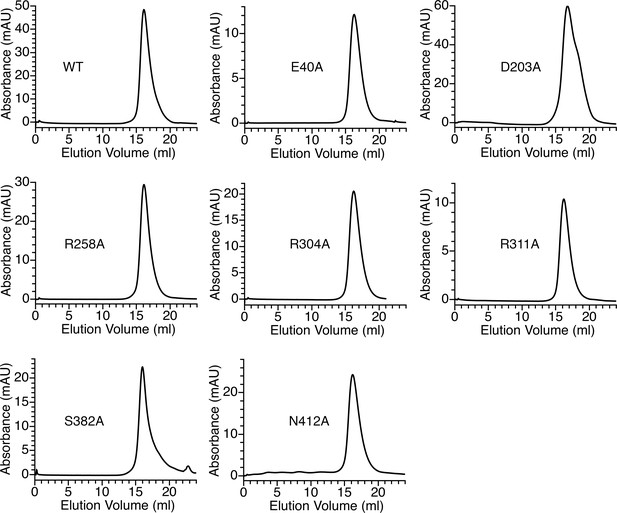
Gel filtration characterization of the wild type and substituted StOAD βγ sub-complex.
The experiments were performed on a Supersose 6 column as described in Materials and methods.
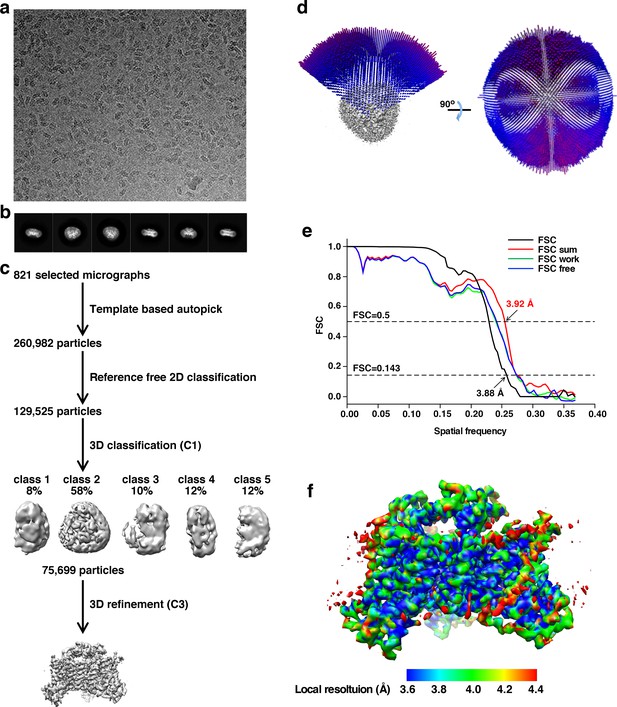
Structure determination of the StOAD βγ sub-complex by cryo-EM.
(a) A representative cryo-EM micrograph. (b) Representative reference-free 2D averages. (c) Workflow of the 3D reconstruction. (d) Angular distribution of particles in the 3D reconstruction. (e) Fourier Shell Correlation (FSC) curves of the 3D reconstruction. FSC, gold-standard FSC curve between the two half maps; FSC sum, FSC between the atomic model and the map; FSC work, FSC between the first half map and the atomic model refined against this map; FSC free, FSC between the second half map and the atomic model refined against the first half map. Resolutions for FSC = 0.143 and FSC sum = 0.50 are indicated. (f) Local resolution of the cryo-EM map.
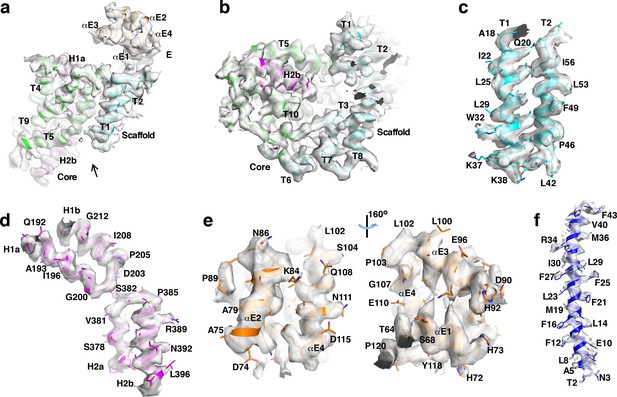
Cryo-EM densities.
(a)-(b) Density for the β subunit. In panel (b), one views along the arrow in panel (a). (c)-(e) Densities for representative regions in the β subunit: helices T1 and T2 (c), the helical hairpins (d), the E domain (e). (f) Density for the γ subunit. Densities for the β and γ subunits are contoured at 5σ and 4σ, respectively. The refined structure is shown for reference.
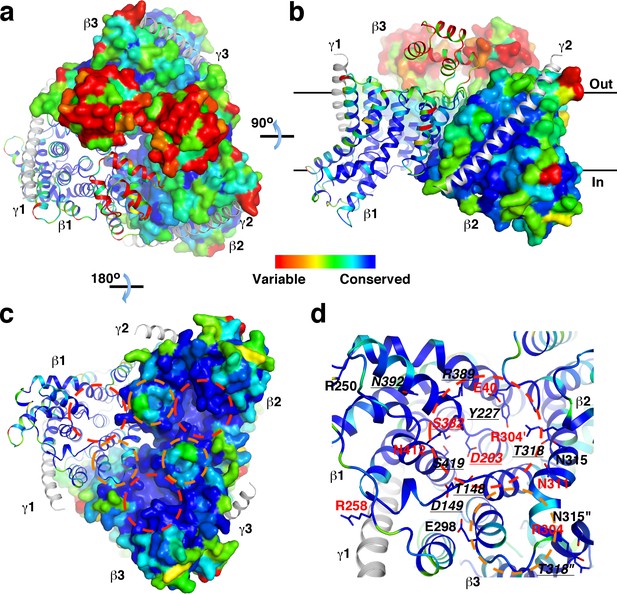
Conservation of individual residues in the β subunit.
Residues in the StOAD β subunit are colored according to their conservation calculated from 150 sequences of OAD, MCD or GCD identified by the Dali server. The γ subunits are presented in cartoon representation and colored in gray. (a)-(c) Conservation of residues in the β subunit. One of the β subunits in the StOAD βγ sub-complex is presented in cartoon representation and the other two are in surface representation. (d) Conserved residues with polar or charged side chains in the β subunit cytoplasmic face. Residues labeled in red and with underlined labels were characterized by our study and previous studies on KpOAD, respectively. The ′ and ″ signs indicate residues in the second and third β subunits in the βγ sub-complex, respectively. The red and orange cycles in (c) and (d) indicate the first and second negatively charged regions in the β subunit cytoplasmic face, respectively.
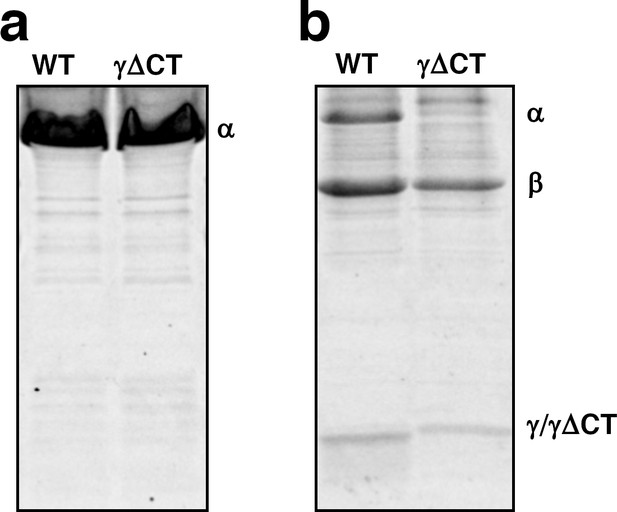
The γ subunit C-terminal tail plays a critical role in the interaction between the α subunit and the βγ sub-complex.
(a) The γΔCT truncation does not significantly change the protein level of the α subunit in the soluble fraction. SDS-PAGE analysis of the α subunit purified by monomeric avidin agarose from the soluble fraction is shown. (b) The γΔCT truncation severely inhibits the interaction between α subunit and the βγ sub-complex. SDS-PAGE analysis of proteins in the membrane fraction purified by Ni-NTA agarose is shown. The resin binds to the 6x Histidine tag engineered to the N-terminus of the γ subunit. The StOAD subunits are indicated. The identity of γΔCT has been confirmed by mass spectrometry.
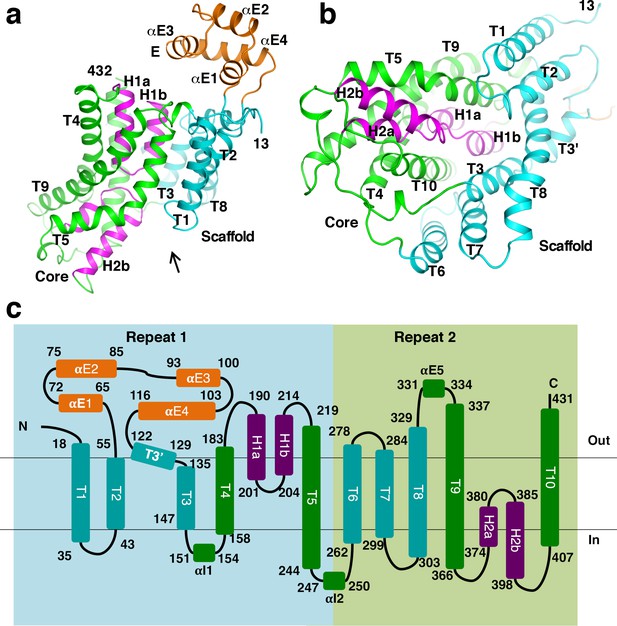
Structure of the β subunit.
(a)-(b) Structure of the StOAD β subunit. In panel (b), one views along the arrow indicated in panel (a). (c) Topology of the β subunit. Boxes in light blue and light green indicate the inverted repeats in the β subunit.
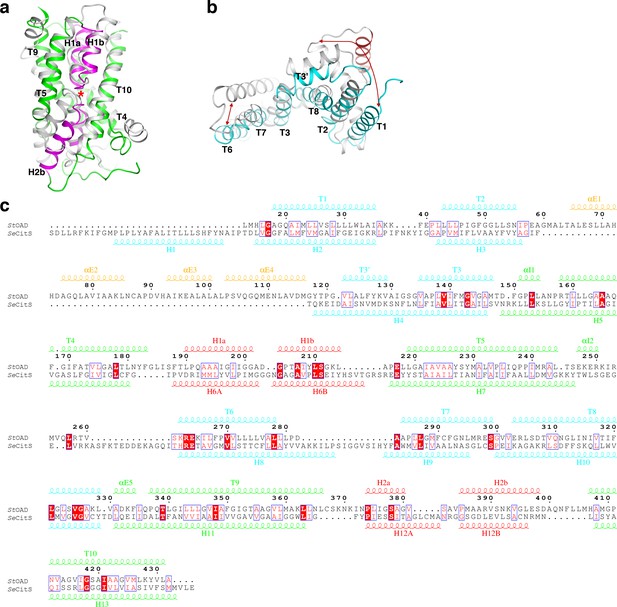
Structural homology between the StOAD β subunit and CitS.
(a)-(b) Structural superimposition of the core (a) and scaffold (b) domains in the StOAD β subunit and equivalent regions in SeCitS (PDB 5A1S, colored in gray). The red star in panel (a) indicates the space between the helical hairpin tips. The extra helix at the SeCitS N terminus is colored in red in panel (b). The red arrows in panel (b) indicate the large conformational differences between T1, T6 and their equivalents in SeCitS. (c) Structure-based sequence alignment of the StOAD β subunit and SeCitS. Secondary structure elements for the StOAD β subunit and SeCitS are shown above and below the sequences, respectively. Residue numbers for the StOAD β subunit are indicated.
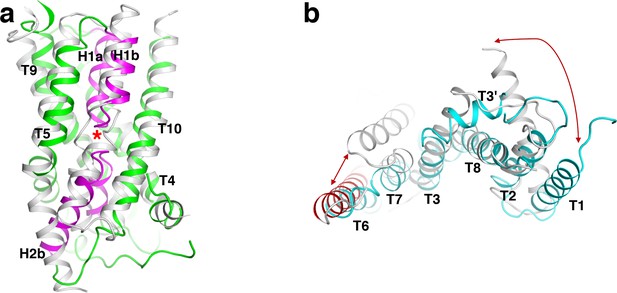
Structural homology between the StOAD β subunit and NapA.
(a)-(b) Structural superimposition of the core (a) and scaffold (b) domains in the StOAD β subunit and equivalent regions in the Thermus thermophilus NapA (TtNapA, PDB 5BZ2, colored in gray). The red star in panel (a) indicates the space between the helical hairpin tips. The extra helix in the TtNapA scaffold domain is colored in red in panel (b). The red arrows in panel (b) indicate the large conformational differences between T1, T6 and their equivalents in TtNapA.
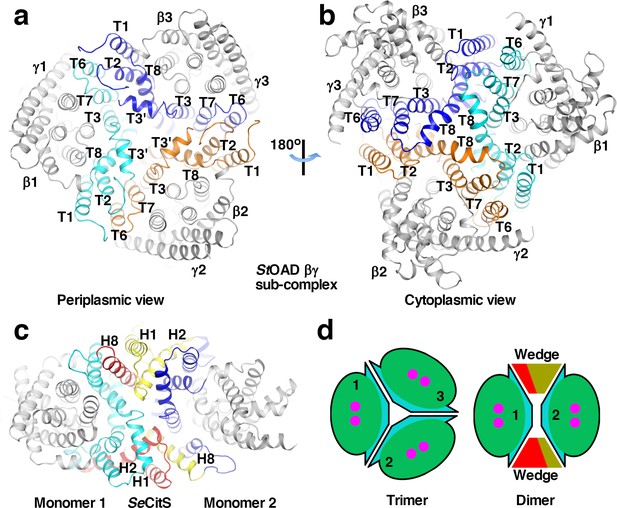
Interactions between StOAD β subunits.
(a)-(b) Structure of the StOAD βγ sub-complex. The scaffold domains in the three β subunits are highlighted in cyan, orange and blue. Their secondary structure elements are labeled. The β subunit core domains and the γ subunits are colored in gray. (c) Structure of the SeCitS dimer (PDB 5A1S). Monomer 1 in the inward-open conformation is aligned to β1 in the StOAD βγ sub-complex in panel (a). Monomer 2 is in the outward-open conformation. Structural elements constituting the ‘wedge’ in monomers 1 and 2 are labelled and highlighted in red and yellow, respectively. Additional elements in the scaffold domain are colored in cyan and blue, respectively. (d) Schematic drawing of the StOAD β subunit trimer and the SeCitS dimer. The monomers are colored as in Figure 1a.
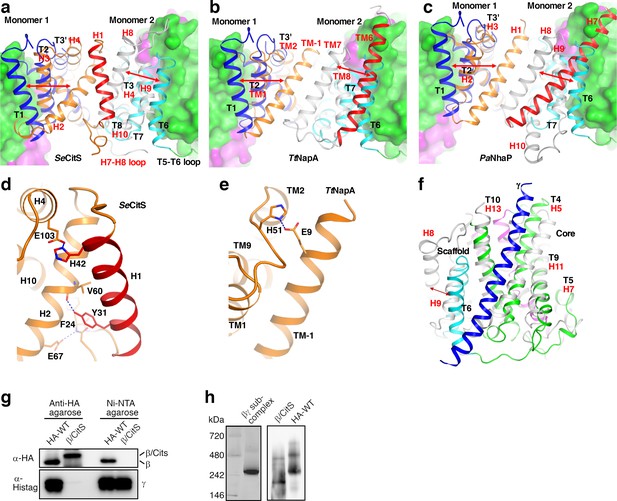
Conformational differences between T1 and T6 and their equivalents in the β subunit homologues.
(a)-(c) Conformations of the T1 and T6 equivalents at the SeCitS (a) TtNapA (b) and the Pyrococcus abyssi NhaP (PDB 4CZ9, PaNhaP, c) dimer interfaces. The scaffold domains of monomers 1 and 2 in SeCitS, TtNapA and PaNhaP are colored in orange and gray, respectively. The extra helices in their scaffold domains are colored in red. Two StOAD β subunits are superimposed on the SeCitS, TtNapA or PaNhaP monomers. Their core domains are presented in surface representation. Their scaffold domains are presented in carton representation in blue and cyan, respectively. The red arrows indicate the large conformational differences between T1, T6 and their equivalents in SeCitS, TtNapA or PaNhaP. Red labels are for SeCitS, TtNapA or PaNhaP. In SeCitS, the T5-T6 loop equivalent (H7-H8 loop) is about 10 residues longer and allows the T6 equivalent (H8) to adopt a conformation away from the core domain. The H8 conformation is also stabilized by mostly hydrophobic interactions with the neighboring H9, and with helices H4 and H10. In TtNapA and PaNhaP, the extra helix in the scaffold domain occupies the position of T6 and ‘pushes’ the T6 equivalent away from the core domain. The conformation of the T6 equivalent is also stabilized by interactions with the T7 equivalent. (d) Hydrogen bonding and salt bridge interactions stabilizing the T1 equivalent in SeCitS (H2). (e) A salt bridge to the N-terminus of the T1 equivalent in TtNapA (TM-1) stabilizes it. (f) T6 mediates interactions between the StOAD β and γ subunits. The structure of the SeCitS monomer (gray) is superimposed. The red arrow indicates the conformational difference between T6 and the equivalent H8 in SeCitS. (g) Co-purification experiments probing the interaction between the StOAD β and γ subunits. In the β/CitS protein, T1, T6, T7 and the T5-T6 loop were substituted with equivalent regions in SeCitS, SeCitS residues N-terminal to the T1 equivalent were also included. The StOAD complex with hemagglutinin (HA)-tagged wild type β subunit or the β/CitS variant and 6x Histidine-tagged γ subunit was purified by anti-HA agarose or Ni-NTA agarose and analyzed for the β and γ subunits with anti-HA and anti-6x Histidine tag antibodies, respectively. (h) Blue native PAGE analysis probing the molecular weight of StOAD and its variants. The left and right panels were from the same gel. In the right panel, samples on the corresponding gel portion were transferred to a polyvinylidene fluoride membrane and probed with anti-HA anti-body to detect the β subunit. In the left panel, the apparent molecular weight of the major species in the Ni-NTA-purified StOAD βγ sub-complex sample (~300 kDa) is somewhat larger than the expected molecular weight of the β3γ3 hetero-hexamer (168 kDa), but most likely represent the hexamer since our EM studies indicated that it is the major species in this sample. The minor species with an apparent molecular weight of ~500 kDa might represent a dimer of the β3γ3 hetero-hexamer. In the right panel, the anti-HA agarose-purified wild type StOAD also contains a major species with an apparent molecular weight of ~300 kDa, suggesting that the β3γ3 hetero-hexamer is the major species in this sample. Since the β/CitS protein does not interact with the γ subunit that mediate interactions with the α subunit (panel g), the anti-HA agarose-purified StOAD with the β/CitS substitution should contain only the β/CitS protein. Two species were observed for this sample (right panel). The apparent molecular weight of the lower molecular weight species is significantly smaller than that of the β3γ3 hetero-hexamer. The size of the β/CitS monomer (53 kDa) is close to the size of the β and γ monomers combined (57 kDa including the HA tag), suggesting that the low molecular weight species is unlikely a β/CitS trimer but a dimer. The high molecular weight species may represent a dimer of the β/CitS dimer.
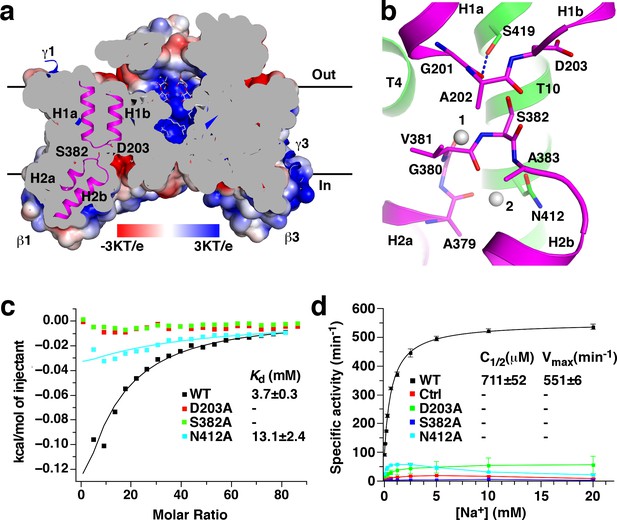
Putative sodium binding sites in the StOAD β subunit.
(a) Cutaway view of the solvent-accessible surface of the StOAD β3 sub-complex. The surface is colored according to the electrostatic potential. Helical hairpins H1, H2 and the Asp203 and Ser382 side chains at their tips are highlighted. The γ subunits are presented in cartoon representation and colored in blue. The DDM molecules in the cavity at the center of the complex are presented in stick representation with their carbon atoms in gray. (b) Structure of the putative sodium binding sites in the β subunit. The gray spheres 1 and 2 indicate equivalent positions of the first and second sodium binding sites in CitS, respectively. Polar or charged groups in the proximity are highlighted. (c) ITC experiments probing sodium binding to the wild type StOAD βγ sub-complex and variants with substitutions at the putative β subunit sodium binding sites. Errors are derived from fitting the data to the one set of sites binding model. (d) Oxaloacetate decarboxylation by the wild type StOAD and variants with substitutions at the putative β subunit sodium binding sites. The activity was reconstituted by supplementing the α subunit with detergent-solubilized βγ sub-complex. The specific activity is defined as the reaction velocity divided by the amount of StOAD. In the control experiment (Ctrl), the βγ sub-complex was omitted in the reaction mixture. The error bars represent standard deviations of three independent experiments. C1/2, sodium concentration at half-maximum activity; Vmax, maximum specific activity. Values of C1/2 and Vmax and their errors were derived from data fitting.
-
Figure 4—source data 1
Summary of ITC experiments.
- https://cdn.elifesciences.org/articles/53853/elife-53853-fig4-data1-v2.xlsx
-
Figure 4—source data 2
Summary of OAD activity experiments.
- https://cdn.elifesciences.org/articles/53853/elife-53853-fig4-data2-v2.xlsx
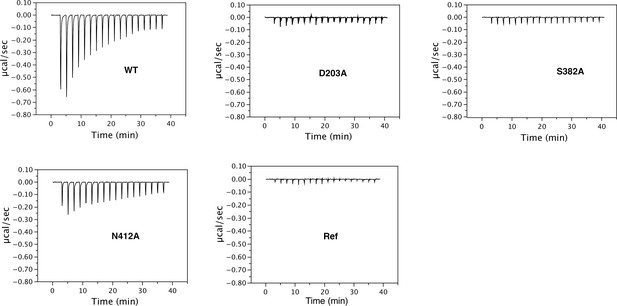
Thermograms of ITC experiments probing sodium binding to the wild type and substituted StOAD βγ sub-complex.
The Reference experiment (Ref) was performed in the absence of the StOAD βγ sub-complex.
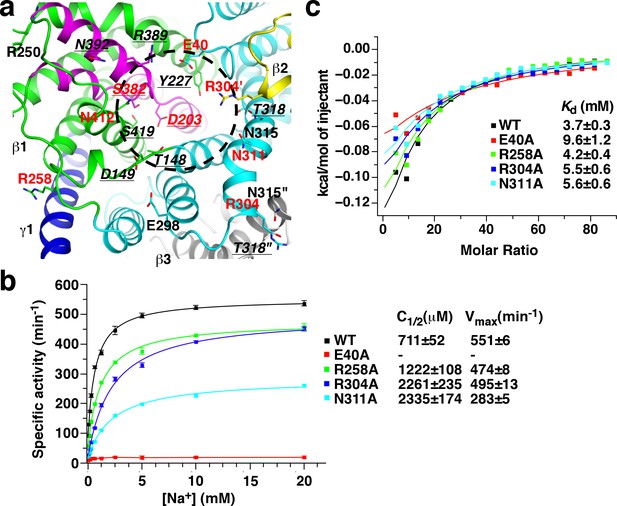
Mutagenesis screen for residues essential for the function of the β subunit.
(a) Structure of the β subunit cytoplasmic face. Highly conserved residues with polar or charged side chains are highlighted. Residues with red and underlined labels were characterized by our study and previous studies on KpOAD, respectively. The ′ and ″ signs indicate residues in the second (yellow) and third (gray) β subunits in the complex, respectively. The black cycle indicates the putative sodium binding cavity. (b) Oxaloacetate decarboxylation by StOAD with substitutions in the β subunit cytoplasmic face. Data for the wild type complex is shown for comparison. (c) ITC experiments probing sodium binding to StOAD βγ sub-complex with substitutions in the β subunit cytoplasmic face. Data for the wild type complex is shown for comparison.
-
Figure 5—source data 1
Summary of OAD activity experiments.
- https://cdn.elifesciences.org/articles/53853/elife-53853-fig5-data1-v2.xlsx
-
Figure 5—source data 2
Summary of ITC experiments.
- https://cdn.elifesciences.org/articles/53853/elife-53853-fig5-data2-v2.xlsx
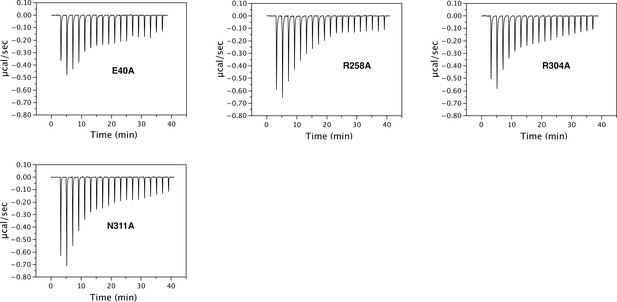
Thermograms of ITC experiments probing sodium binding to the StOAD βγ sub-complex.
Substitutions were introduced on residues selected for the mutagenesis screen.
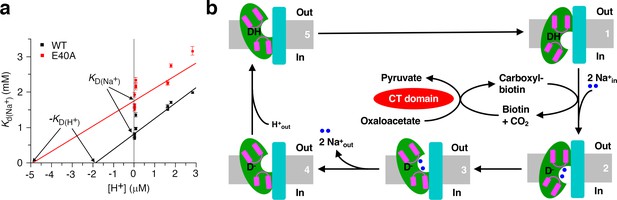
Insights into the OAD reaction cycle.
(a) Sodium binding to the StOAD βγ sub-complex is affected by pH. The dissociation constants (Kd(Na+)) derived from ITC experiments at different pH is plotted against the proton concentration. Error bars represent errors derived from fitting the ITC data to the one set of sites model. The solid lines indicate data fitting with the competitive binding model. The y- and x-axis intercepts of the lines indicate the values for the absolute dissociation constants for sodium and proton (KD(Na+) and KD(H+)), respectively. (b) A model of the OAD-catalyzed sodium transport. Components in the β subunit are colored as in Figure 1. For simplicity, only one β subunit is shown and its domain E is omitted. The gray bars indicate the membrane bilayer. The letter ‘D’ indicates the direct proton donor for carboxyl-biotin decarboxylation.
-
Figure 6—source data 1
Summary of ITC experiments at different pH.
- https://cdn.elifesciences.org/articles/53853/elife-53853-fig6-data1-v2.xlsx
-
Figure 6—source data 2
Dissociation constants of sodium binding to the StOAD βγ sub-complex at different pH measured by ITC.
- https://cdn.elifesciences.org/articles/53853/elife-53853-fig6-data2-v2.xlsx
-
Figure 6—source data 3
Enthalpy of sodium binding to the StOAD βγ sub-complex at different pH measured by ITC.
- https://cdn.elifesciences.org/articles/53853/elife-53853-fig6-data3-v2.xlsx
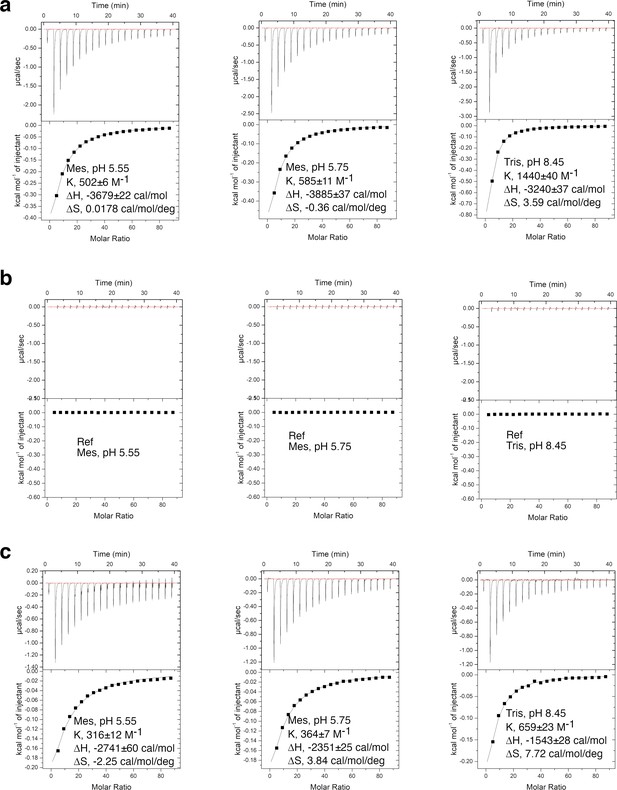
ITC experiments probing sodium binding to the wild type (a) and the E40A substituted (c) StOAD βγ sub-complex at different pH.
(b) Reference experiments carried out in the absence of the StOAD βγ sub-complex.
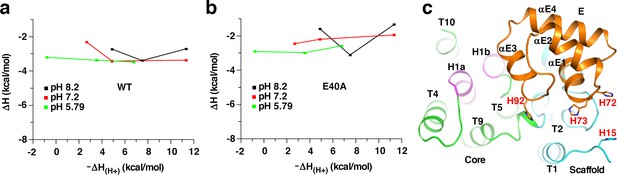
ITC-measured enthalpy of sodium binding to the StOAD βγ sub-complex does not correlate with the buffer protonation enthalpy.
(a)-(b) Plots of the ITC-measured enthalpy of sodium binding to the wild type (a) or E40A substituted (b) StOAD βγ sub-complex (ΔH) versus the buffer protonation enthalpy (ΔH(H+)). (c) A cluster of histidine residues at the periplasmic face of the StOAD β subunit. The histidine residues are highlighted with red labels.
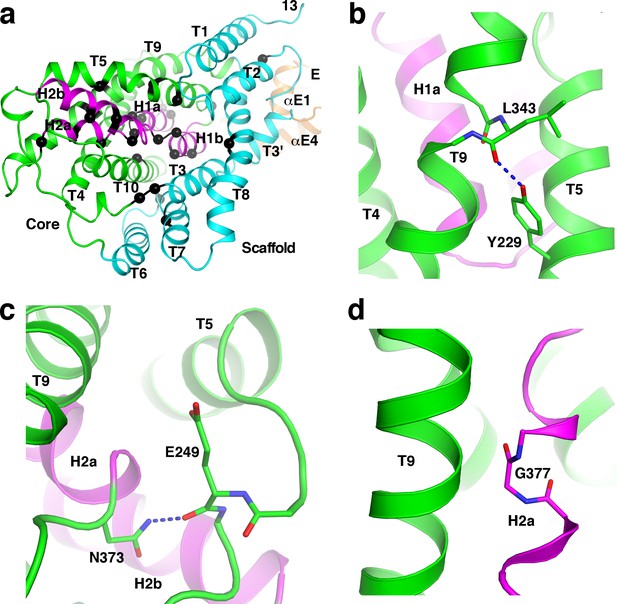
OAD β subunit residues characterized by previous mutagenesis studies.
(a) OAD β subunit residues characterized by previous mutagenesis studies on KpOAD. They are mapped onto the structure of the StOAD β subunit and highlighted with black spheres. (b)-(d) Structural basis for the functional importance of Tyr229 (b), Asn373 (c) and Gly377 (d). They contribute to the stability of the core domain by interacting with neighboring residues.
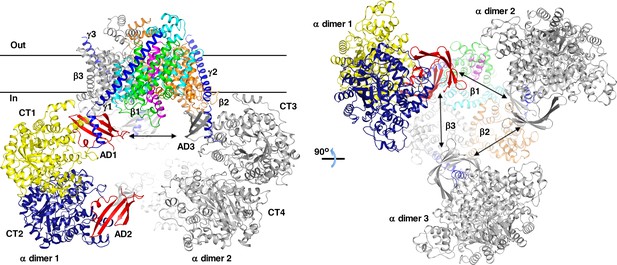
model of the OAD holoenzyme.
The α subunit dimer is modeled based on the structure of pyruvate carboxylase (PDB 3BG5), whose CT and PT domains are homologous to the CT and AD domains in the α subunit. One of the AD domains in the α subunit dimer interacts with the γ subunit cytosolic tail. The BCCP domain is omitted due to its structural flexibility and small size. CT domains 1 and 2 in the α subunit dimer one are colored in yellow and blue, respectively; the AD domains are in red. α subunit dimers 2 and 3 are colored in gray. The arrows indicate potential interactions between the α subunit dimers. The black lines indicate boundaries of the membrane bilayer.
Tables
Cryo-EM data collection and structure refinement statistics
Cryo-EM structure of the StOAD βγ sub-complex (PDB 6IWW) | |
---|---|
Data collection and procession | |
Magnification (nominal) | 105,000 |
Voltage (kV) | 300 |
Electron exposure (e-/Å2) | 50 (32 frames) |
Defocus range (μm) | 1.0–3.5 |
Pixel size (Å) | 0.68 |
Symmetry imposed | C3 |
Initial particle images (no.) | 260,982 |
Final particle images (no.) | 75,699 |
Map resolution (Å) | 3.88 |
FSC threshold | 0.143 |
Refinement | |
Initial model used | EMBUILDER generated model |
Model resolution (Å) | 3.88 |
FSC threshold | 0.143 |
Map sharpening B factor (Å2) | −250 |
Model composition | |
Non-hydrogen atoms | 10,227 |
Protein residues | 1386 |
Ligands | 3 |
B-factors (Å2) | |
Protein | 41.7 |
Ligand | 23.9 |
R.m.s deviations | |
Bond lengths (Å) | 0.008 |
Bond angles (°) | 1.016 |
Validation | |
Molprobity score | 1.61 |
Clashscore | 5.83 |
Poor rotamers (%) | 0 |
Ramachandran plot | |
Favored | 95.78 |
Allowed (%) | 4.22 |
Disallowed (%) | 0 |
Reagent type (species) or resource | Designation | Source or reference | Identifiers | Additional information |
---|---|---|---|---|
Gene (Salmonella typhimurium) | StOAD | GenBank | GenBank: CP007235.2, bases 836524–839869 | |
Strain, strain background (Escherichia coli) | BL21 star (DE3) | Thermo Fischer | C602003 | Chemical competent cells |
Antibody | anti-HA (rabbit monoclonal) | Cell Signaling Technology | Cat# 3724S | 1:1000 |
Antibody | HRP-linked anti-rabbit IgG (goat polyclonal) | Cell Signaling Technology | Cat# 7074P2 | 1:2000 |
Antibody | HRP-linked anti-6x Histidine tag (goat polyclonal) | Abcam | Cat# ab1269 | 1:5000 |
Recombinant DNA reagent | StOAD-pET28a (plasmid) | This paper, Materials and methods | StOAD expersion, can be obtained from the Xiang lab | |
Recombinant DNA reagent | StOAD-β/CitS-pET28a (plasmid) | This paper, Materials and methods | StOAD β/CitS variant expersion, can be obtained from the Xiang lab | |
Recombinant DNA reagent | StOADα- pET28a (plasmid) | This paper, Materials and methods | StOAD α subunit expersion, can be obtained from the Xiang lab | |
Commercial assay or kit | NativePAGE Novex Bis-Tris gel | Thermo Fisher | Cat# BN1001BOX | |
Software, algorithm | DYNAMICS V6 | Wyatt Technologies | ||
Software, algorithm | MotionCor2 | Zheng et al., 2017 | ||
Software, algorithm | CTFFIND4 | Rohou and Grigorieff, 2015 | ||
Software, algorithm | e2boxer.py | Tang et al., 2007 | ||
Software, algorithm | RELION | Scheres, 2012 | ||
Software, algorithm | EMAN | Ludtke et al., 1999 | ||
Software, algorithm | EMBUILDER | Zhou et al., 2017 | ||
Software, algorithm | COOT | Emsley and Cowtan, 2004 | ||
Software, algorithm | PHENIX | Adams et al., 2010 | ||
Software, algorithm | ResMap | Kucukelbir et al., 2014 | ||
Software, algorithm | Dali server | Holm and Rosenström, 2010 | ||
Software, algorithm | HKL2000 | Otwinowski and Minor, 1997 | ||
Software, algorithm | MOLREP | Vagin and Teplyakov, 1997 | ||
Software, algorithm | ORIGIN 7.0 | Originlab | ||
Software, algorithm | QTIPLOT | www.qtiplot.com |
Additional files
-
Supplementary file 1
Supplementary data.
(A) Crystal diffraction data collection and structure refinement statistics.(B) Previous mutagenesis studies performed on KpOAD. (C) Summary of ITC experiments at different pH.
- https://cdn.elifesciences.org/articles/53853/elife-53853-supp1-v2.docx
-
Transparent reporting form
- https://cdn.elifesciences.org/articles/53853/elife-53853-transrepform-v2.docx